Abstract
For successful gene therapy, expansion of appropriate gene delivery systems could be one of the factors of major significance. Gene therapy provides large opportunities for treating diseases, including genetic disorders, infections, and cancer. Polymeric carriers have relatively low cytotoxicity and immunogenicity. Polymeric gene carriers are a potential substitute to using viral vectors. Overall, polymeric carriers can contain large-sized DNA, be conjugated with suitable functionalities, and be administered frequently. However, polymeric gene carriers have some restrictions, such as low gene transfection efficiencies and a moderately short period of gene expression.
This study explores the current status of development of polymeric gene carriers, and presents guidelines for the prospective use of the polymer-based gene delivery systems in gene therapy.
Introduction
Gene therapy has gathered a lot of attention in the fields of medicine, pharmacology, and biotechnology, due to its potential to treat chronic diseases and genetic disorders (CitationPark et al. 2006, CitationLedley 1996), and its ability to facilitate localized delivery to target tissues, strongly improving the efficacy of various therapies. In recent years, significant effort has been made in the area of nanotechnology for drug delivery, since it offers a suitable means of delivering small molecular drugs, such as proteins, peptides or genes to the organ or tissue of interest, by either localized or targeted delivery (CitationBengali et al. 2005, CitationYang et al. 2001). Nanocarriers have provided a novel stage for target-specific delivery of therapeutic agents. Several delivery tools have been designed based on different nanoparticles, such as polymers (CitationLai et al. 2009), dendrimers (CitationEnsign et al. 2012), liposomes (CitationKnowles et al. 1995), and gold nanoparticles (CitationGhosh et al. 2008).
Gene delivery systems should be organized to protect the genetic materials from premature degradation in the systemic blood stream, and to effectively transfer the therapeutic genes to target cells (CitationPark et al. 2006, CitationHan et al. 2000).
Nanoparticle systems can be engineered to have a number of attractive qualities for therapy, including: (i) sustained and controlled release of drugs locally (CitationKrebs et al. 2010, CitationKievit et al. 2009), (ii) deep tissue penetration due to their nanoscale size (CitationPark et al. 2006, CitationPissuwan et al. 2011), (iii) cellular uptake and sub-cellular trafficking (CitationMalafaya et al. 2007, CitationJeong et al. 2007), and (iv) protection of cargo therapeutics at both the extracellular and intracellular levels (CitationPark et al. 2006, CitationJeong et al. 2007, CitationPanyam and Labhasetwar 2003).
The size of nanoparticles (NPs) ranges from 10 to 200 nm, which is the same as the size of protein molecules. The small size offers the potential of targeted gene delivery, allowing the deep penetration of tissues at the proper site, like solid tumors, with a high level of specificity (CitationJin et al. 2009).
With the small size, NPs can easily interact with biomolecules on the cell surface or inside cells, and deliver genetic materials such as DNA, RNA, or small interfering RNA (siRNA) into target cells or tissues for gene expression (CitationKaul and Amiji 2005, CitationKaul 2005, CitationKneuer et al. 2000a, Citation2000b).
This review will discuss the existing status of use of natural polymers, dendrimers, liposomes, and gold nanoparticles (AuNPs) for gene delivery. Gene therapy involves intracellular transfer of nucleic acid drugs to adjust cellular functions and responses by expressing exogenous proteins, by inhibiting instruction of a specific gene, or by controlling undesirable genomic mutations.
Polymers in gene delivery
Biomaterial sciences have already explained that polymers can be considered to have a very biocompatible, nontoxic form, with no immunogenicity, which in theory enables repeated administration and continuing therapeutic effects (CitationSchaffert and Wagner 2008).
Natural polymers have been used for a wide range of gene therapies, from NPs to three-dimensional scaffolds. These particles have been used successfully for oral and intramuscular delivery as non-viral gene therapy systems (CitationDang and Leong 2006, CitationCaplan 2005). Polymeric NPs are used in adenosine deaminase enzyme deficiency (ADA) and acute lymphoblastic leukemia (CitationAnajwala et al. 2010).
In condensing the anionic DNA into dense nanoparticles, the polymeric gene carrier can provide protection from DNAses and lengthen the bioavailability of the integrated DNA. In addition, polymers with available functional groups can be tailored with surface ligands to realize targeted gene delivery via receptor-mediated endocytosis (CitationJunghans et al. 2000, CitationAbdallah et al. 1996, CitationBurke and Pun 2008).
