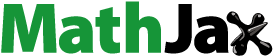
Abstract
Today, technologies based on magnetic nanoparticles (MNPs) are regularly applied to biological systems with diagnostic or therapeutic aims. Nanoparticles made of the elements iron (Fe), gadolinium (Gd) or manganese (Mn) are generally used in many diagnostic applications performed under magnetic resonance imaging (MRI). Similar to molecular-based contrast agents, nanoparticles can be used to increase the resolution of imaging while offering well biocompatibility, poisonousness and biodistribution. Application of MNPs enhanced MRI sensitivity due to the accumulation of iron in the liver caused by discriminating action of the hepatobiliary system. The aim of this study is about the use, properties and advantages of MNPs in MRI.
Introduction
Nanoparticles may provide advanced biomedical research tools based on polymeric or inorganic formulations. They can be used in many different medical and biological applications as in diagnostic test assays for early recognition of disease (Deerinck Citation2008, Gilmore and Spelke Citation2008, Hoffman-Amtenbrink et al. Citation2009, Son et al. Citation2007). The requirements for nanoparticles as biomedical agents span a broad range of narrative research strategies, materials and synthesis techniques. Diagnostic and therapeutic strategies need a different chemical or physical property of the particles included, which depend on the particular function played by the nanoparticles in that treatment (e.g. vector, porous receptacle, magnetic moment carrier). Magnetic nanoparticles (MNPs) are one category of these wide cancer-therapy designed nanoparticles (Goya et al. Citation2008, Oberdörster et al. Citation2005).
MNPs are spherical nanocrystals of 10–20 nm of size with a Fe3+ and Fe2+ core, sometimes enclosed by different molecules, such as dextran or polyethylene glycol (Lu et al. Citation2007, Pantic Citation2012, Sanvicens and Marco Citation2008). MNPs do not include any inert or non-degradable moieties, and it has been demonstrated that the iron oxide core of these agents is metabolized during two weeks, after which the free iron is used in the production of new red blood cells (Sosnovik Citation2007). The magnetic properties allow them to mark various biomolecules in new diagnostic techniques such as magnetic resonance imaging (MRI). In addition, they might be functionalized for dynamic targeting in vivo or for in vitro diagnostics (Lu et al. Citation2007, Pantic Citation2012, Sanvicens and Marco Citation2008).
The paradigmatic example is the MRI, a method which uses the magnetic moments of MNPs as a disorder of the proton resonance to achieve images (Goya et al. Citation2008). MRI is one of the high resolution (75–300 µs), most confident and multi-function imaging modalities of modern medicine. It also allows the exploring of cells, molecules and drug delivery carriers in the human body. The use of nanoparticles in modalities like MRI can significantly increase sensitivity, showing the potential for high-resolution molecular imaging. MRI has high spatial resolution (Ito and Cotsarelis Citation2005, Jun et al. Citation2007), which is compatible in nature, uses non-ionizing radiation and proposes multi-planar tomographic capabilities (Jun et al. Citation2007). Nanoparticles can be engineered to have magnetic characteristics that can be detected by MRI at low concentrations, and simultaneously include ligands which target particular molecules (Jun et al. Citation2007, Lodhia et al. Citation2012).
MRI scanners do not directly detect MNPs. They induce contrast improvement effects on the recreated MR images, either as a signal increase (positive contrast agents) or a signal decrease (negative contrast agents). MRI can supply a high spatial resolution as awaited, which is sometime of inadequate sensitivity by other hypointense areas. In attempting to find more complementary and efficient information about the physical structure and physiological function for disease diagnosis, novel nanoparticulate-based imaging platform is highly desired to combine the advantages of each imaging modality (Kolosnjaj-Tabi et al. Citation2014). In this review, we focus on the application of MNPs and their properties and advantages in MRI. We will also discuss the contrast agents (CAs) enhancement by using MNPs in this study.
MNPs in MRI
There are several imaging modalities clinically available such as MRI, computed tomography (CT), ultrasound (US), positron emission tomography (PET) or single photon emission computed tomography. Every technique has its own benefits and negatives but combining techniques can compensate for drawbacks in any specific technique () (Cheon and Lee Citation2008, Massoud and Gambhir Citation2003, Rudin and Weissleder Citation2003).
Table I. Comparison of several imaging modalities (Cheon and Lee Citation2008, Hachani et al. Citation2011, Massoud and Gambhir Citation2003).
