Abstract
In the present work, we designed an amperometric glucose biosensor based on nickel oxide nanoparticles (NiONPs)-modified carbon paste electrode. The biosensor was prepared by incorporation of glucose oxidase and NiONPs into a carbon paste matrix. It showed good analytical performances such as high sensitivity (367 μA mmolL–1) and a wide linear response from 1.9 × 10–3 mmolL–1 to 15.0 mmolL–1 with a limit of detection (0.11 μmolL–1). The biosensor was used for the determination of glucose in human serum samples. The results illustrate that NiONPs have enormous potential in the construction of biosensor for determination of glucose.
Introduction
Diabetes mellitus is a group of carbohydrate metabolic disorders affecting about 150 million people around the world. This disease leads to death and failure of several organs such as heart, kidneys, and eyes (Mercado and Moussy Citation1998, Moussy et al. Citation1994, Privett et al. Citation2008). This makes glucose biosensing very important because accurate and rapid determination of blood glucose is crucial for treatment and management of diabetic patients.
Conventional techniques for glucose determination such as chemiluminescence (Zhu et al. Citation2002), colorimetric (Wang et al. Citation2009), and capilary electrophoresis (Wang et al. Citation2011) are complex, expensive, and time consuming. In comparison, enzyme-based amperometric biosensors have advantages over nonenzymatic ones; such as the ease of operation, low cost of fabrication, high selectivity, and operational stability for real-time measurements (Guo et al. Citation2011).
In amperometric glucose biosensors, glucose oxidase (GOD) catalyzes the oxidation of glucose to gluconolactone and oxygen reduces to hydrogen peroxide (H2O2). An enzyme electrode for glucose determination is based on the detection of enzymatically generated H2O2 during the enzymatic reaction (Yang et al. Citation2009, Usman Ali et al. Citation2010).
The H2O2 can be oxidized or reduced directly at ordinary solid electrodes. However, these processes in analytical applications are limited by slow electrode kinetics and high overpotential which may incur large interferences from other existing electroactive species available in real samples such as ascorbic acid, uric acid, urea, paracetamol, etc. Thus, the determination of glucose is mainly focused on the electrode modifications in order to decrease the overpotential and increase the electron transfer kinetics. For this reason, a large range of materials such as redox mediators (Jiang et al. Citation2008), conducting polymers (Çolak et al. Citation2012), carbon nanotubes (Zargoosh et al. Citation2012) have been employed to construct of the electrochemical sensors for detection of glucose. On the other hand, in past decades, nanomaterials have attracted great research interest owing to their desirable chemical, physical, and electronic properties that are different from those of bulk materials.
Recently, NiONPs are considered as important metal oxides with attractive electrochemical, thermal, optical, and mechanical properties with their high surface to volume ratio (Lata et al. Citation2012, Hotovy et al. Citation2002). In constructing enzyme-based biosensors, NiONPs are favorable because of their high isoelectric point for physical adsorption of enzyme molecules by electrostatic interactions (Li et al. Citation2008). The electrochemical applications of NiONPs in glucose (Ding et al. Citation2011, Mu et al. Citation2011), myoglobin (Zong et al. Citation2010), DNA (Noorbakhsh and Salimi Citation2011), and H2O2 (Salimi et al. Citation2007a) sensors have been applied in various fields.
In the present study, we developed a new glucose biosensor based on NiONPs-modified carbon paste GOD electrode for amperometric detection of glucose. The optimum working conditions such as working potential, enzyme unit, pH, and temperature were determined. The analytical performance factors and application of the developed glucose biosensor to real samples were investigated.
Experimental
Chemicals and reagents
GOD (EC 1.1.3.4, from Aspergillus niger, EC 1.1.3.4 39680 Units/g solid) and nickel oxide nanoparticles (< 50 nm) were purchased from Sigma. Glucose, graphite powder, and paraffin oil were obtained from Fluka. Uric acid (UA), ascorbic acid, creatinine, urea, glucose, glutaraldeyde, and bovine serum albumine (BSA) were also purchased from Sigma. H2O2 (35%, v/v aqueous solution), di-sodium monohydrogen phosphate heptahydrate, and sodium dihydrogen phosphate dihydrate were acquired from Riedel de Haën. All other chemicals were of analytical grade and used without further purification. Glucose stock solutions were allowed to mutarotate for at least 24 h at room temperature prior to use and stored at 4°C. All solutions were prepared with ultrapure water.
Instrumentations
All electrochemical experiments were performed with a computer controlled on IVIUM-STAT electrochemical analyzer (Ivium Technologies, The Netherlands) and a three-electrode cell stand (Bioanalytical Systems, Inc., USA). A conventional three-electrode system comprises with a modified carbon paste electrode as the working electrode, a Pt wire (BAS MW 1034) as the counter electrode, and an Ag/AgCl including 3 mol L–1 NaCl (BAS MF 2052) as the reference electrode. Temperature control was accomplished with a Grant LTD GG thermostat. The pH values of the buffer solutions were measured with an ORION model 720A pH-ion meter. The ultrapure water (18.2 MΩ cm) was obtained from Elga Purelab device (Veolia Water Systems Ltd., UK).