A certain advantage of polymer systems is the abundance of potential chemical modifications, such as the synthesis of block and comb polymers (CitationMehnert and Mäder 2001, CitationMüller et al. 2000).
The communication between the positively charged polymer backbone and negatively charged DNA leads to the spontaneous formation of nano-sized complexes (polyplexes) (CitationLee and Kim 2005).The structure of the polyplex core effectively prevents the entrance of nucleases into the enclosed nucleic acid drugs. They should maintain their solidity before cellular uptake by endocytosis (CitationJeong et al. 2007, CitationAbdallah et al. 1996, CitationGlover et al. 2005) ().
Figure 1. (a) The formation of polyplexes by ionic interaction between negatively charged DNA and polycations (b) major barriers that polyplexes should overcome for efficient gene delivery Extracellular barriers include the maintenance of stability of the polyplexes during systemic circulation, and the transport from blood vessels to the tissue. Intracellular barriers for delivery include (i) cellular uptake via endocytosis, (ii) endosomal escape, (iii) transport to the perinuclear space, (iv) unpacking of the polyplexes, and (v) nuclear translocation (Molecular Design of Functional Polymers for Gene Therapy, 2007).
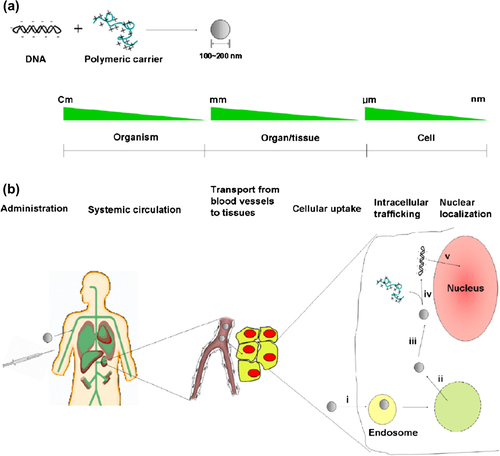
Polymeric non-viral carriers consist of: polyethylenimine (PEI), poly(L-lysine) (PLL), poly(2-(dimethylamino)ethyl methacrylate (pDMAEMA), dendrimers, biodegradable polymers, natural polymers, and non-ionic neutral polymers (CitationBrem and Gabikian 2001, CitationChoi et al. 1999).
PEI has been commonly used in paper industries, water purification, and food industries. The first time PEI was used in gene therapy was in 1995, and PEI has become one of the gold standards of non-viral gene delivery (CitationEliyahu et al. 2005, CitationWivel and Wilson 1998, CitationRosenberg et al. 1990). PEI is an angiogenic, endothelial cell-targeted gene delivery system (CitationPark et al. 2006).
Not only does the molecular weight of the PEI have advantages, the degree of branching also plays a significant role in the biological properties of complexes with nucleic acids (CitationDang and Leong 2006, CitationEliyahu et al. 2005, CitationMelo et al. 2004, CitationPartridge and Oreffo 2004). Branched PEI molecules have primary, secondary, and tertiary amino groups, while linear PEI molecules mostly have secondary amines. On the other hand, studies with linear PEIs showed constantly higher transfection efficiency and lower cytotoxicity compared to branched PEI (CitationPark et al. 2006, CitationFerrari et al. 1997, CitationColl et al. 1999, CitationWightman et al. 2001).
The PEI-grafted PEG (PEI-g-PEG) copolymer has been shown to lessen PEI's toxicity with special PEG grafting ratios, by providing a physical barrier between the cell and PEI (CitationKievit et al. 2009, CitationPetersen et al. 2002, CitationSuh et al. 2002).
The PEI-g-PEG-RGD/DNA complexes showed much lower cytotoxicity and about five times higher transfection competence in VEGF-stimulated angiogenic human dermal microvascular endothelial cells (HDMEC) than the PEI/DNA complexes.
In addition, PEI, one of the most commonly used polymeric gene carriers, can increase the transfection competence by evading DNA complexes from degradative endosome or lysosome compartments (CitationSchaffert and Wagner 2008, CitationKasyanenko et al. 2012, CitationGyawali et al. 2012, CitationKim et al. 2005).
PLL is used in nonviral gene delivery, and has been utilized in physicochemical and biological experiments (CitationWolfert et al. 1999, CitationMerdan et al. 2002). PLL, having a molecular weight of less than 3000, cannot shape firm complexes with DNA, suggesting that the number of primary amines in the PLL backbone is significant for the complex pattern. PLL is biodegradable, and this property exhibits modest to high toxicity (CitationPhillips 1995, CitationFajac et al. 2000).