MRI is among today’s best studied imaging modalities for cellular tracking and labeling as it presents several advantages, for example, a great spatial (Kouhi et al. Citation2014, Smirnov Citation2008) and contemporary resolution, and the use of non-ionizing radiation. In medical performances, MRI detects the signal of the protons present in water, which will reply to a magnetic field in an environment-dependant method (i.e. different tissues) (Hachani et al. Citation2011).
The strength of the magnetic field in usual MRI scanners is in the range of 1–3 T, which is 20,000–60,000 times stronger than the Earth’s magnetic field. When a patient is introduced in the gantry, the hydrogen protons (1H) align their spins along the direction of the magnetic field. The sum of each one of the magnetic moments of these spins represents the macroscopic magnetization vector (M) of the biological tissue (Lodhia et al. 2012).
Contemporary methods, containing US, CT and MRI, notice liver tumors only when they have grown to about 5 cm in diameter. By means of that time, the cancer is specifically aggressive, resisting chemotherapy and hard to remove surgically (Blasiak et al. Citation2013).
Lately, there have been many efforts to develop nano-sized difference agents for MRI and CT that are more active and practical than those present on today’s marketplace. Convinced scientists study superparamagnetic iron oxide particles (50–100 nm in size) as promising difference agents, because they have much greater magnetic susceptibility than traditional MR distinctions, for example, gadolinium (Gd) (Cuenca Citation2006, Pantic Citation2012). Contrarily to compute CT, a different functional imaging modality, it does not maintain by on ionizing radiation.
In 2009, it was anticipated that ultrasmall superparamagnetic elements of iron oxide (USPIO)-enhanced MRI in combination with diffusion-weighted magnetic character imaging could be a different, accurate and fast technique for detecting pelvic lymph node metastases even in normal-sized nodes of patients with bladder or prostate cancer (Abbasi et al. Citation2014, Pantic Citation2012).
Application of iron-based nanoparticles enhanced MRI sensitivity due to the accumulation of iron in the liver caused by discriminating action of the hepatobiliary system (). This kind of contrast delivery does not apply, though, to most of the cancers consequently targeted, and active transfer is used (Blasiak et al. Citation2013).
Figure 1. An MRI (gradient echo, TR/TE = 100/4 ms, flip angle 30°, FOV = 5.5 × 2.5 cm, 256 × 256) of a mouse liver obtained at 9.4 T (A) before and (B) after injection of iron oxide (Nano-Ocean, Springdale, AR). The decrease of MR signal within the liver (yellow arrow) is visible (Blasiak et al. Citation2013).
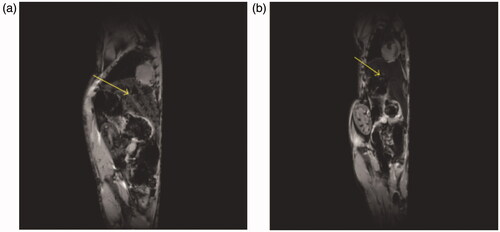
To order a difference agent, the special of core and monolayer material is a critical step because this composition defines the primary physical and chemical properties and also reactivity, solubility and interfacial interactions. Most common core between the MRI CAs are paramagnetic lanthanide metals (Gd, manganese and dysprosium ion complexes) and superparamagnetic magnetite elements (iron oxides) (Yurt and Kazancı Citation2008). Iron oxide elements are widely studied in MRI applications as they change the relaxation times of tissues in which they are current and due to the low toxicity when associated to Gd chelates (Silva et al. Citation2012). From the opinion of view of MRI method, to increase MRI sympathy, two types of difference agents, providing positive or negative image difference, are used (Blasiak et al. Citation2013).
Contrast agents (CAs)
CAs are normally defined, created on their reduction properties, their magnetic properties and their biodistribution. When describing a CA based on reduction properties, the efficiency is described by the longitudinal and transverse relaxivity R1 and R2, respectively. The relativity reflects the alteration in the reduction rate as a function of the CA concentration. The relativities are affected by the size and the composition of these elements (Silva et al. Citation2012, Yurt and Kazancı Citation2008).