Preparation of unmodified carbon paste and modified carbon paste electrodes
For unmodified carbon paste electrode (UCPE) construction, carbon paste was prepared by mechanically hand mixing graphite powder (70.0% w/w) and paraffin oil (30.0% w/w) until homogeneous paste was obtained. NiONPs-modified CPE (NiONPs–CPE) was prepared by mixing NiONPs with graphite powder in a ratio of 1:3 (w/w), followed by the incorporation of paraffin oil, and mixed regularly until a uniform paste was obtained. For the GOD enzyme and NiONPs incorpareted carbon-paste electrode (GOD–NiONPs–CPE), the enzyme solution containing various units of GOD, 10 μL of glutaraldehyde (1.25% (v/v)) and 1.0 mg BSA were added into the graphite powder–NiONPs mixture. Finally, carbon paste was obtained by mixing with 15 μL paraffin oil for 20 min. A portion of the given paste was placed firmly into a cavity of polyetheretherketone (PEEK) rod electrode bodies (with 3 mm internal diameter) and the surfaces of electrodes were smoothed on a weighing paper before starting new experiment. The obtained electrodes were stored in the refrigerator at +4°C when not in use.
Electrochemical measurements
Electrochemical behavior of the NiONPs–CPE was examined in 0.1 mol L–1 KCl solution containing 1 mmol L–1 Fe(CN)63–/4– by CV and EIS methods and compared to those of UCPE. The cyclic voltammograms (CVs) were recorded in potential range between –0.2 V and 0.6 V at different scan rates. The EIS studies were performed at the frequency range between 100 kHz and 0.01 Hz with 5 mV perturbation at open-circuit potential (EOCP).
Electrocatalytic activity of the NiONPs–CPE was investigated in the absence and presence of 1 mmol L–1 H2O2 in 0.1 mol L–1 phosphate buffer soltion (PBS, pH 7.0) by CV and chronoamperometry methods and compared to that of UCPE. The CVs were recorded in potential range between –0.3 V and 1.0 V at a 50 mVs–1 scan rate. The amperometric measurements were carried out at +0.4 V vs. Ag/AgCl.
Amperometric determination of glucose was performed by the oxidation of enzymatically produced H2O2 at + 0.4 V vs. Ag/AgCl in an aqueous solution containig 0.1 mol L–1 PBS (pH 7.0). First, the GOD enzyme electrode was equilibrated in PBS at +0.4 V until a constant current (i1), which was called as background current, was obtained. Then, aliquots of glucose solution were added to electrochemical cell. The steady-state current response (i2) to the addition of glucose was recorded and the current difference (Δi = i2–i1) was determined with the change between the steady-state current and the background current. A calibration curve of Δi–glucose concentration was plotted.
Results and discussion
The effect of the quantity of NiONPs on the performance of the modified CPE electrode
The quantity of NiONPs to be added into carbon paste would affect the performance of the modified electrode, so the NiONPs–CPEs were prepared at the various mass ratio of NiONPs/graphite (1:5, 1:4, 1:3, and 1:2). In order to examine the electron transfer features of the prepared NiONPs–CPEs were used Fe(CN)63–/4– as redox probe. The cyclic voltammetry of ferricyanide is a useful tool to monitor the electrochemical behavior of the modified electrodes (Liu et al. Citation2006). shows the CVs of the NiONPs–CPEs in the solution of redox couple. A pair of redox waves of Fe(CN)63–/4– was observed between 0.0 V and 0.4 V at all electrodes. Both cathodic peak current and anodic peak current generally increase with increasing quantity of NiONPs. It can be said that NiONPs act as an electrocatalyst. The electrochemical data obtained from are presented . While the anodic and cathodic peak currents at the ratios of 1:3 and 1:4 are the highest, the peak-to-peak potential separation (ΔEp) between the cathodic and anodic waves at the ratio of 1:4 is the lowest. The NiONPs–CPEs prepared with the ratios of 1:3 and 1:4 exhibited the comparatively lowest ΔEp and highest peak currents, indicating fast electron transfer kinetics. To determine the most appropriate combination of electrode, the amperometric responses of the three NiONPs–CPEs to the successive addition of H2O2 were recorded at an applied potential of +0.4 V. displays the relationships between Δi and H2O2 concentration (0.20–0.91 mmol L–1). The highest sensitivity was obtained when the NiONPs–CPE at the ratio of 1:3 was used. The mass ratio of NiONPs and graphite powder are significant for electrochemical detection based on the oxidation of H2O2. Furthermore, a large amount of nanoparticles incorporated into the carbon paste can lead to unfavorable mechanical properties of CPE and increase the resistance of the electrode (Li et al. Citation2011).
