Abstract
Most of the classical therapies for solid tumors have limitations in achieving long-lasting anti-tumor responses. Therefore, treatment of cancer requires additional and multimodal therapeutic strategies. One option is based on the vaccination of cancer patients with autologous inactivated intact tumor cells. The master requirements of cell-based therapeutic tumor vaccines are the: (a) complete inactivation of the tumor cells; (b) preservation of their immunogenicity; and (c) need to remain in accordance with statutory provisions. Physical treatments like freeze-thawing and chemotherapeutics are currently used to inactivate tumor cells for vaccination purposes, but these techniques have methodological, therapeutic, or legal restrictions. For this reason, we have proposed the use of a high hydrostatic pressure (HHP) treatment (p ≥ 100 MPa) as an alternative method for the inactivation of tumor cells. HHP is a technique that has been known for more than 100 years to successfully inactivate micro-organisms and to alter biomolecules. In the studies here, we show that the treatment of MCF7, B16-F10, and CT26 tumor cells with HHP ≥ 300 MPa results in mainly necrotic tumor cell death forms displaying degraded DNA. Only CT26 cells yielded a notable amount of apoptotic cells after the application of HHP. All tumor cells treated with ≥ 200 MPa lost their ability to form colonies in vitro. Furthermore, the pressure-inactivated cells retained their immunogenicity, as tested in a xenogeneic as well as syngeneic mouse models. We conclude that the complete tumor cell inactivation, the degradation of the cell’s nuclei, and the retention of the immunogeneic potential of these dead tumor cells induced by HHP favor the use of this technique as a powerful and low-cost technique for the inactivation of tumor cells to be used as a vaccine.
Introduction
The standard therapy for the treatment of human cancers is mostly via the use of surgery, radiation therapy, and/or chemotherapy. Single and combined treatments often lead to local tumor control and/or regression, and can prolong the lifespan of the patients (Paukovits et al., Citation2004; Ott et al., Citation2009). However, these treatments are often restricted in their capacities to provide a long-lasting anti-tumor effect and also to be effective against hidden metastases and recurrent cancers. Apart from those drawbacks, there are often numerous toxic side effects that impact on normal tissues.
It has become increasingly clear that the immune system is involved in the control of tumor progression and maintenance of a host’s subsequent tumor-free status (Apetoh et al., Citation2008). The immunotherapy of cancer is a growing and promising approach and various trials have been performed with a range of candidate treatments during the past decade. Various cancer immunotherapy studies (i.e., Bocchia et al., Citation2000; Amato, Citation2003; Umansky et al., Citation2009) arose not only from a significant expansion of the understanding of immune responses and the interactions between host and tumor, but also of how one can manipulate the immune system to exert a tumor-specific immune response. Several of these types of studies have focused on the immunization of the patients with peptides or whole antigens (Yi, Citation2003), intact tumor cells (de Gruijl et al., Citation2008), or dendritic cells (DC) pulsed with antigens from cancers (Esche et al., Citation1999).
The immunotherapy of cancer (i.e., the use of tumor vaccines) has become an important modality for completing and enhancing the current standard therapies that are being devised and/or utilized for the long-term control of a malignancy (Chen, Chang, and Goldenberg, Citation2009). However, the clinical successes of such vaccines have been underachieving (Rosenberg, Yang, and Restifo, Citation2004). Insufficient immune responses induced by these kinds of vaccines—leading to inadequate amounts of reactive lymphocytes—are thought to be one reason for a lack of efficacy (Rosenberg et al., Citation2004). In addition, the induction of tolerance and the activation of immune suppressors (such as regulatory T-lymphocytes and myeloid-derived suppressor cells) may contribute to the poor clinical outcomes. Hence, the development of new vaccines that are able to activate the immune system to produce a specific and long-lasting anti-tumor immunity is still necessary. The therapeutic application of whole-cell tumor vaccines offers the advantage of presenting various antigens, thus leading to a better outcome; this approach has already been demonstrated in clinical trials with renal cell carcinoma (Jocham et al., Citation2004) or multiple myeloma (Rosenblatt and Avigan, Citation2008). It has also become increasingly clear that vaccines against tumors should induce the presentation of tumor-associated antigens to the immune system in a manner that will elicit strong immune responses and induce immunological memory. Both of these factors would be critical for assuring a substantive effective immune response against small primary tumors, residual cancers, and (micro-) metastases (Moingeon et al., Citation2002; Yannelli et al., Citation2003).
One option for vaccination is a formulation that presents the host with autologous tumor cells—thereby making a vast number of defined tumor antigens accessible to the immune system simultaneously (Copier et al., Citation2007; Dillman et al., Citation2007). Regardless of the ultimate goal, each of these vaccines has to also fulfill the master requirements of all cell-based therapeutic tumor vaccines, including (a) the complete inactivation of the tumor cells, (b) the preservation of the tumor cells’ immunogenicity, and, finally, (c) being in accordance with statutory provisions. Currently, physical treatments (e.g., freeze-thawing, X-ray) and chemotherapeutics are used to inactivate tumor cells for vaccination experiments; however, these techniques often have restrictions. Either the cells cannot be safely inactivated by a mild treatment or the immunogenicity of the dying cells is very weak. One example of the legal restrictions is listed in the German drug laws, i.e., there is a prohibition against the X-ray irradiation of agents that are to be injected into humans (Drug Law, 2009). Another is that tumor cells killed by toxic agents have to be free of the lethal drug before re-injection.
One could argue that necrotic tumor cells are safely inactivated, induce inflammation, and display high immunogenicity. In contrast, apoptotic cells are often poorly immunogeneic (Voll et al., Citation1997). Mouse models revealed that apoptotic cells display a significant lower immunogenicity in comparison to viable ones that induce immune responses when injected in a xenogeneic species (Stach et al., Citation2000; Gaipl et al., Citation2003a). In the syngeneic situation, under healthy conditions, viable and apoptotic cells do not induce immune reactions or even lead to anti-inflammation (Voll et al., Citation1997). In contrast, necrotic cells lead to immune stimulation. However, ex vivo inactivation processes that induce necrosis do not generally exhibit good immunogeneic responses in vivo. The immunogenicity of the inactivated cells is strongly dependent on the death-initiating stimulus and has to be analyzed individually (Krysko et al., Citation2006; Kepp et al., Citation2009). Ultimately, the inactivation processes have to be performed in a highly reproducible manner—in accordance with good manufacturing practices—and meet all legal requirements.