The PLL/DNA complexes show a reasonably high cytotoxicity and are poor gene delivery vectors (CitationMalafaya et al. 2007, CitationXiao et al. 2003), having an affinity to combine and precipitate, depending on the ionic potency (CitationLucas et al. 1999). For example, addition of chloroquine for constant reasonable transfection activity (CitationXiao et al. 2003).
On the other hand, the properties of the complex surface may be the cause for cytotoxicity. Moreover, the charged surface can enable the nonspecific adsorption of serum proteins, resulting in rapid clearance of the complexes from the blood stream (CitationOgris et al. 1999). Therefore, to solve this problem, PEG was attached, to prevent the interaction and to increase complex stability in the presence of serum protein (CitationLee and Kim 2005, CitationHarada-Shiba et al. 2002).
Dendrimers
The word ‘dendrimer’ originates from two Greek words, dendra: tree, and meros: part. Dendrimers are characterized by their distinct architectural feature, in which several hyper-branched arms extend from a central core to the surface, in a controlled manner (CitationHarada-Shiba et al. 2002).
Dendrimers of lower generations (0, 1, and 2) have extremely asymmetric shapes and have more open structures as compared to higher generation dendrimers (CitationKlajnert and Bryszewska 2000). Dendrimers become closely packed as they pull out to the periphery, which forms a closed membrane-like structure. When a critical branched state is reached, dendrimers cannot grow because of a lack of space (CitationYin and Alivisatos 2004) ().
Figure 2. Electrophoresis for DNA concentration with polymer (DNA–Polymer Complexes for Gene Therapy, 2012).
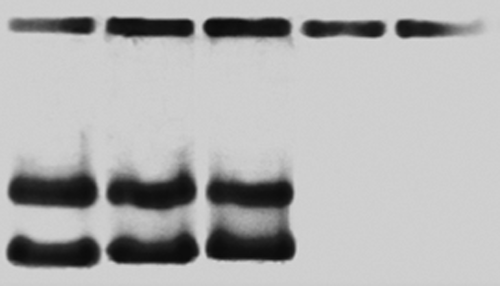
Dendrimers are monodisperse macromolecules, unlike linear polymers. The solubility of dendrimers is powerfully influenced by the nature of surface groups. Dendrimers ending in hydrophilic groups are soluble in polar solvents, while dendrimers having hydrophobic end groups are soluble in nonpolar solvents (CitationMourey et al. 1992).
Dendrimers consist of three main parts, namely, the surface (functional peripheral group), interior (affects host-guest properties), and core (affects the 3D shape of the dendrimer) (CitationMa et al. 2002).
Dendrimers have been used in in vitro diagnostics. There are more than fifty families of dendrimers. They have been tested in preclinical studies, such as magnetic resonance imaging (MRI) (CitationFischer and Vögtle 1999).
There have been attempts to use dendrimers in the targeted delivery of drugs and other therapeutic agents (CitationHawker and Frechet 1992).
The biological properties of dendrimers are important because of the increasing attention in using them in biomedical applications (CitationFrechet 1994).
Liposomes
Liposomes were discovered by Bangham et al. in the 1960s, and were introduced as drug delivery vehicles in the 1970s (CitationBangham and Horne 1964, CitationWissing et al. 2004, CitationEldem et al. 1991). They are spherical vesicles composed of one or more phospholipid bilayers, in most cases phosphatidylcholine. The main advantage of this carrier system is the reduction of side effects caused at the injection site (CitationSchmidt 1997).
Particulate drug carriers investigated for many years include oil-in-water (O/W) emulsions, liposomes, microparticles and nanoparticles (CitationMüller et al. 2000, Citationzur Mühlen et al. 1998). The O/W emulsions have been introduced effectively in clinical practice for parenteral nutrition. Anchored in these emulsions for parenteral nutrition, drug-containing emulsion structures have been developed, e.g. containing diazepam and etomidate (CitationSchwarz et al. 1994).
It was the anti-aging product Capture (Dior) which paved the way for liposome-based pharmaceutical products. The use of solid lipids as a matrix material for drug delivery is well-known, from the use of lipid pellets for oral drug delivery (CitationKakkar et al. 2011).
The nanoparticles are in the submicron size range (50–1000 nm), and they are composed of physiological lipids. At room temperature, the particles are in the solid state. Therefore, the mobility of entrapped drugs is reduced, which is a requirement for controlled drug release (CitationAkiyoshi et al. 1998).