CAs have been progressive since the inception of MRI, to selectively alteration the longitudinal and transverse reduction times (T1 and T2) of 1H in biological tissues. Such energy transmissions mostly happen though interactions taking place among the magnetic fundamentals and the spins of hydrogen protons in their vicinity (Caravan Citation2006, Caravan Citation1999). In parallel with the expansion of paramagnetic CAs, progress in the field of MRI CAs also occurred through the exceptional properties of iron oxide NPs. By opposition to paramagnetic molecules, which produce “positive” contrast in MRI, iron oxide NPs are well-known for their potential to rise the signal.
MRI positive CAs
CAs for longitudinal reduction of protons mostly comprise of paramagnets as these possess a great number of free electrons (lanthanides, for example) and shorten T1, thus forming bright difference (Caravan Citation1999, Fallahzadeh et al. Citation2010). The shortening of T1 depends solely on the direct conversation of energy among hydrogen protons in water molecules and the paramagnetic component’s outer shell electrons, hence an indirect correlation with the external of NPs (Hachani et al. Citation2011).
MRI negative CAs
Negative difference can be provided by superparamagnetic iron oxide NPs which condense the T2 relaxation time and create a darker image. Two main factors perform a role in the contrast enhancement: the iron oxide core and its covering. The limitation of T2 is determined by transverse reduction of hydrogen protons of water molecules surrounding the superparamagnetic iron oxide NPs (Gillis and Koenig Citation1987, Hachani et al. Citation2011).
MRI contrast enhancement
Both T1 and can be condensed by the use of a magnetic CA. The most public CAs presently used are paramagnetic Gd ion complexes, though agents based on superparamagnetic nanoparticles are commercially presented, for example, “Feridex I. V.,” an iron oxide CA marketed by Advanced Magnetics Inc. (Rochester, IN) for the organ-specific targeting of liver lesions. The superparamagnetic elements used are magnetically saturated in the normal range of magnetic field strengths used in MRI scanners, thus founding a considerable locally perturbing dipolar field which leads, via Eq. (Equation1
(1)
(1) ), to a marked shortening of
() along with a less marked reduction of T1 (Elster Citation1994)
(1)
(1)
where ΔB0 is the difference in the field brought about either through distortions in the homogeneity of the functional field itself, or by local differences in the magnetic susceptibility of the organization (Brown and Semelka Citation2015).
is the smaller relaxation time and γ is the gyromagnetic ratio which γ = 2.67 × 108 rad s−1 T−1 for 1H protons (Pankhurst et al. Citation2003).
Figure 2. Effect of magnetic particle internalization in cells on relaxation times: (A) the protons in cells tagged by magnetic particles have a shorter
relaxation time than those in (B) untagged cells (Pankhurst et al. Citation2003, Yang et al. Citation2010).
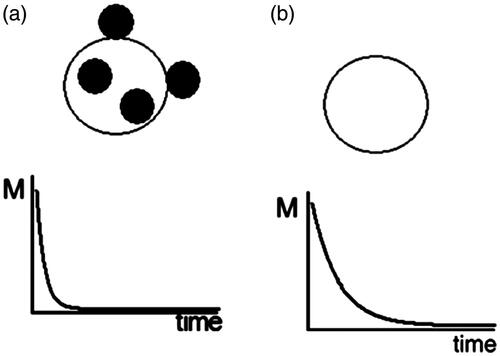
Gd: Paramagnetic
Gadolinium-based difference agents are intravenous drugs used in diagnostic imaging actions to improve the quality of MRI or magnetic resonance angiography (MRA). Gd (III) containing MRI difference agents (often termed just “gado” or “gad”) are the most usually used for improvement of vessels in MRA or for brain tumor improvement associated with the degradation of the blood-brain barrier. For great vessels, for example, the aorta and its branches, the Gd (III) dose can be as low as 0.1 mmol per kg body mass. Higher attentions are frequently used for finer vasculature. Gd (III) chelates do not agreement the blood-brain barrier as they are hydrophilic. Therefore, these are convenient in enhancing lesions and tumors where the Gd (III) leaks out. In the rest of the body, the Gd (III) originally remains in the circulation but then dispenses into the interstitial space or is reduced by the kidneys (Hosseininasab et al. Citation2014). For instance, a free solubilized aqueous ion, Gd (III) is slightly toxic, but was usually viewed as safe when administered as a chelated complex. In animals at liberty Gd (III) ion displays a 100–200 mg/kg 50% lethal dose, but the LD50 is improved by a factor of 100 when Gd (III) is chelated, thus that its toxicity becomes comparable to iodinated X-ray difference complexes (Penfield and Reilly Citation2007). The chelating carrier molecule for Gd for MRI contrast use can be categorized by whether they are macrocyclic or have a right geometry and whether they are ionic or not. Cyclical ionic Gd (III) complexes are considered the least likely to release the Gd (III) ion, and, therefore, the safest.