Figure 1. (A) CVs of 1 mmol L–1 Fe(CN)63–/4– on the various NiONPs–CPEs with the mass ratios of NiONPs to graphite powder were 1:2 (a), 1:3 (b), 1:4 (c), and 1:5 (d) (Scan rate: 50 mV s–1), (B) the plot of the peak current vs. H2O2 concentration for the various NiONPs‐CPEs in 0.1 M PBS (pH 7.0) at 0.4 V vs. Ag/AgCl.
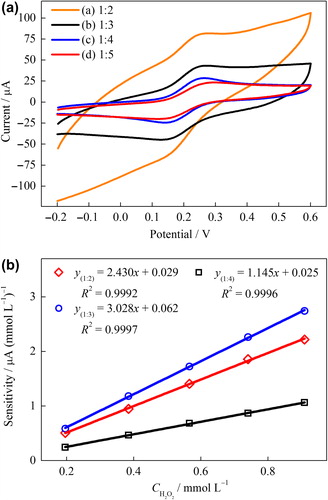
Table I. Comparisons of electrochemical characteristics of the NiONPs–CPEs were prepared at the various mass ratio of NiONPs/graphite toward the redox probe of Fe(CN)63–/4– (scan rate = 50 mVs–1).
Electrochemical properties of unmodified and modified electrodes
The effect of NiONPs on the electron transfer features of carbon paste electrode was investigated in KCl solution containing Fe(CN)63–/4–. The CVs of the UCPE and NiONPs–CPE were shown in . The redox peaks of Fe(CN)63–/4– were clearly observed for UCPE and NiONPs–CPE. The current intensity of the redox waves for the NiONPs–CPE extremely was increased when compared to that of the UCPE. In addition, while the peak-to-peak separation (Ep,a−Ep,c = ΔEp) of Fe(CN)63–/4– waves for the UCPE was 320 mV, the ΔEp for the NiONPs‐CPE was 100 mV. These results denoted that NiONPs provide the increasing electron transfer at solution/electrode interface due to their electrocatalytic and conductivity properties and/or the extended electroactive surface area of the modified electrode (Yao and Shiu Citation2007, Zhang et al. Citation2009, Zheng and Zhang Citation2007). The effect of scan rate on the voltammetric response of the NiONPs–CPE was shown in . As the scan rate increases, the peak currents increase while the redox peak potentials shift slightly. The anodic and cathodic peak currents (, inset) exhibit a linear dependence on the square root of the scan rate, indicating the mass transfer phenomenon at the electrochemical probe and the electrode interface is mainly a diffusion-controlled process (Du et al. Citation2007, Song et al. Citation2010).
Figure 2. (A) CVs of the UCPE (a) and NiONPs–CPE (b) at scan rate 10 mVs–1, (B) the plot of the peak current vs. the square root of the scan rate for NiONPs‐CPE (inset: CVs at different scan rates) in 0.1 mol L–1 KCl solution containing 1 mmol L–1 Fe(CN)63–/4–.
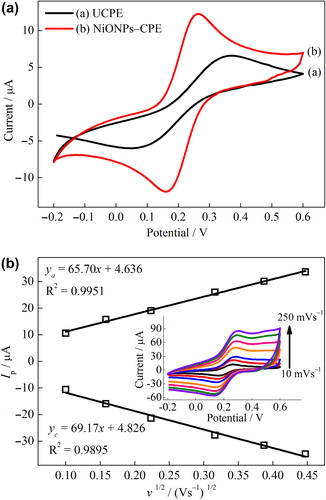
The EIS is an effective technique to investigate the interface properties of modified electrodes (Wang Citation2006b). The electrochemical impedance spectra (Nyquist curves) of the UCPE and NiONPs–CPE are shown in . The Nyquist curves include a semicircle portion at high frequencies and a linear portion at low frequencies. The semicircle portion and the linear portion correspond to the electron transfer limited process and the diffusion process, respectively. The semicircle diameter is equal to electron transfer resistance (Ret) value (Wang Citation2006b). The UCPE has a large semicircle with a large electron transfer resistance (Ret) of approximately 16 kΩ (). Whereas, the NiONPs–CPE exhibits almost a straight line, indicating that NiONPs provide fast electron transfer kinetics for the Fe(CN)63–/4– redox system. This is attributable to the good electron transfer ability of NiONPs which can facilitate the electrochemical oxidation of H2O2 on the electrode surface (Li et al. Citation2011).