They are stabilized with non-toxic surfactants like poloxamer and lecithin (Citationzur Mühlen et al. 1998, CitationGasco 1993). Rupenagunta et al., in 2011, showed that the advantages of solid lipid nanoparticles (SLNs) are the use of physiological lipids, decrease in the the danger of acute and chronic toxicity, and avoidance of organic solvents in production. Fahr and Liu, in 2007, demonstrated the improved bioavailability of poorly water soluble molecules, protection of both chemically labile agents from degradation in the gut and sensitive molecules from the outer environment, thereby achieving a high concentration of efficient compound (CitationFahr and Liu 2007).
The avoidance of organic solvents, a potential extensive application range (dermal, intravenous), and the high pressure homogenization as a recognized production method, allow large-scale production. Moreover, improved bioavailability, and protection of sensitive drug molecules from the outer environment (water, light), are added advantages (CitationJores et al. 2005).
To date, numerous studies have been conducted regarding the optimization of production parameters (CitationSmith 1986), long term stability (CitationMüller et al. 1996), recrystallization behaviour (CitationWestesen and Siekmann 1997), morphological characterization (CitationAlmeida and Souto 2007), and in vivo toxicity (CitationWissing et al. 2004).
Solid lipid nanoparticles (SLNs) were introduced in 1991, as a novel drug delivery system for pharmaceutical drugs in a variety of application routes, and as alternative carriers (CitationMehnert and Mäder 2001). At the turn of the millennium, modifications of SLNs, the so-called nanostructured lipid carriers (NLC) and the lipid drug conjugate (LDC) nanoparticles, have been introduced in the literature (Citationzur Mühlen et al. 1998). The disadvantages of SLNs include their poor drug loading capacity.
Gold nanoparticles
AuNPs are particularly interesting because biologically, they should not be cytotoxic (CitationDaniel and Astruc 2004).
The fact that the surface of AuNPs suggests well established routes for functionality makes them particularly favored vehicles. Charged or hydrophobic motifs can be bound to the surface, and in fact can be combined with modification protocols that include targeting moieties such as antibodies and receptors. These too can be mixed with a payload such as double-stranded DNA (dsDNA) or single-stranded DNA (ssDNA), which is then transfected into cells (CitationSandhu et al. 2002, CitationRosi et al. 2006).
AuNPs have a high affinity for biomolecules and have been used as nonviral vectors for DNA delivery (CitationMelo et al. 2004, CitationThomas and Klibanov 2003). AuNPs chemically functionalized with alkylthiol-terminated oligonucleotides (CitationMirkin et al. 1996) are highly stable in saline solutions, and bind complementary nucleic acids in a very selective and cooperative manner (CitationJin et al. 2003); their equilibrium association constants can be more than two orders of magnitude greater than those observed for unmodified oligonucleotides and their complements (CitationRosi et al. 2006).
Many studies have shown that AuNPs have played a role as drug and vaccine carriers into target cells or specific tissues. Generally, this has been achieved by modifying the surface of the AuNPs, so that they can bind to the specific targeting drugs or other biomolecules. The delivery of drugs with nanoparticles can result in higher concentrations than possible with normal drug delivery schemes (CitationChen et al. 2008).
AuNPs are also attractive because of their unique properties, which we have described previously. For example, in 2001, there was an investigation of AuNPs that had been functionalized with cationic quaternary ammonium groups and then electrostatically bound to plasmid DNA. This composite particle could protect the DNA from enzymatic degradation and could regulate DNA transcription of T7 RNA polymerase (CitationMcIntosh et al. 2001, CitationHan et al. 2006) ().
Conclusion
Biomaterial sciences have explained that polymeric systems can be safely used in a very nontoxic, biocompatible, and non-immunogenic form, which in theory enables continual administration and continuing therapeutic effects. Nanocarriers and biomaterials have provided a narrative stage for target-specific delivery of therapeutic agents.
Authors’ contributions
AA and TK conceived of the study and participated in its design and coordination. EA participated in the sequence alignment and drafted the manuscript. All authors read and approved the final manuscript.
Acknowledgments
The authors thank the Department of Medical Nanotechnology, Faculty of Advanced Medical Science, Tabriz University, for all support provided. This work is funded by the Grant 2011-0014246 of the National Research Foundation of Korea. This work is also funded by the 2014 Drug Applied Research Center Tabriz University of Medical Sciences Grant.