Properties of MNPs for MRI
A very great fraction of molecular and cellular MRI submissions are based on the design and fabrication of MNPs consisting of: a) an inorganic nanocrystal core, for example, Fe2O3/Fe3O4, Gd2O3, MnO, NaGdF4), b) a coating completed of small ligands or biocompatible biological molecules and c) an efficient anchor that is used to graft particular molecules, complementary imaging functionalities (e.g. radioactive atoms or fluorescent molecules), or medicinal complexes for drug delivery.
The MRI difference enhancement by MNPs is dependent on their structure, size, external things and degree of aggregation (). It has been confirmed for instance that size is a critical parameter inducing the transverse relaxivity R2 (Tromsdorf et al. Citation2007) although the surface contact influences the longitudinal relaxivity R1 (Hachani et al. Citation2011, Tromsdorf et al. Citation2007). Great efforts have been devoted to construct multifunctional lanthanide-based nanoprobes for multi-modality imaging (Billotey et al. Citation2003, Corot Citation2006, Darrasse and Ginefri Citation2003, Karnoosh-Yamchi et al. Citation2014, Lévy et al. Citation1993, Pawelczyk et al. Citation2009, Smirnov Citation2008, Wilhelm et al. Citation2002). Some lanthanide ions with unpaired 4f electrons, like Gd3+ ions, are generally applied as CAs for MRI. Gd-, Yb- and Lu-doped nanostructures have been used as exceptional CT difference agents due to their stronger X-ray reduction and higher atomic number than those of usually used small iodinated molecules (Alizadeh et al. Citation2014, Chaudeurge et al. Citation2002, Fayol et al. Citation2002, Kolosnjaj-Tabi et al. Citation2014, Wilhelm et al. Citation2007).
Table II. Comparison of some nanoparticles with high transverse relaxivity for T2 MRI (Benyettou et al. Citation2011, Hachani et al. Citation2011, Huang et al. Citation2011, Jung and Jacobs Citation1995, Ruiz et al. Citation2006).
Iron oxide NPs are, in general, separated in two classes: small elements of iron oxide (SPIO) and ultrasmall components of iron oxide (USPIO). SPIOs are complete iron oxide cores of mean diameters in the range 3–20 nm; though the method agglomerates of hydrodynamic diameter usually superior to 50 nm. The theory of hydrodynamic diameter () mentions to the total actual diameter of an element suspended in a fluid and forming a colloid. The hydrodynamic diameter is mostly measured by means of laser analysis (dynamic light scattering), by evaluating the Brownian motion of the put off NPs. USPIOs instead, refer to the class of iron oxide NPs that are completed of iron oxide cores (3–20 nm diameter) bounded by a coating of molecules that preserve their individuality (mean diameter <50 nm).
Figure 3. Schematic representations of (A) the structure of functional magnetic nanoparticles (MNPs) for MRI applications and (B) a colloidal nanoparticle suspended in biological media.
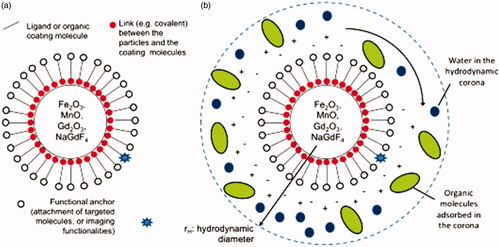
Nanoparticle coating
NPs must form steady colloids in physiological media (blood, plasma, lymph and urine) for being considered in MRI applications. They must also show respectable biocompatibility and stretched vascular retention. The ligands and polymers, which are used as element coverings, must capably cover the particles and stimulate their individualization. NPs must be coated with a surface ligand or a polymer that either induces an electrostatic repulsion among the elements, or exert a strong steric attraction among them (Daraee et al. Citation2014). Amongst superparamagnetic difference agent, dextran-covered iron oxides are biocompatible and are excreted via the liver after the action. They are selectively taken up by the reticuloendothelial system, a system of cells lining blood vessels whose purpose is to remove foreign materials from the bloodstream; MRI contrast relies on the differential acceptance of different tissues (Lawaczeck et al. Citation1997, Pankhurst et al. Citation2003).