Electrocatalytic activity of unmodified and NiONPs-modified electrodes
The electrocatalytic characteristic of the NiONPs–CPE electrode was examined using cyclic voltammetry (CV) and chronoamperometry (CA) methods for H2O2 oxidation and compared to that of UCPE. exhibits the CVs of NiONPs–CPE and UCPE in the absence and presence H2O2 (1 mmol L–1). As seen in and (c), while a small background current was obtained for the UCPE in PBS in the potential range from −0.3 to 1.0 V, a significant increase of current response was obtained for the NiONPs–CPE. It is indicated that the NiONPs have excellent electrochemical properties, which could be ascribed to the large surface area, high electrical conductivity and enhanced electron transfer of NiONPs (Noorbakhsh and Salimi Citation2011, Zhang et al. Citation2009) Furthermore, a pair of redox couples due to the Ni2+/Ni3+ redox process was observed between 0.4 and 0.9 V at the NiONPs–CPE (Adekunle and Ozoemena Citation2008, Adekunle and Ozoemena Citation2010). Upon the addition of 1 mmol L–1 H2O2, a little increase of current intensity at the UCPE was observed after about + 0.6 V, indicating the oxidation of H2O2 (). On the other hand, a remarkably increase of current intensity at the NiONPs–CPE was shown after about +0.2 V, which could be attributed to the electrocatalytic activity of NiONPs () (Zheng and Zhang Citation2007). The developed behavior for H2O2 oxidation on the surface of NiONPs–CPE may be due to an increase in the rate of electron transfer from H2O2 to the electrode surface incorporated the NiONPs. The NiONPs–CPE also provided a noticeable decrease in the overpotential for H2O2 oxidation. This indicates that it allows amperometric detection of H2O2 at low potential.
Figure 4. (A) CVs of the UCPE and NiONPs–CPE in the absence and presence of 1 mmol L–1 H2O2 (scan rate = 50 mVs–1) in 0.1 mmol L–1 PBS (pH 7.0). (B) Amperometric responses of the UCPE and NiONPs–CPE for the oxidation of H2O2 in 0.1 M PBS (pH 7.0) at 0.4 V vs. Ag/AgCl.
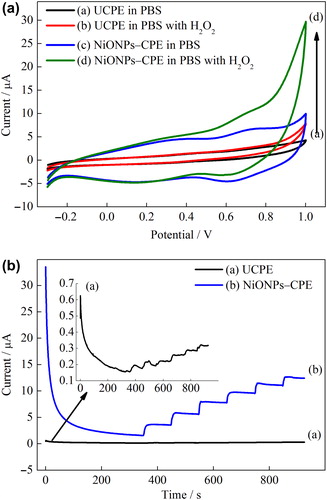
In order to investigate further the electrocatalytic activity of the NiONPs-modified electrode, amperometric measurements were performed at the NiONPs–CPE. shows the amperometric responses to H2O2 of the NiONPs–CPE and UCPE to the successive addition of H2O2 at an applied potential of 0.4 V. As seen in , the current responses to H2O2 of the NiONPs–CPE were higher than that of the UCPE. The NiONPs-modified electrode developed the current density corresponds to the oxidation of H2O2, indicating the electrocatalytic activity of NiONPs. Consequently, the NiONPs can be used for construction of sensors including amperometric detection of H2O2 at low potential.
Effect of working potential on the response of biosensor
The dependency of the biosensor response on the working potential was carried out in 0.1 mol L–1 PBS, pH 7.0. shows the effect of working potential on the amperometric response of the biosensor in the potential ranging from 0.3 to 0.6 V using varying H2O2 concentrations from 0.2 mmol L–1 to 1.38 mmol L–1. As expected, the current response of the biosensor increased with the applied potential up to a value of 0.6 V vs. Ag/AgCl. However, the interference effect of the most common species, present in many body fluids, is remarkably considered at high potentials. Accordingly, a potential of a 0.4 V, where the interference species are not oxidizable and resulting in less interference, was used in all measurements (Xu et al. Citation2004).
Effect of enzyme unit, pH and temperature on the response of biosensor
The sensitivities of the biosensor were determined at different GOD units (5–15 U). The effect of enzyme loading on biosensor sensitivity was investigated by measuring the different GOD concentrations (5–15 U)-loaded biosensors responses to sequential glucose increments from 0.02 to 0.20 mmol L–1. The other parameters were kept constant at +0.4 V. As expected from the increased biocatalytic activity of the electrode, the sensitivity was enhanced with the increasing of the amount of enzyme in the carbon paste matrix. Although the maximum sensitivity was obtained at 15 U GOD loading, 12 U was selected due to negligible increment (Supplementary Figure 1 to be found online at http://www.informahealthcare.com/doi/abs/10.3109/21691401.2013.808649).
The influence of the pH was checked in 0.1 mol L–1 PBSs with glucose increments from 0.02 to 0.20 mmol L–1 in the range of pH 5.0–9.0. As can be seen from , the sensitivity of the biosensor was increased with the pH value was increased up to pH 7.5. The immobilized GOD presented maximum activity at pH 7.5. This is in agreement with that reported in literatures (Pan et al. Citation2004, Li and Lin Citation2007). However, pH 7.0 was selected in the glucose detection due to its linear working range was relatively wider than that of others (not shown).
Figure 6. The sensitivity of the GOD–NiONPs–CPE as a function of buffer pH in 0.1 mmol L–1 PBS at 0.4 V vs. Ag/AgCl.