High hydrostatic pressure (HHP) treatment fulfills these specifications (Masson et al., Citation2001; San Martin et al., Citation2002; Aertsen et al., Citation2009; Shearer et al., Citation2009). The use of HHP has been established for the sterilization of foodstuffs and pharmaceuticals; in these cases, bacteria and fungi are inactivated without thermal impact or the use of chemical compounds or radiation (Balny et al., Citation1992; Masson et al., Citation2001; San Martin et al., Citation2002; Aertsen et al., Citation2009). In medicine, one potential application of HHP is the disinfection of bone transplants while maintaining the biomechanical stability of the bone (Diehl et al., Citation2008; Frey et al., Citation2008). HHP has also been explored for use as a new method of viral and bacterial vaccine development (Shearer and Kniel, Citation2009). Furthermore, we and other investigators have shown that HHP is able to alter biomolecules and to induce distinct forms of cell death in human tumor cell lines (Diehl et al., Citation2003; Frey et al., Citation2004, Citation2008; Korn et al., Citation2004). In the present study, we focused on the use of this technique to fulfill at least two of the above-noted master requirements for devising a cell-based therapeutic tumor vaccine, i.e., if HHP can effectively inactivate tumor cells while preserving their immunogenicity.
In our settings, HHP is defined as pressure head equal to or greater than 100 MPa [1 MPa = 10 bar = 9.86923 atm = 145.0377 psi (Frey et al., Citation2008)]. The influences of this non-physiological pressure on biomolecules have already been investigated, reported in detail, and reviewed elsewhere (e.g., Balny et al., Citation1992; Balny, Citation1999; Masson et al., Citation2001; Balny, Masson et al., Citation2002; Boonyaratanakornkit et al., Citation2002; Macgregor, Citation2002; San Martin et al., Citation2002; Smeller, Citation2002; Winter et al., Citation2004; Meyer-Pittroff et al., Citation2006; Aertsen et al., Citation2009). In general, it is well established that protein tertiary and quaternary structures can be altered by HHP; this implies that proteins can be denatured while maintaining their primary structure. With certain types of proteins, the significance of i.e., debatable, that is, several studies have shown that enzymes may partly or completely forfeit their efficiency after HHP treatment (Balny, Citation1999; Boonyaratanakornkit et al., Citation2002; Smeller, Citation2002; Winter and Dzwolak, Citation2004). In a somewhat similar vein, the (primary) structure of DNA has been shown to be highly resistant to HHP [i.e., early studies have described the primary structure of DNA to be stable up to an HHP of 1000 MPa (Heden, Citation1964; Heden and Rupprecht, Citation1966)] even though at an HHP below 1000 MPa, there is a clear transition from double- to single-stranded DNA (Dubins et al., Citation2001; Macgregor, Citation2002). Furthermore, at these lower pressures, the conformation of phospholipid bilayers is altered from a fluid-crystalline to a gel-like type (Winter, Citation2002; Winter and Dzwolak, Citation2004).
To examine the capability of HHP to inactivate tumor cells, differing mammalian tumor cell lines were treated with pressures from 100 to 500 MPa. Thereafter, cell death was monitored over the course of a 48 h culture period. To test the effect of the HHP on the proliferative ability of these tumor cells, a clonogenic assay was performed. Finally, the immunogenicity of these HHP-treated tumor cells was tested in a xenogeneic, as well as in a syngeneic, mouse model.
Materials and methods
Unless elsewise specified, reagents and disposable cell culture equipment were purchased from Sigma (St. Louis, MO); and Greiner BioOne (Greiner BioOne, Kremsmuenster, Austria), respectively.
Cell lines and culture
Mammalian tumor cell lines were used for the HHP treatment. Two mouse tumor cell lines, for example, the melanoma cell line B16-F10 (CRL-6475) and the colon carcinoma cell line CT26.WT (CRL-2638), as well as two human tumor cell lines, e.g., the human adenocarcinoma MCF7 (HTB-22) and the human Burkitt’s lymphoma B-lymphocyte Raji (CCL-86), were used in this study. All cell lines were obtained from ATCC/LGC standards GmbH (Wesel, Germany). The cell lines B16-F10 and MCF7 were cultured with Dulbecco’s modified Eagle’s medium (PAN Biotech GmbH, Aidenbach, Germany) supplemented with 10% fetal bovine serum (FBS; Biochrom, Berlin, Germany), 1% L-glutamine, 1% penicillin–streptomycin, 1 g glucose/L, and 1% sodium pyruvate (all from Invitrogen, Karlsruhe, Germany). The CT26.WT and the Raji cells were cultured with RPMI 1640 (Invitrogen) supplemented with 10% FBS, 1% L-glutamine, and 1% penicillin–streptomycin. All cell lines were maintained under conditions of 37°C and 80% relative humidity in a 5.5% CO2 atmosphere.
High hydrostatic pressure treatment of cells
Before treatment, cells were detached with Trypsin-EDTA (Invitrogen), centrifuged, and then re-suspended in culture medium. For a pressure treatment, cryogenic vials (Greiner BioOne) were used to avoid leakage during pressure treatment. The cryogenic vials (with a volume of 2.0 mL) were filled completely with cell suspension and closed tightly to avoid the appearance of air bubbles possibly leading to a drop in pressure during an HHP treatment. Afterwards, the vials were sealed with Parafilm (American National Can, Chicago, IL). The vials prepared in this way were placed in the pressure chamber (also called pressure-autoclave) of the high-pressure equipment; a schematic representation of the system employed is depicted in . The pressure-autoclave was subsequently locked by a screwing flange and the pressure was generated with the hand pump. The cells were always treated over a time period of 300 sec.
Figure 1. Schematic representation of the high hydrostatic pressure (HHP) equipment. The HHP equipment consists of the following components: (1) flanged closure; (2) pressure autoclave; (3) hand pump (SITEC-Sieber Engineering, Maur, Switzerland); (4) reservoir filled with pressure-transmitting media (PTM) (Tivela S220; Shell, Hamburg, Germany); (5) valves SITEC-Sieber Engineering, Maur, Switzerland); and (6) digital pressure sensor. The wrapped sample has to be positioned in the pressure autoclave (2). The pressure autoclave (2) was filled completely with PTM from the reservoir (4; with avoidance of trapped air) and subsequently locked by screwing the flange (1). Pressure was applied manually by means of the hand pump (3) with an approximate pressure increment of 10 MPa/sec. When the aspired pressure was reached, the valve (5) for the pressure autoclave was locked and pressure was held for 300 sec. The pressure was monitored continually via the digital pressure sensor (6). Afterwards, the valve was opened slowly and pressure was decompressed to atmospheric pressure by inverse moving of hand pump (3).
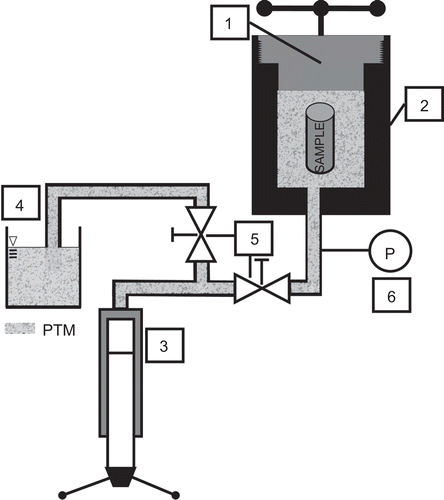
Detection of morphological changes of the cells by flow cytometry
To distinguish between viable and dead cells, the morphology of the cells were measured by analyzing the FSc (Forward Scatter) for the dimension of the cells and the SSc (Side Scatter) to obtain a description of their granularity, as described previously (Elstein et al., Citation1994; Vermes et al., Citation2000).