Conclusion
Superparamagnetic iron oxide NPs are proving to be a division of narrative probes practical for in vitro and in vivo cellular and molecular imaging. Superparamagnetic CAs have an improvement of producing an enhanced proton relaxation in MRI in comparison with paramagnetic ones. As a result, less amount of an SPIO mediator are required to dose the human body than a paramagnetic one. To relate the magnetic fluids to an MRI CA, an SPIO should be dispersed into a biocompatible and biodegradable carrier.
Acknowledgements
The authors thank the Department of Medical Nanotechnology, Faculty of Advanced Medical Science of Tabriz University for all the supports provided.
Declaration of interest
The authors declare that they have no competing interests. This work is funded by 2015 Drug Applied Research Center Tabriz University of Medical Sciences Grant.
References
- Abbasi E, Aval SF, Akbarzadeh A, Milani M, Nasrabadi HT, Joo SW, et al. 2014. Dendrimers: synthesis, applications, and properties. Nanoscale Res Lett. 9:247.
- Alizadeh E, Akbarzadeh A, Eslaminejad MB, Barzegar A, Hashemzadeh S, Nejati-Koshki K, Zarghami N. 2014. Up-regulation of liver enriched transcription factors (HNF4a and HNF6) and liver specific microRNA (miR-122) by inhibition of Let-7b in mesenchymal stem cells. Chem Biol Drug Des. 85:600–608.
- Benyettou F, Lalatonne Y, Chebbi I, Di Benedetto M, Serfaty JM, Lecouvey M, Motte L.. 2011. A multimodal magnetic resonance imaging nanoplatform for cancer theranostics. Phys Chem Chem Phys. 13:10020–10027.
- Billotey C, Wilhelm C, Devaud M, Bacri JC, Bittoun J, Gazeau F. 2003. Cell internalization of anionic maghemite nanoparticles: quantitative effect on magnetic resonance imaging. Magn Reson Med. 49:646–654.
- Blasiak B, van Veggel FCJM, Tomanek B. 2013. Applications of nanoparticles for MRI cancer diagnosis and therapy. J Nanomater. 2013:148578.
- Brown MA, Semelka RC. 2015. MRI: Basic Principles and Applications. John Wiley & Sons International Society for Autism Research.
- Caravan P. 1999. Gadolinium (III) chelates as MRI contrast agents: structure, dynamics, and applications. Chem Rev. 99:2293–2352.
- Caravan P. 2006. Strategies for increasing the sensitivity of gadolinium based MRI contrast agents. Chem Soc Rev. 35:512–523.
- Chaudeurge A, Wilhelm C, Chen-Tournoux A, Farahmand P, Bellamy V, Autret G, et al. 2002. Can magnetic targeting of magnetically labeled circulating cells optimize intramyocardial cell retention? Cell Transplant. 21:679–691.
- Cheon J, Lee JH. 2008. Synergistically integrated nanoparticles as multimodal probes for nanobiotechnology. Acc Chem Res. 41:1630–1640.
- Corot C. 2006. Recent advances in iron oxide nanocrystal technology for medical imaging. Adv Drug Deliv Rev. 58:1471–1504.
- Cuenca AG. 2006. Emerging implications of nanotechnology on cancer diagnostics and therapeutics. Cancer 107:459–466.
- Daraee H, Etemadi A, Kouhi M, Alimirzalu S, Akbarzadeh A. 2014. Application of liposomes in medicine and drug delivery. Artif Cells Nanomed Biotechnol. [Epub ahead of print]. DOI:https://doi.org/10.3109/21691401.2014.953633.
- Darrasse L, Ginefri JC. 2003. Perspectives with cryogenic RF probes in biomedical MRI. Biochimie. 85:915–937.
- Deerinck TJ. 2008. The application of fluorescent quantum dots to confocal, multiphoton, and electron microscopic imaging. Toxicol Pathol. 36:112–116.
- Ebrahimi E, Akbarzadeh A, Abbasi E, Khandaghi AA, Abasalizadeh F, Davaran S. 2014. Novel drug delivery system based on doxorubicin-encapsulated magnetic nanoparticles modified with PLGA-PEG1000 copolymer. Artif Cells Nanomed Biotechnol. [Epub ahead of print]. DOI:https://doi.org/10.3109/21691401.2014.944646.