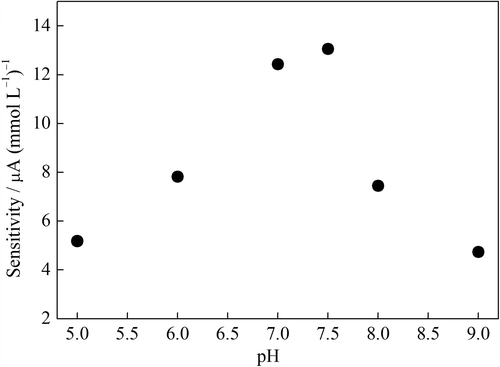
The increment in the response of sensors could be affected by temperature increases due to enhanced enzymatic activity, increased membrane permeability of various participating species (Vaddiraju et al. Citation2011). Investigation of the temperature dependency on the response of the glucose biosensor was obtained by measuring the electrode responses to sequential glucose increments from 0.02 to 0.20 mmol L–1 maintained at the desired temperature (10–50°C). The sensitivity of the biosensor increased with increasing of temperature until 45°C, and then, it declined slowly (Supplementary Figure 2 to be found online at http://www.informahealthcare.com/doi/abs/10.3109/21691401.2013.808649). The decline in the response of sensors could be due to denaturation of GOD. However, for practical convenience, the temperature of 25°C was selected as a working temperature for all further experiments.
Effect of glucose concentration on the response of biosensor
The effect of the glucose concentration on the amperometric response was studied using varying concentration of glucose (1 × 10–5 –1 × 10–1 mol L–1). As seen in , two linear working ranges were obtained. The inset in shows a good linear response in the range of 1.9 × 10–3 –9.1 × 10–3 mmol L–1 glucose with a correlation coefficient of 0.966 and the regression equation is I (μA) = 367.13Cglucose + 13.05. Also, a good linear response in the range of 1.31–15.00 mmol L–1 glucose with a correlation coefficient of 0.966 was obtained () and the regression equation is I (μA) = 0.15Cglucose + 18.80. Forzani et al. have reported that the normal clinical range for glucose in blood is between 3.5 and 6.1 mmol L–1 (Forzani et al. Citation2004). The linear range of the GOD–NiONPs–CPE covers the normal serum glucose level.
Figure 7. (A) Calibration curve of the GOD–NiONPs–CPE for glucose in 0.1 M PBS (pH 7.0) at 0.4 V vs. Ag/AgCl. (inset: linear working ranges plots), (B) Substrate concentration/current response vs. substrate concentration plot (Hanes–Woolf plot).
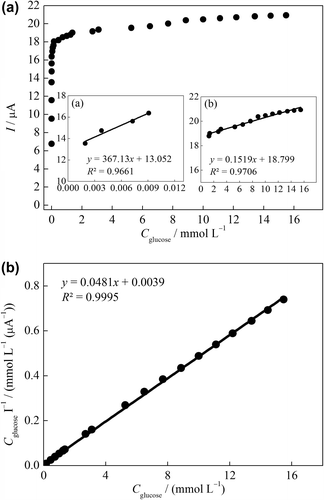
The limit of detection (LOD) is calculated to be 1.1 × 10–4 mmol L–1 (S/N = 3). LOD values confirmed the sensitivity of the GOD–NiONPs–CPE which is calculated using following equation:
where s is the standard deviation of the current (five runs) of the lowest concentration of the linearity range and m is the slope of the related calibration curve.
Km gives an indication of the enzyme–substrate kinetics for the glucose biosensor. Kinetic parameters such as Km and Imax were obtained from the Hanes–Woolf plot by plotting “substrate concentration/current response vs. substrate concentration” (). Km and Imax were calculated as 0.081 mM and 20.79 μA, respectively, using following equation (Garrett and Grisham Citation2009).
It is desired to obtain the highest imax and lowest Km for construction of enzyme electrodes (Wang Citation2006a). The Km in the present work is lower than GOD immobilized on NiO hollow nanospheres (7.76 mmol L–1) (Li et al. Citation2008), NiO-doped ZnO nanorods (7.4 mmol L–1) (Chu et al. Citation2012), and electrodeposited NiO nanoparticles (2.7 mmol L–1) (Salimi et al. Citation2007b).
Repeatability, reproducibility and storage stability
The repeatability of the enzyme electrode was evaluated using the sensitivities of same glucose biosensor for eight times consecutively at +0.4 V. The relative standard deviation of the obtained sensitivities was found as 6.58%. This result was the indication of good stability.
The reproducibility of the biosensor was also carried out by measuring the biosensor responses to sequential glucose increments from 0.02 to 0.20 mmol L–1 in the same day. Seven different biosensors, fabricated independently, had a relative standard deviation of 10.66%. These results indicate that the repeatability and reproducibility of the biosensor were highly satisfactory and obtained biosensor can be used in many analyses.