Annexin V–FITC/propidium iodide staining
For the flow cytometric discrimination between apoptotic and necrotic cells, a staining procedure with FITC-labeled recombinant human annexin A5 (AxV) (Genethor, Berlin, Germany) and PI (Sigma) was used. AxV binds with a high specificity and in a Ca2+-dependent manner to phosphatidylserine (PS). The PS, normally relegated to the inner leaflet within viable cells, is exposed on the outer leaflet of apoptotic cells (Vermes et al., Citation1995, Citation2000). However, due to the loss of membrane integrity, AxV also gets access to PS on the inner leaflet of necrotic cells. Therefore, counter-staining with PI was used to discriminate necrotic from apoptotic cells. Briefly, ≈ 8 × 105 cells were incubated with 0.2 µg AxV-FITC and 8.0 ng PI for 30 min at 4°C in the dark and subsequently analyzed by flow cytometry. Viable cells showed neither binding of AxV nor staining with PI (referred to as AxV−/PI− cells). Apoptotic and necrotic cells were defined as AxV+/PI− and AxV+/PI+, respectively.
Detection of cells with sub-G1 DNA content
To determine the cellular DNA content, cells were stained with PI in the presence of the detergent Triton X-100 (Sigma), according to the method described by Nicoletti et al., (Citation1991). In brief, an aqueous solution composed of 0.1% sodium citrate, 0.1% Triton X-100, and 0.7 µg/mL PI was incubated for at least 12 h in the dark with ≈ 8 × 105 cells. PI-fluorescence was then recorded using an EPICS™ XL-MCL flow cytometer (Beckman Coulter, Fullerton, CA).
Clonogenic assay
Following a given HHP treatment, the cells were plated (in complete medium) into culture dishes. After 14–18 days, colonies in the dishes were stained with methylene blue solution for 30 min and then counted. The calculation of colony-forming units (CFU) was performed by setting the total numbers of colonies associated with the untreated cells (i.e., mock) to 100% and then calculating the number of the colonies present in the dishes of cells that received the HHP treatment divided by the number associated with the untreated (mock) cells.
Immunization mouse models
Throughout these studies, all mice were provided a special diet and water ad libitum, and were kept individually in well-ventilated cages under standard conditions of humidity (55 ± 5%), temperature (22 ± 2°C), and light (12/12 h light-dark cycles). The animal studies were approved by the “Regierung von Mittelfranken,” and conducted according to the guidelines of Federation of European Laboratory Animal Science Associations (FELASA) and the “Gesellschaft fuer Versuchstierkunde” (GV-SOLAS). This assured that the studies adhered to internationally accepted standards for the ethical use of animals in research.
For analyzing the immunogenicity of the HHP-inactivated tumor cells, immunization of wild-type mice was performed. For the xenogeneic model, female C57BL/6 mice (Charles River Laboratories, Sulzfeld, Germany) at an age of 4–6 weeks were immunized intraperitoneally with 2 × 106 Raji (CCL-86) cells suspended in Ringer’s solution (DeltaSelect GmbH, Muenchen, Germany). In addition, for the syngeneic model, Balb/c mice (own breeding, Franz-Penzoldt-Zentrum, Erlangen, Germany) were injected with 5 × 106 CT26 cells suspended in Ringer’s solution. The immunization was executed three times, every 14 days. For the xenogeneic model, 2 week after the last immunization, a delayed-type hypersensitivity test (DTH) was performed by injecting 5 × 106 viable Raji cells (70 µL in Ringer’s solution) subcutaneously into the palmar region of one hind paw of the mice. The other hind paw served as control; an equal volume of Ringer’s solution was injected. The swelling of the paws was then measured with a digital caliper (Kroeplin, Schluechtern, Germany).
For both the xenogeneic and the syngeneic model, blood of the mice was drawn before and after the three-time immunization to obtain serum for further analyses. The tumor cell-specific antibodies (i.e., against Raji and CT26 cells) in the sera of mice were analyzed by indirect immune fluorescence. In brief, viable target cells were incubated with the serum of the immunized mouse for 90 min at 4°C in the dark. Thereafter, the cells were washed and the bound antibodies were analyzed by flow cytometry using a fluorescein-tagged F(ab’)2 fragment of goat anti-mouse IgG (Invitrogen, Molecular Probes, Karlsruhe, Germany).
The absolute IgG antibody content in each serum sample was evaluated using a sandwich enzyme-linked immunosorbent analysis (ELISA). Specifically, the wells of 96-well plates (Costar 3590; Corning, Lowell, MA) were coated overnight with a goat anti-mouse IgG capture antibody (SouthernBiotech, Birmingham, AL) using standard protocols. The test serum sample was then added to a dedicated well and the plate incubated at room temperature for 60 min. After gentle washing with PBS-Tween [phosphate-buffered saline (PBS; Invitrogen) with 0.05% (v/v) Tween-20] to remove non-IgG materials; any bound serum IgG was detected using a horseradish peroxidase-conjugated IgG detection antibody (SouthernBiotech). To visualize the secondary antibody present, o-phenylenediamine dihydrochloride (Sigma, Taufkirchen, Germany) was then added to the well as substrate. Once the color in the well had developed, the optical density was measured at 495 nm using an HTS 7000 Perkin Elmer plate reader (Perkin Elmer, Waltham, MA).
Results
Detection of dying tumor cells (using morphologic criteria) after HHP treatment
Dying cells undergo characteristic morphological changes that can be analyzed by flow cytometry. In this approach, the FSc is used to get information about the cell’s dimensions and the SSc reflects its granularity (Elstein and Zucker, Citation1994). Dying cells display an increase of the granularity and shrinkage in size due to “blebbing” (Hacker, Citation2000; Franz et al., Citation2007). Using flow cytometry, the morphology and viability of HHP-treated cells over a culture period of 48 h after the pressure treatment—defined by their FSc/SSc properties—was assessed (). All cell lines, MCF7, CT26, and B16-F10, were hardly influenced [as compared to mock (untreated) cells] if they were treated with 100 MPa. If the cells were treated with 200 MPa, clear differences in viability could be observed. The MCF7 cells were swiftly affected, i.e., by 2 h after treatment, the amount of viable cells decreased to ≈ 30%. In contrast, the B16-F10 and CT26 cells showed a delayed—but stronger—decrease in percentages of viable cells. B16-F10 cells treated with 200 MPa were still viable 2 h after treatment. However, during the subsequent 6 h of culture, almost all cells were dying. CT26 cells behaved in a similar manner. With all three cell lines, only a very few viable cells can be detected at any timepoint during the 48 h culture after an HHP treatment of ≥ 300 MPa.