Declaration of interest
The authors report no declarations of interest. The authors alone are responsible for the content and writing of the paper.
References
- Abdallah B, Hassan A, Benoist C, Goula D, Behr JP, Demeneix BA. 1996. A powerful nonviral vector for in vivo gene transfer into the adult mammalian brain: polyethylenimine. Hum Gene Ther. 7:1947–1954.
- Akiyoshi K, Kobayashi S, Shichibe S, Mix D, Baudys M, Kim SW, Sunamoto J. 1998. Self-assembled hydrogel nanoparticle of cholesterol-bearing pullulan as a carrier of protein drugs: complexation and stabilization of insulin. J Control Release. 54:313–320.
- Almeida AJ, Souto E. 2007. Solid lipid nanoparticles as a drug delivery system for peptides and proteins. Adv Drug Deliv Rev. 59:478–490.
- Anajwala CC, Jani GK, Swamy SV. 2010. Current trends of nanotechnology for cancer therapy. Int J Pharm Sci Nanotechnol. 3: 1043–1056.
- Bangham A, Horne R. 1964. Negative staining of phospholipids and their structural modification by surface-active agents as observed in the electron microscope. J Mol Biol. 8:660–IN10.
- Bengali Z, Pannier AK, Segura T, Anderson BC, Jang JH, Mustoe TA, Shea LD. 2005. Gene delivery through cell culture substrate adsorbed DNA complexes. Biotechnol Bioeng. 90:290–302.
- Brem H, Gabikian P. 2001. Biodegradable polymer implants to treat brain tumors. J Control Release. 74:63–67.
- Burke RS, Pun SH. 2008. Extracellular barriers to in vivo PEI and PEGylated PEI polyplex-mediated gene delivery to the liver. Bioconjug Chem. 19:693–704.
- Caplan AI. 2005. Review: mesenchymal stem cells: cell-based reconstructive therapy in orthopedics. Tissue Eng. 11:1198–1211.
- Chen PC, Mwakwari SC, Oyelere AK. 2008. Gold nanoparticles: from nanomedicine to nanosensing. Nanotechnol Sci Appl. 1:45.
- Choi YH, Liu F, Choi JS, Kim SW, Park JS. 1999. Characterization of a targeted gene carrier, lactose-polyethylene glycol-grafted poly-L-lysine, and its complex with plasmid DNA. Hum Gene Ther. 10:2657–2665.
- Coll JL, Chollet P, Brambilla E, Desplanques D, Behr JP, Favrot M. 1999. In vivo delivery to tumors of DNA complexed with linear polyethylenimine. Hum Gene Ther. 10:1659–1666.
- Dang JM, Leong KW. 2006. Natural polymers for gene delivery and tissue engineering. Adv Drug Deliv Rev. 58:487–499.
- Daniel M-C, Astruc D. 2004. Gold nanoparticles: assembly, supramolecular chemistry, quantum-size-related properties, and applications toward biology, catalysis, and nanotechnology. Chem Rev. 104:293–346.
- Eldem T, Speiser P, Hincal A. 1991. Optimization of spray-dried and-congealed lipid micropellets and characterization of their surface morphology by scanning electron microscopy. Pharm Res. 8:47–54.
- Eliyahu H, Barenholz Y, Domb A. 2005. Polymers for DNA delivery. Molecules. 10:34–64.
- Ensign LM, Cone R, Hanes J. 2012. Oral drug delivery with polymeric nanoparticles: the gastrointestinal mucus barriers. Adv Drug Deliv Rev. 64:557–570.
- Fahr A, Liu X. 2007. Drug delivery strategies for poorly water-soluble drugs. Expert Opin Drug Deliv. 4:403–416.
- Fajac I, Allo JC, Souil E, Merten M, Pichon C, Figarella C, et al. 2000. Histidylated polylysine as a synthetic vector for gene transfer into immortalized cystic fibrosis airway surface and airway gland serous cells. J Gene Med. 2:368–378.
- Ferrari S, Moro E, Pettenazzo A, Behr JP, Zacchello F, Scarpa M. 1997. ExGen 500 is an efficient vector for gene delivery to lung epithelial cells in vitro and in vivo. Gene Ther. 4:1100–1106.
- Fischer M, Vögtle F. 1999. Dendrimers: from design to application—a progress report. Angew Chem Int Ed. 38:884–905.
- Frechet JM. 1994. Functional polymers and dendrimers: reactivity, molecular architecture, and interfacial energy. Science. 263: 1710–1715.