The long-term stability is one of the important features required for the satisfactory application of a biosensor. The stability of the glucose biosensor was performed by measuring the amperometric responses to sequential glucose increments from 0.02 to 0.20 mmol L–1. The long-term stability of the GOD–NiONPs–CPE was determined by performing activity assays within 20 days. The electrode was stored in dry atmosphere at +4°C when not in use. An activity loss of 10% was observed on the 20th day. The long-term stability of the GOD–NiONPs–CPE is much better compared to the stability of another carbon material electrode with a 26.5% loss of response after 22 days (Tang et al. Citation2004). Good long-term stability can be attributed to the mild immobilization procedure and a beneficial environment for preventing enzyme leakage. Immobilization of GOD in carbon paste matrix provides a biocompatible microenvironment around the enzyme. The developed biosensor achieved 95% of the steady-state current within about 5 s which is quite satisfactory for biosensor response. Such a fast response may reflect the increased electron transfer in the NiONPs-modified carbon paste.
Effect of interferences on the response of biosensor
The real sample contains other common species that could potentially interfere with the glucose detection. In the light of low concentrations of the ascorbic acid, dopamine, and uric acid in the blood, the interference needs to be seriously considered because they could be easily oxidized at a relatively high over potential, and a lower working potential helps to avoid the electrochemical reaction of the interferences.
The selectivity of proposed biosensor was performed in the presence of common interfering species such as ascorbic acid (AA, 0.1 mmol L–1), dopamine (DA, 0.1 mmol L–1), and uric acid (UA, 0.1 mmol L–1) in oxygenated PBS considering their physiological levels (). The percentages of interference were determined in terms of the difference in percentage of amperometric response between the buffer solution containing glucose (i0) and buffer solution containing interfering species and glucose (ix). Thus, the equation, (ix − i0)/i0 × 100 was used. The biosensor shows quick response for each addition of 0.1 mmol L‐1 glucose (G). However, as can be seen from no noteworthy response was observed for the addition of ascorbic acid, dopamine, and uric acid into buffer solution containing glucose at + 0.4 V (vs. Ag/AgCl). Although the ascorbic acid, dopamine, and uric acid caused a small increase in the current, these results can be overseen for the determination of glucose in serum samples due to maximum concentrations of L-ascorbic acid and uric acid in human body is about 0.1 and 0.05 mmol L–1, respectively (Xiao et al. Citation2000, CitationAydoğdu et al.). Thus, the developed biosensor is highly selective for glucose and can be applied for glucose determination in human serum samples under physiological conditions.
Figure 8. Amperometric responses of the glucose biosensor upon addition of AA (0.1 mmol L–1, DA (0.1 mmol L–1), and UA (0.1 mmol L–1) in 0.1 M PBS (pH 7.0) at 0.4 V vs. Ag/AgCl.
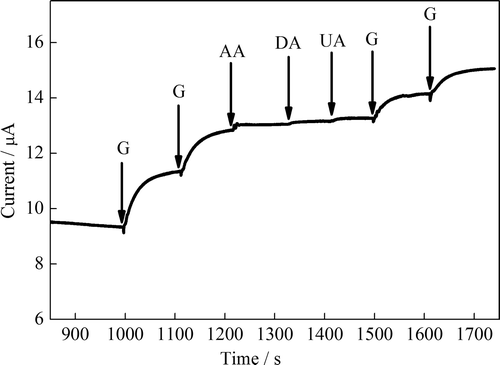
Table II. Effects of interferences on glucose response.
Determination of glucose in human serum
The determination of glucose in the serum sample was investigated on the GOD–NiONPs–CPE utilizing a standard addition method. Human serum samples were provided by a local hospital, which were first analyzed with Beckmann Coulter Olympus AU2700 analyzer which is based on hexokinase method. Then, serum samples of 10 μL were successively added into 5 mL phosphate buffered (0.1 mmol L–1 pH 7.0), and the response of the proposed biosensor was obtained at 0.4 V. The accuracy of glucose biosensor was controlled by t-test. The t value was found as 0.408 at 95% confidence level, for tcritic 4.30. As illustrated in , a good correlation between the two methods results allows us to ascertain the practical applicability of the developed biosensor.
Table III. The determination of glucose levels in human serum samples.
Conclusion
In the present study, a novel NiONPs-modified carbon paste enzyme electrode was developed for the determination of glucose. The NiONPs and GOD incorporated carbon paste electrode acted as a biosensor. The performance of CPE was dramatically improved due to the excellent electrocatalytic activity of NiONPs. The NiONPs-modified glucose biosensor showed good characteristics such as a wide determination range with a low detection limit (0.11 μmol L–1), high sensitivity, and stability. Furthermore, it has good reproducibility and applicability to human serum analysis and provides the determination of glucose at a low potential (+ 0.4 V). Kinetic parameters of the presented glucose biosensor Km and Imax were calculated as 0.081 mmol L–1 and 20.79 μA, respectively. The easy fabrication and excellent electrocatalytic capability of the prepared glucose biosensor can be expected to find practical applications to fabricate novel electrochemical biosensors.