Figure 2. High hydrostatic pressure (HHP) treatment effects on the viability of tumor cells. Viability of the cells was determined by their FSc/SSc properties. The line plots display the percentage of viable MCF7, B16-F10, or CT26 cells after pressure application up to 500 MPa in dependence on culture time. Data (expressed as mean ± SD) were obtained from at least three independent experiments, each performed in duplicate.
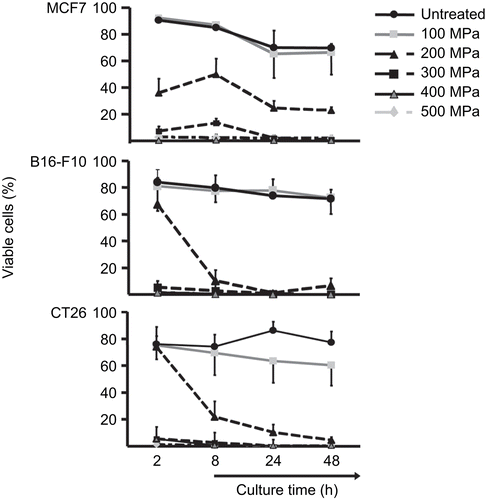
Detection of apoptosis and necrosis among HHP-treated tumor cells
To evaluate the type of cell death induced by HHP treatment as a function of the amount of applied pressure, an AxV-FITC/PI staining was performed. As expected, the fraction of viable cells (AxV−/PI−) was predominant (compared with apoptotic and necrotic ones at any time of the analyses) in the mock-treated cells. An HHP of 100 MPa did not significantly affect the viability of the cells (). Treatment with 200 MPa led to a continuous decrease in the numbers of viable cells and increased the relative content of necrotic cells. After 24 h of culture, the necrotic cell fraction increased to 85% and 95% for the MCF7 and B16-F10 cells, respectively. In comparison, CT26 cells treated with 200 MPa displayed a higher level of apoptotic (AxV+/PI−) cells.
Figure 3. High hydrostatic pressure (HHP) treatment effects upon the levels of necrosis in tumor cells. The bar charts represent the percentage of viable (AxV−/PI−), apoptotic (AxV+/PI−), and necrotic (AxV+/PI+) (A) MCF7, (B) B16-F10, and (C) CT26 tumor cells that were treated with the indicated pressures, as a function of the length of post-treatment culture time. Data (expressed as mean ± SD) were obtained from at least three independent experiments, each performed in duplicate.
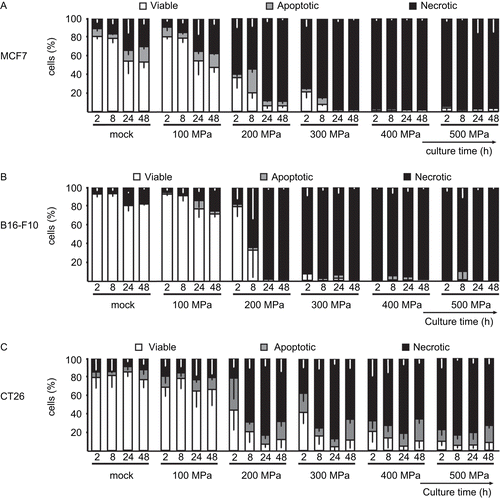
If cells were treated with pressure of 300 MPa, an even more expeditious reduction in the levels of viable cells was detected. After 8 h of culture, viable MCF7 cell levels decreased to 10% and viable B16-F10 levels dropped to 5%. As at the lower pressures, the CT26 cells again displayed the highest pressure resistance at 300 MPa. Treatment of the MCF7 and B16-F10 cells with a pressure of 400 or 500 MPa led to a complete inactivation (characterized by the presence of > 90% of necrotic cells) directly after the treatment. In contrast, treatment of the CT26 cells with these pressure stages led to a lower induction of necrosis; up to 25% of the cells were apoptotic and up to 10% of the cells still showed a viable phenotype (based on the level of changes in PS exposure).
Analysis of cellular DNA content in HHP-treated tumor cells
For the determination of DNA content, HHP-treated cells were stained with PI in the presence of the detergent Triton X-100. Each phase of a cell cycle is characterized by its relative DNA content. Apart from allowing for assessment of relative cell cycle status among the cells, this staining method also enables a determination of the levels of degraded DNA (sub-G1 DNA; a hallmark of apoptotic or secondary necrotic cell death) present to be made. As displayed in , the treatment of the three different tumor cell lines with 200 MPa yielded similar results (). The amount of degraded sub-G1 DNA increased during the observation period. After 48 h, the sub-G1 content reached more than 80% in each of the cell lines examined. Treatment with a pressure ≥ 300 MPa resulted in no significant increase in the sub-G1 DNA content of the cells during the 8 h of culturing. Only at later timepoints did the sub-G1 DNA content become highly increased.
Figure 4. High hydrostatic pressure (HHP) treatment effects upon necrotic tumor cell and degraded DNA content. The DNA content of (A) MCF7, (B) B16-F10, and (C) CT26 cells is illustrated by the bars. The cells were treated with the indicated pressure and then cultured for up to 48 h. The percentages of fractions of cells with sub-G1, G1, and S/G2 content are displayed. Data (expressed as mean ± SD) were obtained from at least three independent experiments, each performed in duplicate.
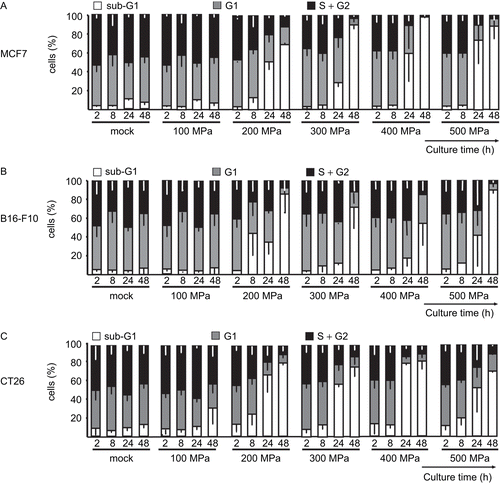
Analysis of clonogenic behavior of HHP-treated tumor cells
The clonogenic assay was performed to investigate the ability of HHP-treated cells to form colonies in vitro. It was previously shown that these results often correlate with the tumor cell “outgrow” in vivo. After treatment with 100 MPa, the colony-forming ability of the B16-F10 and CT26 cells was reduced by ≈ 30% (). MCF7 cells, in contrast, displayed a slight increase in ability. However, all cells treated with ≥ 200 MPa lost their ability to form colonies in vitro. Even during long-term cultures (i.e., >14 days), none of these ≥ 200 MPa-treated cells were able to yield up colonies (data not shown).