- Gasco MR. 1993. Method for producing solid lipid microspheres having a narrow size distribution. Google Patents.
- Ghosh P, Han G, De M, Kim CK, Rotello VM. 2008. Gold nanoparticles in delivery applications. Adv Drug Deliv Rev. 60:1307–1315.
- Glover DJ, Lipps HJ, Jans DA. 2005. Towards safe, non-viral therapeutic gene expression in humans. Nat Rev Genet. 6:299–310.
- Gyawali D, Palmer M, Tran RT, Yang I. 2012. for Theranostic Systems. Biomedical Materials and Diagnostic Devices, p. 435.
- Han G, Martin CT, Rotello VM. 2006. Stability of gold nanoparticle‐bound DNA toward biological, physical, and chemical agents. Chem Biol Drug Des. 67:78–82.
- Han S-o, Mahato RI, Sung YK, Kim SW. 2000. Development of biomaterials for gene therapy. Mol Ther. 2:302–317.
- Harada-Shiba M, Yamauchi K, Harada A, Takamisawa I, Shimokado K, Kataoka K. 2002. Polyion complex micelles as vectors in gene therapy–pharmacokinetics and in vivo gene transfer. Gene Ther. 9:407–414.
- Hawker CJ, Frechet JM. 1992. Unusual macromolecular architectures: The convergent growth approach to dendritic polyesters and novel block copolymers. J Am Chem Soc. 114:8405–8413.
- Jeong JH, Kim SW, Park TG. 2007. Molecular design of functional polymers for gene therapy. Prog Polym Sci. 32:1239–1274.
- Jin R, Wu G, Li Z, Mirkin CA, Schatz GC. 2003. What controls the melting properties of DNA-linked gold nanoparticle assemblies? J Am Chem Soc. 125:1643–1654.
- Jin S, Leach JC, Ye K. 2009. Nanoparticle-mediated gene delivery In: Lee IW, Foote RS, Eds. Micro and Nano Technologies in Bioanalysis. Berlin: Springer, pp. 547–557.
- Jores K, Haberland A, Wartewig S, Mäder K, Mehnert W. 2005. Solid lipid nanoparticles (SLN) and oil-loaded SLN studied by spectrofluorometry and Raman spectroscopy. Pharm Res. 22: 1887–1897.
- Junghans M, Kreuter J, Zimmer A. 2000. Antisense delivery using protamine–oligonucleotide particles. Nucleic Acids Res. 28: e45–e45.
- Kakkar V, Singh S, Singla D, Kaur IP. 2011. Exploring solid lipid nanoparticles to enhance the oral bioavailability of curcumin. Mol Nutr Food Res. 55:495–503.
- Kasyanenko N, Lysyakova LA, Dribinskii BA, Zolotova YuI, Nazarova OV, Panarin EF. 2012. DNA-polymer complexes for gene therapy. Polym Sci Ser C. 54:57–68.
- Kaul G, Amiji M. 2005. Cellular interactions and in vitro DNA transfection studies with poly (ethylene glycol)‐modified gelatin nanoparticles. J Pharm Sci. 94:184–198.
- Kaul G. 2005. Amiji M. Tumor-targeted gene delivery using poly (ethylene glycol)-modified gelatin nanoparticles: in vitro and in vivo studies. Pharm Res. 22:951–961.
- Kievit FM, Veiseh O, Bhattarai N, Fang C, Gunn JW, Lee D, et al. 2009. PEI–PEG–chitosan‐copolymer‐coated iron oxide nanoparticles for safe gene delivery: synthesis, complexation, and transfection. Adv Funct Mater. 19:2244–2251.
- Kim WJ, Yockman JW, Lee M, Jeong JH, Kim YH, Kim SW. 2005. Soluble Flt-1 gene delivery using PEI- g-PEG-RGD conjugate for anti-angiogenesis. J Control Release. 106:224–234.
- Klajnert B. Bryszewska M. 2000. Dendrimers: properties and applications. Acta Biochim Polonica. 48:199–208.
- Kneuer C, Sameti M, Bakowsky U, Schiestel T, Schirra H, Schmidt H, Lehr CM. 2000a. A nonviral DNA delivery system based on surface modified silica-nanoparticles can efficiently transfect cells in vitro. Bioconjug Chem. 11:926–932.
- Kneuer C, Sameti M, Haltner EG, Schiestel T, Schirra H, Schmidt H, Lehr CM. 2000b. Silica nanoparticles modified with aminosilanes as carriers for plasmid DNA. Int J Pharm. 196:257–261.