Supplementary Figure 1 and 2
Download ()Declaration of interest
The authors report no declarations of interest. The authors alone are responsible for the content and writing of the paper
References
- Adekunle AS, Ozoemena KI. 2008. Electron transfer behaviour of single-walled carbon nanotubes electro-decorated with nickel and nickel oxide layers. Electrochim Acta. 53:5774–5782.
- Adekunle AS, Ozoemena KI. 2010. Electron transport and electrocatalytic properties of MWCNT/nickel nanocomposites: Hydrazine and diethylaminoethanethiol as analytical probes. J Electroanal Chem. 645:41–49.
- Aydoğdu G, Zeybek DK, Pekyardımcı Ş, Kılıç E. 2013. A novel amperometric biosensor based on ZnO nanoparticles-modified carbon paste electrode for determination of glucose in human serum. Artif Cells Nanomed Biotechnol. 41:332–338.
- Chu X, Zhu X, Dong Y, Chen T, Ye M, Sun W. 2012. An amperometric glucose biosensor based on the immobilization of glucose oxidase on the platinum electrode modified with NiO doped ZnO nanorods. J Electroanal Chem. 676:20–26.
- Çolak Ö, Yaşar A, Çete S, Arslan F. 2012. Glucose biosensor based on the immobilization of glucose oxidase on electrochemically synthesized polypyrrole-poly(vinyl sulphonate) composite film by cross-linking with glutaraldehyde. Artif Cells Blood Substit Biotechnol. 40:354–361.
- Ding Y, Liu Y, Zhang L, Wang Y, Bellagamba M, Parisi J, et al. 2011. Sensitive and selective nonenzymatic glucose detection using functional NiO–Pt hybrid nanofibers. Electrochim Acta. 58:209–214.
- Du D, Ding J, Cai J, Zhang A. 2007. Determination of carbaryl pesticide using amperometric acetylcholinesterase sensor formed by electrochemically deposited chitosan. Colloids Surf B Biointerfaces. 58:145–150.
- Forzani ES, Zhang H, Nagahara LA, Amlani I, Tsui R, Tao N. 2004. A conducting polymer nanojunction sensor for glucose detection. Nano Lett. 4:1785–1788.
- Garrett RH, Grisham CM. 2009. Biochemistry. Boston: Brooks/Cole Cengage Learning.
- Guo M, Fang H, Wang R, Yang Z, Xu X. 2011. Electrodeposition of chitosan-glucose oxidase biocomposite onto Pt–Pb nanoparticles modified stainless steel needle electrode for amperometric glucose biosensor. J Mater Sci Mater Med. 22:1985–1992.
- Hotovy I, Rehacek V, Siciliano P, Capone S, Spiess L. 2002. Sensing characteristics of NiO thin films as NO2 gas sensor. Thin Solid Films. 418:9–15.
- Jiang L, Liu H, Liu J, Yang Q, Cai X. 2008. A sensitive biosensor based on Os-complex mediator and glucose oxidase for low concentration glucose determination. J Electroanal Chem. 619–620:11–16.
- Lata S, Batra B, Karwasra N, Pundir CS. 2012. An amperometric H2O2 biosensor based on cytochrome c immobilized onto nickel oxide nanoparticles/carboxylated multiwalled carbon nanotubes/polyaniline modified gold electrode. Process Biochem. 47:992–998.
- Li C, Liu Y, Li L, Du Z, Xu S, Zhang M, et al. 2008. A novel amperometric biosensor based on NiO hollow nanospheres for biosensing glucose. Talanta. 77:455–459.
- Li F, Li J, Feng Y, Yang L, Du Z. 2011. Electrochemical behavior of graphene doped carbon paste electrode and its application for sensitive determination of ascorbic acid. Sens Actuators B Chem. 157:110–114.
- Li J, Lin X. 2007. Glucose biosensor based on immobilization of glucose oxidase in poly(o-aminophenol) film on polypyrrole-Pt nanocomposite modified glassy carbon electrode. Biosens Bioelectron. 22:2898–2905.
- Liu Y, Yuan R, Chai Y, Tang D, Dai J, Zhong X. 2006. Direct electrochemistry of horseradish peroxidase immobilized on gold colloid/cysteine/nafion-modified platinum disk electrode. Sens Actuators B Chem. 115:109–115.
- Mercado RC, Moussy F. 1998. In vitro and in vivo mineralization of Nafion membrane used for implantable glucose sensors. Biosens Bioelectron. 13:133–145.
- Moussy F, Jakeway S, Harrison DJ, Rajotte RV. 1994. In vitro and in vivo performance and lifetime of perfluorinated ionomer-coated glucose sensors after high-temperature curing. Anal Chem. 66: 3882–3888.
- Mu Y, Jia D, He Y, Miao Y, Wu HL. 2011. Nano nickel oxide modified non-enzymatic glucose sensors with enhanced sensitivity through an electrochemical process strategy at high potential. Biosens Bioelectron. 26:2948–2952.