Figure 5. Colony-forming ability of tumor cells after high hydrostatic pressure (HHP) treatment. Line plot represents the percentage of colony-forming units (CFU) [normalized to the CFU associated with untreated (mock) cells] of MCF7, B16-F10, and CT26 cells after HHP treatment. Representative data (expressed as mean ± SD) from independent experiments—each performed in triplicate—are displayed.
![Figure 5. Colony-forming ability of tumor cells after high hydrostatic pressure (HHP) treatment. Line plot represents the percentage of colony-forming units (CFU) [normalized to the CFU associated with untreated (mock) cells] of MCF7, B16-F10, and CT26 cells after HHP treatment. Representative data (expressed as mean ± SD) from independent experiments—each performed in triplicate—are displayed.](/cms/asset/df907107-bfbc-43be-bfe3-7848a34df8bf/iimt_a_466250_f0005_b.gif)
Analysis of the immunogeneic potential of HHP-treated tumor cells
For the analysis of the immunogeneic potential of the HHP-treated tumor cells, a xenogeneic as well as a syngeneic mouse model was used. In this study, C57BL/6 or Balb/c mice were immunized with treated human Raji cells () or murine CT26 cells (), respectively. Both viable (untreated) and pressure-inactivated xenogeneic Raji cells exhibited an immunogenic potential that was evidenced by an increase in the levels of Raji-specific IgG antibodies present in the blood serum (in comparison to the IgG levels found in the serum of Ringer’s solution-injected mice) (). Of all the treatments evaluated using this Raji-specific IgG endpoint, 500 MPa caused the most potent immune response. Still, and most importantly, HHP-treated cells preserved their immunogenicity, even when treated with 200 or 300 MPa.
Figure 6. Effects on immunogenicity of xenogeneic and syngeneic high hydrostatic pressure (HHP)-treated tumor cells. Specific IgG antibodies against (A) xenogeneic and (C) syngeneic tumor cells in sera of immunized mice were detected using flow cytometry. (A) C57BL/6 mice were immunized with viable or HHP-treated Raji cells, and (C) Balb/c mice with syngeneic HHP-treated CT26 tumor cells. Serum derived from mice that were injected with Ringer solution served as the control. (B) Delayed-type hypersensitivity test (DTH) response (evaluated by measuring swelling of mice footpads) after injection of xenogeneic tumor cells. (D) Absolute IgG in serum after immunization of Balb/c mice with syngeneic CT26 tumor cells inactivated with the indicated pressure. Each open circle implies a single animal, while the black line is the median of all animals per group. w/o: untreated, viable cells; MFI: mean fluorescence intensity.
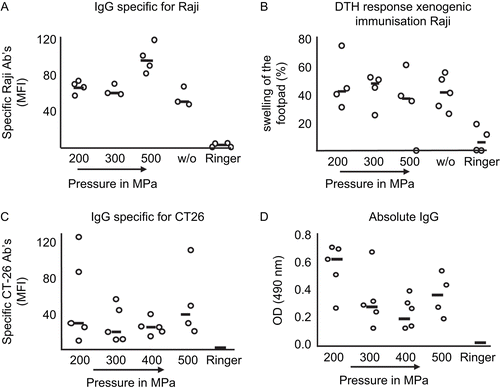
The results of the analyses of the T-lymphocyte reactions against the immunized cells using a DTH test are displayed in . All pressure-inactivated cells maintained their immunogenicity. It should be noted that the xenogeneic immune reaction to viable cells was always strong and that the inactivation of the xenogeneic tumor cells did not diminish the immune response against them. On the basis of the testing of the immunogenicity of pressure-inactivated xenogeneic cells, additional tests were also performed in a syngeneic situation using Balb/c mice immunized three times with HHP-treated CT26 cells.
The immunogenicity of the syngeneic tumor cells was analyzed via the formation of specific IgG antibodies against CT26 cells (as well as the absolute IgG amounts) in the immunized mice, as a degree of immunological memory. Note, for the detection of immunogeneic properties of xenogeneic and syngeneic cells, only tumor cells treated with ≥ 200 MPa (a level leading to no colony formation; see ) were used. Balb/c mice that were injected with Ringer’s solution served as a control group during these experiments. As illustrated in , immunization with HHP-treated tumor cells led to an increase in the absolute IgG content in sera in comparison to the level noted in control hosts. In this setting, an immunization with cells treated with 200 MPa exhibited the most favorable results. A pressure treatment with 500 MPa led to a slightly higher IgG content after immunization than did cells that received a treatment with 300 or 400 MPa. Additional clues that HHP-treated tumor cells induced the production of tumor cell-specific antibodies are illustrated in . Specifically, immunization with pressure-treated cells resulted in an increased amount of CT26-specific antibodies in the sera of the immunized mice (in comparison to levels noted in mice that received only a Ringer’s injection). Taken together, these results appear to suggest that an anti-tumor immune response can be induced in mice using immunization with pressure (HHP)-treated cells.
Discussion
Recent work has pointed out the crucial role of the immune system in the therapy of malignancies. Radiotherapy and chemotherapy have been shown to contribute to the development of anti-tumor immunity (Tesniere et al., Citation2008); the latter modality may lead to specific and long-lasting tumor control. In the development of multimodal concepts for the treatment of malignant diseases, additional cancer immunotherapies—such as tumor vaccines—should be taken into account (Yannelli and Wroblewski, Citation2004). One promising example of the vaccination approach is the treatment of cancer patients with autologous tumor cells (de Gruijl et al., Citation2008). The purpose of using whole tumor cells for such a vaccination is the presence of a plethora of antigens in the vaccine. Our research group recently outlined a general scheme that explains how dead tumor cells—alone or in combination with other immune activating substances (like the protein AxV)—can be applied as a tumor vaccine (Frey et al., Citation2009).
For this potential anti-cancer therapy, the inactivation of the tumor cells is one of the most crucial steps. As already pointed out, this inactivation has to be safe (i.e., non-metastogenic), should induce highly immunogenic tumor cells, and has to be in accordance with legal requirements. Ultimately, both the safe inactivation and the high immunogenicity of the treated cells may correlate directly with the therapeutic effect of the vaccine. Of these two parameters, the maintenance of the immunogenicity of the inactivated cells seems to be the biggest challenge during whole-cell–based tumor vaccine development. It seems clear that whether a dying cell is immunogenic or not depends on the death-initiating stimulus and does not simply correlate with the cell death form (Kepp et al., Citation2009).
In earlier investigations, we demonstrated that a HHP approach is a safe, low cost, easy-to-apply technique for the inactivation of tumor cells to be used for vaccination purposes. Those studies showed that the treatment of tumor cells with HHP inactivated the cells and induced unique cell death pathways that were related to apoptosis and/or necrosis (Frey et al., Citation2004, Citation2008; Korn et al., Citation2004). The main issue in the present study was to investigate whether mammalian tumor cells could be efficiently inactivated by HHP while preserving their immunogenicity.