- Elster AD. 1994. Questions and Answers in Magnetic Resonance Imaging. St. Louis: Mosby-Year Book. Inc.
- Fallahzadeh S, Bahrami H, Akbarzadeh A, Tayarani M. 2010. High-isolation dual-frequency operation patch antenna using spiral defected microstrip structure. IEEE Antennas Wireless Propag Lett. 9:122–124.
- Fayol M, Chalard B. 2002. Use of magnetic forces to promote stem cell aggregation during differentiation, and cartilage tissue modeling. Adv Mater. 25:2611–2616.
- Gillis P, Koenig SH. 1987. Transverse relaxation of solvent protons induced by magnetized spheres: application to ferritin, erythrocytes, and magnetite. Magn Reson Med. 5:323–345.
- Gilmore CK, Spelke ES. 2008. Novel nanomaterials for clinical neuroscience. Cognition. 107:932–945. 933.
- Goya GF, Grazu V, Ibarra MR. 2008. Magnetic nanoparticles for cancer therapy. Curr Nanosci. 4:1–16.
- Hachani R, Biskri L, Rossi G, Allaoui A. 2011. Tracking stem cells in tissue-engineered organs using magnetic nanoparticles. Nanoscale. 5:11362–11373.
- Hosseininasab S, Pashaei-Asl R, Khandaghi AA, Nasrabadi HT, Nejati-Koshki K, Akbarzadeh A, et al. 2014. Synthesis, characterization, and in vitro studies of PLGA-PEG nanoparticles for oral insulin delivery. Chem Biol Drug Des. 84:307–315.
- Huang J, Chakraborty P, Lundstrom CC, Holmden C, Glessner JJG. 2011. Casein-coated iron oxide nanoparticles for high MRI contrast enhancement and efficient cell targeting. ACS Appl Mater Interfaces. 5:4632–4639.
- Ito A, Cotsarelis G. 2005. Medical application of functionalized magnetic nanoparticles. J Biosci Bioeng. 100:1–11.
- Jun YW, Lee JH, Cheon J. 2007. Nanoparticle contrast agents for molecular magnetic resonance imaging. In Nanobiotechnology II: More Concepts and Applications, 321–346.
- Jung CW, Jacobs P. 1995. Physical and chemical properties of superparamagnetic iron oxide MR contrast agents: ferumoxides, ferumoxtran, ferumoxsil. Magn Reson Imaging. 13:661–674.
- Karnoosh-Yamchi J, Mobasseri M, Akbarzadeh A, Davaran S, Ostad-Rahimi AR, Hamishehkar H, et al. 2014. Preparation of pH sensitive insulin-loaded nano hydrogels and evaluation of insulin releasing in different pH conditions. Mol Biol Rep. 41:6705–6712.
- Kolosnjaj-Tabi J, Di Corato R, Lartigue L, Marangon I, Guardia P, Silva AKA, et al. 2014. Cell labeling with magnetic nanoparticles: opportunity for magnetic cell imaging and cell manipulation. J Nanobiotechnol. 11:S7.
- Kouhi M, Vahedi A, Akbarzadeh A, Hanifehpour Y, Joo SW. 2014. Investigation of quadratic electro-optic effects and electro absorpti process in GaN/AlGaN spherical quantum dot. Nanoscale Res Lett. 9:131–136.
- Lawaczeck R, Bauer H, Frenzel T, Hasegawa M, Ito Y, Kito K. 1997. Magnetic iron oxide particles coated with carboxydextran for parenteral administration and liver contrasting: pre-clinical profile of SH U555A. Acta Radiol. 38:584–597.
- Lévy KN, Meehan KB, Temes CM, Yeomans FE, et al. 1993. How cellular processing of superparamagnetic nanoparticles affects their magnetic behavior and NMR relaxivity. Contrast Media Mol Imaging. 7:373–383.
- Lodhia J, Saunders C, Shawe-Taylor J, Cristianini N, Watkin C. 2012. Development and use of iron oxide nanoparticles (Part 1): synthesis of iron oxide nanoparticles for MRI. Biomed Imaging Interv J. 6:e12.