- Noorbakhsh A, Salimi A. 2011. Development of DNA electrochemical biosensor based on immobilization of ssDNA on the surface of nickel oxide nanoparticles modified glassy carbon electrode. Biosens Bioelectron. 30:188–196.
- Pan D, Chen J, Nie L, Tao W, Yao S. 2004. Amperometric glucose biosensor based on immobilization of glucose oxidase in electropolymerized o-aminophenol film at Prussian blue-modified platinum electrode. Electrochimica Acta. 49:795–801.
- Privett BJ, Shin JH, Schoenfisch MH. 2008. Electrochemical sensors. Anal Chem. 80:4499–4517.
- Salimi A, Sharifi E, Noorbakhsh A, Soltanian S. 2007a. Direct electrochemistry and electrocatalytic activity of catalase immobilized onto electrodeposited nano-scale islands of nickel oxide. Biophys Chem. 125:540–548.
- Salimi A, Sharifi E, Noorbakhsh A, Soltanian S. 2007b. Immobilization of glucose oxidase on electrodeposited nickel oxide nanoparticles: direct electron transfer and electrocatalytic activity. Biosens Bioelectron. 22:3146–3153.
- Song M-J, Kim J, Lee S, Lee J-H, Lim D, Hwang S, Whang D. 2010. Pt-polyaniline nanocomposite on boron-doped diamond electrode for amperometic biosensor with low detection limit. Microchim Acta. 171:249–255.
- Tang H, Chen J, Yao S, Nie L, Deng G, Kuang Y. 2004. Amperometric glucose biosensor based on adsorption of glucose oxidase at platinum nanoparticle-modified carbon nanotube electrode. Anal Biochem. 331:89–97.
- Usman Ali SM, Nur O, Willander M, Danielsson B. 2010. A fast and sensitive potentiometric glucose microsensor based on glucose oxidase coated ZnO nanowires grown on a thin silver wire. Sens Actuators B Chem. 145:869–874.
- Vaddiraju S, Legassey A, Wang Y, Qiang L, Burgess DJ, Jain F, Papadimitrakopoulos F. 2011. Design and fabrication of a high-performance electrochemical glucose sensor. J Diabetes Sci Technol, 5:1044–51.
- Wang J. 2006a. Electrochemical Sensors. Analytical Electrochemistry. New York: John Wiley & Sons, Inc.
- Wang J. 2006b. Study of Electrode Reactions and Interfacial Properties. Analytical Electrochemistry. New York: John Wiley & Sons, Inc.
- Wang XD, Chen HX, Zhou TY, Lin ZJ, Zeng JB, Xie ZX, et al. 2009. Optical colorimetric sensor strip for direct readout glucose measurement. Biosens Bioelectron. 24:3702–3705.
- Wang X, Zhang Y, Cheng C, Dong R, Hao J. 2011. Glucose in human serum determined by capillary electrophoresis with glucose micro-biosensor. Analyst. 136:1753–1759.
- Xiao L, Chen J, Cha CS. 2000. Elimination of the interference of ascorbic acid in the amperometric detection of biomolecules in body fluid samples and the simple detection of uric acid in human serum and urine by using the powder microelectrode technique. J Electroanal Chem. 495:27–35.
- Xu JJ, Luo XL, Du Y, Chen HY. 2004. Application of MnO2 nanoparticles as an eliminator of ascorbate interference to amperometric glucose biosensors. Electrochem Commun. 6:1169–1173.
- Yang L, Ren X, Tang F, Zhang L. 2009. A practical glucose biosensor based on Fe3O4 nanoparticles and chitosan/nafion composite film. Biosens Bioelectron. 25:889–895.
- Yao Y, Shiu KK. 2007. Electron-transfer properties of different carbon nanotube materials, and their use in glucose biosensors. Anal Bioanal Chem. 387:303–309.
- Zargoosh K, Chaichi MJ, Shamsipur M, Hossienkhani S, Asghari S, Qandalee M. 2012. Highly sensitive glucose biosensor based on the effective immobilization of glucose oxidase/carbon-nanotube and gold nanoparticle in nafion film and peroxyoxalate chemiluminescence reaction of a new fluorophore. Talanta. 93:37–43.
- Zhang Y, Gui Y, Wu X, Feng H, Zhang A, Wang L, Xia T. 2009. Preparation of nanostructures NiO and their electrochemical capacitive behaviors. Int J Hydrogen Energy. 34:2467–2470.
- Zheng YZ, Zhang ML. 2007. Preparation and electrochemical properties of nickel oxide by molten-salt synthesis. Mater Lett. 61:3967–3969.
- Zhu L, Li Y, Tian F, Xu B, Zhu G. 2002. Electrochemiluminescent determination of glucose with a sol–gel derived ceramic–carbon composite electrode as a renewable optical fiber biosensor. Sens Actuators B Chem. 84:265–270.
- Zong SZ, Cui RJ, Fei L, Li WW, Ju HX. 2010. Immobilization of myoglobin on NiO nanoparticles matrix for preparation of novel biosensor. Chinese J Anal Chem. 38:1533–1537.