The studies here also focused on the form of cell death after HHP treatment; dying and dead cells are strong modulators of the immune system and the immunogenic properties of the cells depend mostly on the death-inducing stimulus (Matzinger, Citation1994; Voll et al., Citation1997; Gallucci and Matzinger, Citation2001; Gaipl et al., Citation2003b, Citation2007; Munoz et al., Citation2007; Kepp et al., Citation2009). Apoptotic cells contribute mostly to anti-inflammatory immune reactions; in stark contrast, necrotic cells mostly drive inflammatory processes (Gaipl et al., Citation2007). FSc/SSc and AxV/PI analyses here demonstrated that an exposition of cells to a pressure of 100 MPa hardly affected the viability of the cells. However, HHP treatment of cells with a pressure of 300 MPa and above induced a strong reduction of cell viability, mainly leading to necrotic cell death. The HHP treatment of B16-F10 cells with 200 MPa induced necrosis in > 95% of the cells as early as 24 h after initiation of culture. MCF7 and CT26 cells displayed a similar trend, but to a reduced extent. The CT26 cells, in particular, displayed a higher amount of apoptotic cells in comparison to the other cell lines.
The kinetics of the deaths of these cells assumes a programmed fashion of death, as seen in the case of apoptosis, autophagy, and programmed necrosis (Kroemer et al., Citation2009). During the analyses of the DNA content of 200 MPa–treated B16-F10 cells, an increasing amount of degraded sub-G1 DNA was detectable during the post-treatment culture period. This finding is a sign that the DNA degradation was a consequence of DNA fragmentation that arose during apoptosis and not via an effect directly induced by HHP. A pressure ≥ 300 MPa led to necrotic cells, with degraded DNA being found in all three tumor cell lines at least 1 day after the HHP treatment. This effect was the most intensive in the CT26 cells. Degraded DNA is not typical within primary necrotic cells when the necrosis was induced by a “common” stimulus like heat or hydrogen peroxide (Frey et al., Citation2004). However, because Ca2+-dependent DNAses are not inactivated by HHP, this leads to further degradation of DNA in cells that have already lost their membrane integrity (Frey et al., Citation2004). The in vitro clonogenic “survival” test revealed an effective “stop” in the proliferation of tumor cells after an HHP treatment of ≥ 200 MPa. In fact, none of these HHP-treated cells was able to form new colonies. Though not shown in this paper, the same results can be obtained in vivo. Taken together, these data demonstrate that HHP treatment is an effective technique to inactivate tumor cells without the need of any additives.
The next goal of the current studies was to test the immunogenicity of the HHP-inactivated tumor cells. As already outlined, a vaccination with intact (whole) tumor cells should offer the immune system an undefined number of antigens; this, in turn, may enable such a vaccine to confer a long(er)-lasting anti-tumor immunity in the treated host (Copier et al., Citation2007). Accordingly, the immunogenicity of cells (after treatments at different pressure levels) in a xenogeneic as well as in a syngeneic mouse model system was tested. Results from our earlier studies (Korn et al., Citation2004) showed that serum antibodies against xenogeneic Raji cells could be induced by vaccination with HHP-treated cells. In that 2004 study, the level of specific IgG against the tumor cells was not analyzed. In the current study, it was shown that the amount of specific IgG antibodies against the HHP-treated xenogeneic cells was not diminished in comparison to the amounts attained using viable cells. That the repellent T-lymphocyte reaction (measured via a DTH response) against the HHP-treated xenogeneic cells was preserved was also evident. Furthermore, it was also shown for the first time that syngeneic HHP-treated CT26 cells used for immunization induced increased amounts of specific IgG antibodies in immunized mice. In addition, this immunization with HHP-treated syngeneic cells also increased the amount of absolute IgG. These outcomes suggested to us that the HHP-treated tumor cells maintained their immunogenicity, and that those cells that died mainly did by a distinct form of necrosis and concurrently completely lost their clonogenic potential.
Our earlier experiments also revealed that HHP-treated cells preserve their shape for over a period of weeks; this feature seems to be very important for the generation of a potent vaccine (Frey et al., Citation2008). It was also previously shown that this pressure treatment gave rise to a drastic increase in the viscosity of the treated cell’s cytoplasm (Frey et al., Citation2004). We speculate that the increased viscosity of the necrotic tumor cells leads to a retarded—but constant—release of cell-derived antigens and danger signals. Both of these features are essential for the effective uptake of tumor cells, and subsequent presentation of tumor antigens, by DC, processes that lead to specific anti-tumor immunity (Apte and Voronov, Citation2008). These findings further broaden the potential utility of applying the HHP technique in the generation of vaccines.
Each of the above immune response-“boosting” parameters discussed here reflects upon the potential utility of the HHP approach for generating whole-tumor cell vaccines. However, there are other attributes of this technique that should make it more and more acceptable for use by investigators (Shearer and Kniel, Citation2009). For example, the use of HHP in the preparation of vaccines offers an inactivation procedure that—in comparison to traditional methods—is fast, less expensive, and avoids the use of hazardous substances. Furthermore, during HHP inactivation, the pressure is (1) distributed uniformly and instantaneously throughout the media (water or non-toxic oil); (2) applied isostatically, i.e., every part of the treated sample is subjected to the same amount of pressure at the same time; and, (3) propagated through all flexible wrappings (Cheftel, Citation1992; Patterson, Citation2005). Because every treated material is subjected to the same pressure amount, any gradients—especially those that might be created during the treatment of clumpy material (e.g., solid tumor pieces)—can be mitigated. Ultimately, because every treated cell in the system is subjected to exactly the same stress, very high levels of reproducibility are able to be achieved.
These features of the HHP technique, when placed in the context of the new results being presented here, should lead researchers to believe that HHP can be successfully and properly used as a technique for the preparation of whole-cell based tumor vaccines.
Declaration of interest
This work was supported by: the ELAN fond (08.03.12.1) of the University Erlangen-Nuremberg, Germany; the Doktor Robert Pfleger Foundation, Bamberg, Germany; the Interdisciplinary Center for Clinical Research (IZKF) at the University Hospital of the University of Erlangen-Nuremberg; the European Commissions [NOTE (TPA4 FP6)]; and, the Foundation of Berdelle-Hilge, Bodenheim, Germany. The authors report no conflicts of interest. The Authors alone are responsible for the content and writing of the paper.
References
- Aertsen, A., Meersman, F., Hendrickx, M. E., Vogel, R. F., and Michiels, C. W. 2009. Biotechnology under high pressure: Applications and implications. Trends Biotechnol. 27:434–441.
- Amato, R. J. 2003. Vaccine therapy for renal cell carcinoma. Rev. Urol. 5:65–71.
- Apetoh, L., Tesniere, A., Ghiringhelli, F., Kroemer, G., and Zitvogel, L. 2008. Molecular interactions between dying tumor cells and the innate immune system determine the efficacy of conventional anti-cancer therapies. Cancer Res. 68:4026–4030.