- Knowles MR, Hohneker KW, Zhou Z, Olsen JC, Noah TL, Hu PC, et al. 1995. A controlled study of adenoviral-vector–mediated gene transfer in the nasal epithelium of patients with cystic fibrosis. N Engl J Med. 333:823–831.
- Krebs MD, Salter E, Chen E, Sutter KA, Alsberg E. 2010. Calcium phosphate‐DNA nanoparticle gene delivery from alginate hydrogels induces in vivo osteogenesis. J Biomed Mater Res Part A. 92:1131–1138.
- Lai SK, Wang Y-Y, Hanes J. 2009. Mucus-penetrating nanoparticles for drug and gene delivery to mucosal tissues. Adv Drug Deliv Rev. 61:158–171.
- Ledley FD. 1996. Pharmaceutical approach to somatic gene therapy. Pharm Res. 13:1595–1614.
- Lee M, Kim SW. 2005. Polyethylene glycol-conjugated copolymers for plasmid DNA delivery. Pharm Res. 22:1–10.
- Lucas P, Milroy DA, Thomas BJ, Moss SH, Pouton CW. 1999. Pharmaceutical and biological properties of poly (amino acid)/DNA polyplexes. J Drug Target. 7:143–156.
- Ma Y, Kolotuchin SV, Zimmerman SC. 2002. Supramolecular Polymer Chemistry: Self-Assembling Dendrimers Using the DDA⊙ AAD (GC-like) Hydrogen Bonding Motif. J Am Chem Soc. 124: 13757–13769.
- Malafaya PB, Silva GA, Reis RL. 2007. Natural–origin polymers as carriers and scaffolds for biomolecules and cell delivery in tissue engineering applications. Adv Drug Deliv Rev. 59:207–233.
- McIntosh CM, Esposito EA III3rd, Boal AK, Simard JM, Martin CT, Rotello VM. 2001. Inhibition of DNA transcription using cationic mixed monolayer protected gold clusters. J Am Chem Soc. 123: 7626–7629.
- Mehnert W, Mäder K. 2001. Solid lipid nanoparticles: production, characterization and applications. Adv Drug Deliv Rev. 47: 165–196.
- Melo LG, Pachori AS, Kong D, Gnecchi M, Wang K, Pratt RE, Dzau VJ. 2004. Gene and cell-based therapies for heart disease. FASEB J. 18:648–663.
- Merdan T, Kunath K, Fischer D, Kopecek J, Kissel T. 2002. Intracellular processing of poly (ethylene imine)/ribozyme complexes can be observed in living cells by using confocal laser scanning microscopy and inhibitor experiments. Pharm Res. 19:140–146.
- Mirkin CA, Letsinger RL, Mucic RC, Storhoff JJ. 1996. A DNA-based method for rationally assembling nanoparticles into macroscopic materials. Nature. 382:607–609.
- Mourey TH, Turner SR, Rubinstein M, Frechet JMJ, Hawker CJ, Wooley KL. 1992. Unique behavior of dendritic macromolecules: intrinsic viscosity of polyether dendrimers. Macromolecules. 25:2401–2406.
- Müller R, Maaen S, Weyhers H, Specht F, Lucks JS. 1996. Cytotoxicity of magnetite-loaded polylactide, polylactide/glycolide particles and solid lipid nanoparticles. Int J Pharm. 138:85–94.
- Müller RH, Mäder K, Gohla S. 2000. Solid lipid nanoparticles (SLN) for controlled drug delivery–a review of the state of the art. Eur J Pharm Biopharm. 50:161–177.
- Ogris M, Brunner S, Schüller S, Kircheis R, Wagner E. 1999. PEGylated DNA/transferrin-PEI complexes: reduced interaction with blood components, extended circulation in blood and potential for systemic gene delivery. Gene Ther. 6:595–605.
- Panyam J, Labhasetwar V. 2003. Biodegradable nanoparticles for drug and gene delivery to cells and tissue. Adv Drug Deliv Rev. 55:329–347.
- Park TG, Jeong JH, Kim SW. 2006. Current status of polymeric gene delivery systems. Adv Drug Deliv Rev. 58:467–486.
- Partridge KA, Oreffo RO. 2004. Gene delivery in bone tissue engineering: progress and prospects using viral and nonviral strategies. Tissue Eng. 10:295–307.