- Lu AH, Salabas EL, Schüth F. 2007. Magnetic nanoparticles: synthesis, protection, functionalization, and application. Angew Chem Int Ed Engl. 46:1222–1244.
- Massoud TF, Gambhir SS. 2003. Molecular imaging in living subjects: seeing fundamental biological processes in a new light. Genes Dev. 17:545–580.
- Nanostructured Materials for Biomedical Applications, 2009: ISBN: 978-81-7895-397-7 Editor: M. C. Tan Transworld Research Network 37/661 (2), Fort P.O., Trivandrum-695 023, Kerala, India.
- Nejati-Koshki K, Mesgari M, Ebrahimi E, Abbasalizadeh F, Fekri Aval S, Khandaghi AA, et al. 2014. Synthesis and in-vitro study of cisplatin-loaded Fe3O4 nanoparticles modified with PLGA-PEG6000 copolymers in treatment of lung cancer. J Microencapsul. 31:815–23.
- Oberdörster G, Maynard A, Donaldson K, Castranova V, Fitzpatrick J, Ausman K, et al. 2005. Principles for characterizing the potential human health effects from exposure to nanomaterials: elements of a screening strategy. Part Fibre Toxicol. 2:8.
- Pankhurst QA, Connolly J, Jones SK, Dobson J. 2003. Applications of magnetic nanoparticles in biomedicine. J Phys D Appl Phys. 36:R167.
- Pantic I. 2012. Magnetic nanoparticles in cancer diagnosis and treatment: novel approaches. Rev Adv Mater Sci. 26: 67–73.
- Pawelczyk E, Jordan EK, Balakumaran A, Chaudhry A, Gormley N, Smith M, et al. 2009. In vivo transfer of intracellular labels from locally implanted bone marrow stromal cells to resident tissue macrophages. PLoS One. 4:e6712.
- Penfield JG, Reilly RFJr. 2007. What nephrologists need to know about gadolinium. Nat Clin Pract Nephrol. 3:654–668.
- Rudin M, Weissleder R. 2003. Molecular imaging in drug discovery and development. Nat Rev Drug Discov. 2:123–131.
- Ruiz GM, García-Martínez MC, Holgado F. 2006. Biodistribution and pharmacokinetics of uniform magnetite nanoparticles chemically modified with polyethylene glycol. Nanoscale. 5:11400–11408.
- Sanvicens N, Marco MP. 2008. Multifunctional nanoparticles – properties and prospects for their use in human medicine. Trends Biotechnol. 26:425–433.
- Silva A, Silva-Freitas E, Carvalho J, Pontes T, Araújo-Neto R, Silva K, Carriço A, Egito E et al. 2012. Magnetic Particles in Biotechnology: From Drug Targeting to Tissue Engineering. Chapter 13. Intechopen. DOI: https://doi.org/10.5772/30624.
- Smirnov P. 2008. In vivo single cell detection of tumor-infiltrating lymphocytes with a clinical 1.5 Tesla MRI system. Magn Reson Med. 60:1292–1297.
- Son SJ, Bai X, Lee SB. 2007. Inorganic hollow nanoparticles and nanotubes in nanomedicine Part 1. Drug/gene delivery applications. Drug Discov Today. 12:650–656.
- Sosnovik DE. 2007. The promise of molecular MRI in cardiovascular imaging. Technology and Trends. (2):118–122.
- Tromsdorf UI, Bigall NC, Kaul MG, Bruns OT, Nikolic MS, Mollwitz B, et al. 2007. Size and surface effects on the MRI relaxivity of manganese ferrite nanoparticle contrast agents. Nano Lett. 7:2422–2427.
- Wilhelm WW, Johnson JMF, Karlen DL, Lightle DT. 2007. Magnetic control of vascular network formation with magnetically labeled endothelial progenitor cells. Biomaterials. 28:3797–3806.
- Wilhelm C, Gazeau F, Bacri JC. 2002. Magnetophoresis and ferromagnetic resonance of magnetically labeled cells. Eur Biophy J. 31:118–125.
- Yang J, Benyamin B, McEvoy BP, Gordon S, Henders AK, et al. 2010. Potential of magnetic nanoparticles for targeted drug delivery. Nanotechnol Sci Appl. 5:73.
- Yurt Al, Kazancı N. 2008. Investigation of magnetic properties of various complexes prepared as contrast agents for MRI. J Mol Struct. 892:392–397.