- Apte, R. N., and Voronov, E. 2008. Is interleukin-1 a good or bad ‘guy’ in tumor immunobiology and immunotherapy? Immunol. Rev. 222:222–241.
- Balny, C. 1999. High-pressure enzyme kinetics. In Advances in High-Pressure Bioscience and Biotechnology (Ludwig, H., Ed.), Berlin: Springer Verlag, pp. 261–266.
- Balny, C., Hayashi, R., Heremans, K., and Masson, P. (Eds.) 1992. High-Pressure and Biotechnology, Montrouge: Editions John Libbey Eurotext Ltd.
- Balny, C., Masson, P., and Heremans, K. 2002. High-pressure effects on biological macromolecules: From structural changes to alteration of cellular processes. Biochim. Biophys. Acta 1595:3–10.
- Bocchia, M., Bront, V., Colombo, M. P., Dd Vincentiis, A., Di Nicola, M., Forni, G., Lanata, L., Lemoli, R. M., Massaia, M., Rondelli, D., Zanon, P., and Tura, S. 2000. Anti-tumor vaccination: Where we stand. Haematologica 85:1172–1206.
- Boonyaratanakornkit, B. B., Park, C. B., and Clark, D. S. 2002. Pressure effects on intra- and intermolecular interactions within proteins. Biochim. Biophys. Acta 1595:235–249.
- Cheftel, J. C. 1992. Effects of high hydrostatic pressure on food constituents: An overview. In High-Pressure and Biotechnology (Balny, C., Hayashi, R., Heremans, K., and Masson, P., Eds.), Montrouge: INSERM/John Libbey Eurotext Ltd., pp. 195–209.
- Chen, X., Chang, C. H., and Goldenberg, D. M. 2009. Novel strategies for improved cancer vaccines. Expert Rev. Vaccines 8:567–576.
- Copier, J., Ward, S., and Dalgleish, A. 2007. Cell based cancer vaccines: Regulatory and commercial development. Vaccine 25(S2):B35–46.
- De Gruijl, T. D., Van den Eertwegh, A. J., Pinedo, H. M., and Scheper, R. J. 2008. Whole-cell cancer vaccination: From autologous to allogeneic tumor- and dendritic cell-based vaccines. Cancer Immunol. Immunother. 57:1569–1577.
- Diehl, P., Schauwecker, J., Mittelmeier, W., and Schmitt, M. 2008. High hydrostatic pressure, a novel approach in orthopedic surgical oncology to disinfect bone, tendons and cartilage. Anticancer Res. 28:3877–3883.
- Diehl, P., Schmitt, M., Bluemelhuber, G., Frey, B., Van Laak, S., Fischer, S., Muehlenweg, B., Meyer-Pittroff, R., Gollwitzer, H., and Mittelmeier, W. 2003. Induction of tumor cell death by high hydrostatic pressure as a novel supporting technique in orthopedic surgery. Oncol. Rep. 10:1851–1855.
- Dillman, R. O., Depriest, C., Deleon, C., Barth, N. M., Schwartzberg, L. S., Beutel, L. D., Schiltz, P. M., and Nayak, S. K. 2007. Patient-specific vaccines derived from autologous tumor cell lines as active specific immunotherapy: Results of exploratory Phase I/II trials in patients with metastatic melanoma. Cancer Biother. Radiopharm. 22:309–321.
- Dubins, D. N., Lee, A., Macgregor, R. B., Jr., and Chalikian, T. V. 2001. On the stability of double stranded nucleic acids. J. Am. Chem. Soc. 123:9254–9259.
- Elstein, K. H., and Zucker, R. M. 1994. Comparison of cellular and nuclear flow cytometric techniques for discriminating apoptotic sub-populations. Exp. Cell Res. 211:322–331.
- Esche, C., Shurin, M. R., and Lotze, M. T. 1999. The use of dendritic cells for cancer vaccination. Curr. Opin. Mol. Ther. 1:72–81.
- Franz, S., Herrmann, K., Furnrohr, B. G., Sheriff, A., Frey, B., Gaipl, U. S., Voll, R. E., Kalden, J. R., Jack, H. M., and Herrmann, M. 2007. After shrinkage apoptotic cells expose internal membrane-derived epitopes on their plasma membranes. Cell Death Differ. 14:733–742.
- Frey, B., Franz, S., Sheriff, A., Korn, A., Bluemelhuber, G., Gaipl, U. S., Voll, R. E., Meyer-Pittroff, R., and Herrmann, M. 2004. Hydrostatic pressure induced death of mammalian cells engages pathways related to apoptosis or necrosis. Cell. Mol. Biol. (Noisy-le-grand) 50:459–467.
- Frey, B., Janko, C., Ebel, N., Meister, S., Schlucker, E., Meyer-Pittroff, R., Fietkau, R., Herrmann, M., and Gaipl, U. S. 2008. Cells under pressure - treatment of eukaryotic cells with high hydrostatic pressure, from physiologic aspects to pressure-induced cell death. Curr. Med. Chem. 15:2329–2336.
- Frey, B., Schildkopf, P., Rodel, F., Weiss, E. M., Munoz, L. E., Herrmann, M., Fietkau, R., and Gaipl, U. S. 2009. Annexin A5 renders dead tumor cells immunogenic - implications for multimodal cancer therapies. J. Immunotoxicol. 6:209–216.
- Gaipl, U. S., Beyer, T. D., Baumann, I., Voll, R. E., Stach, C. M., Heyder, P., Kalden, J. R., Manfredi, A., and Herrmann, M. 2003a. Exposure of anionic phospholipids serves as anti-inflammatory and immunosuppressive signal - implications for anti-phospholipid syndrome and systemic lupus erythematosus. Immunobiology 207:73–81.
- Gaipl, U. S., Brunner, J., Beyer, T. D., Voll, R. E., Kalden, J. R., and Herrmann, M. 2003b. Disposal of dying cells: A balancing act between infection and autoimmunity. Arthritis Rheum. 48:6–11.
- Gaipl, U. S., Munoz, L. E., Rodel, F., Pausch, F., Frey, B., Brachvogel, B., Von der Mark, K., and Poschl, E. 2007. Modulation of the immune system by dying cells and the phosphatidylserine-ligand Annexin A5. Autoimmunity 40:254–259.
- Gallucci, S., and Matzinger, P. 2001. Danger signals: SOS to the immune system. Curr. Opin. Immunol. 13:114–119.
- Hacker, G. 2000. The morphology of apoptosis. Cell Tissue Res. 301:5–17.
- Heden, C. G. 1964. Effects of hydrostatic pressure on microbial systems. Bacteriol. Rev. 28:14–29.
- Heden, C. G., and Rupprecht, A. 1966. Semi-conductivity of dried oriented DNA. Acta Chem. Scand. 20:583–585.
- Jocham, D., Richter, A., Hoffmann, L., Iwig, K., Fahlenkamp, D., Zakrzewski, G., Schmitt, E., Dannenberg, T., Lehmacher, W., Von Wietersheim, J., and Doehn, C. 2004. Adjuvant autologous renal tumor cell vaccine and risk of tumor progression in patients with renal-cell carcinoma after radical nephrectomy: Phase III randomized controlled trial. Lancet 363:594–599.