- Petersen H, Fechner PM, Martin AL, Kunath K, Stolnik S, Roberts CJ. 2002. Polyethylenimine-graft-poly (ethylene glycol) copolymers: influence of copolymer block structure on DNA complexation and biological activities as gene delivery system. Bioconjug Chem. 13:845–854.
- Phillips SC. 1995. Receptor-mediated DNA delivery approaches to human gene therapy. Biologicals. 23:13–16.
- Pissuwan D, Niidome T, Cortie MB. 2011. The forthcoming applications of gold nanoparticles in drug and gene delivery systems. J Control Release. 149:65–71.
- Rosenberg SA, Aebersold P, Cornetta K, Kasid A, Morgan RA, Moen R, et al. 1990. Gene transfer into humans—immunotherapy of patients with advanced melanoma, using tumor-infiltrating lymphocytes modified by retroviral gene transduction. N Engl J Med. 323: 570–578.
- Rosi NL, Giljohann DA, Thaxton CS, Lytton-Jean AK, Han MS, Mirkin CA. 2006. Oligonucleotide-modified gold nanoparticles for intracellular gene regulation. Science. 312:1027–1030.
- Sandhu KK, McIntosh CM, Simard JM, Smith SW, Rotello VM. 2002. Gold nanoparticle-mediated transfection of mammalian cells. Bioconjug Chem. 13:3–6.
- Schaffert D, Wagner E. 2008. Gene therapy progress and prospects: synthetic polymer-based systems. Gene Ther. 15:1131–1138.
- Schmidt P. 1997. Pharmazeutische Technologie: Moderne Arzneiformen. Lehrbuch für Studierende der Pharmazie, Nachschlagewerk für Apotheker in Offizin, Krankenhaus und Forschung. Von RH Müller und GE Hildebrand. 348 Seiten, 117 Abbildungen, 57 Tabellen. Wissenschaftliche Verlagsgesellschaft mbH, Stuttgart 1997. DM 64,‐, ISBN 3‐8047‐1504‐4. Pharm Unserer Zeit. 26:323–323.
- Schwarz C, Mehnert W, Lucks JS, Müller RH. 1994. Solid lipid nanoparticles (SLN) for controlled drug delivery. I. Production, characterization and sterilization. J Control Release. 30: 83–96.
- Smith A. 1986. Evaluation of poly (lactic acid) as a biodegradable drug delivery system for parenteral administration. Int J Pharm. 30: 215–220.
- Suh W, Han SO, Yu L, Kim SW. 2002. An angiogenic, endothelial-cell-targeted polymeric gene carrier. Mol Ther. 6:664–672.
- Thomas M, Klibanov AM. 2003. Conjugation to gold nanoparticles enhances polyethylenimine's transfer of plasmid DNA into mammalian cells. Proc Natl Acad Sci. 100:9138–9143.
- Westesen K, Siekmann B. 1997. Investigation of the gel formation of phospholipid-stabilized solid lipid nanoparticles. Int J Pharm. 151:35–45.
- Wightman L, Kircheis R, Rössler V, Carotta S, Ruzicka R, Kursa M, Wagner E. 2001. Different behavior of branched and linear polyethylenimine for gene delivery in vitro and in vivo. J Gene Med. 3:362–372.
- Wissing S, Kayser O, Müller R. 2004. Solid lipid nanoparticles for parenteral drug delivery. Adv Drug Deliv Rev. 56:1257–1272.
- Wivel NA, Wilson JM. 1998. Methods of gene delivery. Hematol Oncol Clin North Am. 12:483–501.
- Wolfert MA, Dash PR, Nazarova O, Oupicky D, Seymour LW, Smart S, et al. 1999. Polyelectrolyte vectors for gene delivery: influence of cationic polymer on biophysical properties of complexes formed with DNA. Bioconjug Chem. 10:993–1004.
- Xiao Y, Qian H, Young WG, Bartold PM. 2003. Tissue engineering for bone regeneration using differentiated alveolar bone cells in collagen scaffolds. Tissue Eng. 9:1167–1177.
- Yang S, Leong KF, Du Z, Chua CK. 2001. The design of scaffolds for use in tissue engineering. Part I. Traditional factors. Tissue Eng. 7:679–689.
- Yin Y, Alivisatos AP. 2004. Colloidal nanocrystal synthesis and the organic–inorganic interface. Nature. 437:664–670.
- zur Mühlen A, Schwarz C, Mehnert W. 1998. Solid lipid nanoparticles (SLN) for controlled drug delivery–drug release and release mechanism. Eur J Pharm Biopharm. 45:149–155.