- Kepp, O., Tesniere, A., Schlemmer, F., Michaud, M., Senovilla, L., Zitvogel, L., and Kroemer, G. 2009. Immunogenic cell death modalities and their impact on cancer treatment. Apoptosis 14:364–375.
- Korn, A., Frey, B., Sheriff, A., Gaipl, U. S., Franz, S., Meyer-Pittroff, R., Bluemelhuberh, G., and Herrmann, M. 2004. High hydrostatic pressure-inactivated human tumor cells preserve their immunogenicity. Cell. Mol. Biol. (Noisy-le-grand) 50:469–477.
- Kroemer, G., Galluzzi, L., Van den Abeele, P., Abrams, J., Alnemri, E. S., Baehrecke, E. H., Blagosklonny, M. V., El-Deiry, W. S., Golstein, P., Green, D. R., Hengartner, M., Knight, R. A., Kumar, S., Lipton, S. A., Malorni, W., Nunez, G., Peter, M. E., Tschopp, J., Yuan, J., Piacentini, M., Zhivotovsky, B., and Melino, G. 2009. Classification of cell death: Recommendations of the Nomenclature Committee on Cell Death 2009. Cell Death Differ. 16:3–11.
- Krysko, D. V., D’Herde, K., and Van den Abeele P. 2006. Clearance of apoptotic and necrotic cells and its immunological consequences. Apoptosis 11:1709–1726.
- Macgregor, R. B. 2002. The interactions of nucleic acids at elevated hydrostatic pressure. Biochim. Biophys. Acta 1595:266–276.
- Masson, P., Tonello, C., and Balny, C. 2001. High-pressure biotechnology in medicine and pharmaceutical science. J. Biomed. Biotechnol. 1:85–88.
- Matzinger, P. 1994. Tolerance, danger, and the extended family. Annu. Rev. Immunol. 12:991–1045.
- Meyer-Pittroff, R., Behrendt, H., and Ring, J. 2006. Specific immunomodulation and therapy by means of high-pressure-treated allergens. High Pressure Res. 26:1–5.
- Moingeon, P., De Taisne, C., and Almond, J. 2002. Delivery technologies for human vaccines. Br. Med. Bull. 62:29–44.
- Munoz, L. E., Frey, B., Pausch, F., Baum, W., Mueller, R. B., Brachvogel, B., Poschl, E., Rodel, F., Von der Mark, K., Herrmann, M., and Gaipl, U. S. 2007. The role of Annexin A5 in the modulation of the immune response against dying and dead cells. Curr. Med. Chem. 14:271–277.
- Nicoletti, I., Migliorati, G., Pagliacci, M. C., Grignani, F., and Riccardi, C. 1991. A rapid and simple method for measuring thymocyte apoptosis by propidium iodide staining and flow cytometry. J. Immunol. Meth. 139:271–279.
- Ott, O. J., Rodel, C., Weiss, C., Wittlinger, M., St., Krause, F., Dunst, J., Fietkau, R., and Sauer, R. 2009. Radiochemotherapy for bladder cancer. Clin. Oncol. (R. Coll. Radiol.) 21:557–565.
- Patterson, M. F. 2005. Microbiology of pressure-treated foods. J. Appl. Microbiol. 98:1400–1409.
- Paukovits, P., Himmler, G., and Loibner, H. 2004. Immunotherapy of malignant diseases -developments and prospects. Wien Med. Wochenschr. 154:235–241.
- Rosenberg, S. A., Yang, J. C., and Restifo, N. P. 2004. Cancer immunotherapy: Moving beyond current vaccines. Nat. Med. 10:909–915.
- Rosenblatt, J., and Avigan, D. 2008. Cellular immunotherapy for multiple myeloma. Best Pract. Res. Clin. Haematol. 21:559–577.
- San Martin, M. F., Barbosa-Canovas, G. V., and Swanson, B. G. 2002. Food processing by high hydrostatic pressure. Crit. Rev. Food Sci. Nutr. 42: 627–645.
- Shearer, A. E., and Kniel, K. E. 2009. High hydrostatic pressure for development of vaccines. J. Food Prot. 72:1500–1508.
- Smeller, L. 2002. Pressure-temperature phase diagrams of biomolecules. Biochim. Biophys. Acta 1595:11–29.
- Stach, C. M., Turnay, X., Voll, R. E., Kern, P. M., Kolowos, W., Beyer, T. D., Kalden, J. R., and Herrmann, M. 2000. Treatment with Annexin V increases immunogenicity of apoptotic human T-cells in Balb/c mice. Cell Death Differ. 7:911–915.
- Tesniere, A., Apetoh, L., Ghiringhelli, F., Joza, N., Panaretakis, T., Kepp, O., Schlemmer, F., Zitvogel, L., and Kroemer, G. 2008. Immunogenic cancer cell death: A key-lock paradigm. Curr. Opin. Immunol. 20:504–511.
- Umansky, V., Malyguine, A., and Shurin, M. 2009. New perspectives in cancer immunotherapy and immunomonitoring. Future Oncol. 5:941–944.
- Vermes, I., Haanen, C., and Reutelingsperger, C. 2000. Flow cytometry of apoptotic cell death. J. Immunol. Meth. 243:167–190.
- Vermes, I., Haanen, C., Steffens-Nakken, H., and Reutelingsperger, C. 1995. A novel assay for apoptosis. Flow cytometric detection of phosphatidylserine expression on early apoptotic cells using fluorescein-labeled Annexin V. J. Immunol. Meth. 184:39–51.
- Voll, R. E., Herrmann, M., Roth, E. A., Stach, C., Kalden, J. R., and Girkontaite, I. 1997. Immunosuppressive effects of apoptotic cells. Nature 390:350–351.
- Winter, R. 2002. Synchrotron X-ray and neutron small-angle scattering of lyotropic lipid mesophases, model biomembranes, and proteins in solution at high pressure. Biochim. Biophys. Acta 1595:160–184.
- Winter, R., and Dzwolak, W. 2004. Temperature-pressure configurational landscape of lipid bilayers and proteins. Cell. Mol. Biol. (Noisy-le-grand) 50:397–417.
- Yannelli, J. R., Hirscowitz, E., and Wroblewski, J. M. 2003. Growth and functional reactivity of lymphocytes obtained from three anatomic compartments in patients with non-small-cell lung cancer (NSCLC). Cancer Biother. Radiopharm. 18:735–749.
- Yannelli, J. R., and Wroblewski, J. M. 2004. On the road to a tumor cell vaccine: 20 years of cellular immunotherapy. Vaccine 23:97–113.
- Yi, Q. 2003. Immunotherapy in multiple myeloma: Current strategies and future prospects. Expert Rev. Vaccines 2:391–398.