Abstract
The polyelectrolyte complexes (PECs) are versatile formulations formed by electrostatic interactions between oppositely charged biopolymers. PECs have been investigated widely by the researchers to explore the virtues of this formulation viz. high biocompatibility, excellent biodegradability, low toxicity, cost-effective, environment-friendly, and energy-efficient production. The prime object of the present review is to present the prominent features of PECs including mechanism of PEC formation, structural models of PECs, interactions involved in PEC formation, steps involved in PEC fabrication, factors affecting the formation of PECs and applications of PECs. The patents pertaining to PECs have briefly been tabulated as well.
Introduction
Coulomb’s (electrostatic) interactions between charged microdomains of two oppositely charged polyionic components result in the formation of polyelectrolyte complexes (PECs) (Dautzenberg and Kriz Citation2003, Schatz et al. Citation2004a, Wu and Delair Citation2015). PEC formation, in general, results in the formation of optically homogeneous and stable nanodispersion with dimensions in a colloidal size range (Mao et al. Citation2006, Sun et al. Citation2008). The avoidance of the chemical cross-linking agents reduces the possible toxicity and other undesirable effects of the involved reagents (Lankalapalli and Kolapalli Citation2009).
PECs can be formed with different number of structures and properties, which grants to them, a wide range of applications in different technological and scientific fields viz. medicine, pharmacy, biotechnology and tissue engineering, biomaterials and biomedicals, cosmetics, and so on (Koetz and Kosmella Citation2007). As PECs are biodegradable, biocompatible, and non-toxic, they find utility as a subject of extreme interest in pharmaceutical and biomedical research (Boeris et al. Citation2007).
In the present review, we highlight the key aspects of PECs like structural models of PECs, preparation methodologies, factors affecting the formation and stability of PECs, and characterization of PECs. We also discuss potential applications of PECs, especially in pharmaceutical and biomedical fields. As a framework, we start by introducing the basic mechanism of PEC formation and interactions involved therein. Few patents pertinent to PECs have been tabulated briefly.
Mechanism of PEC formation
The PECs are formed as a consequence of strong electrostatic (Coulomb’s) interactions between charged counterparts of at least two oppositely charged polyelectrolytes () (Dautzenberg and Kriz Citation2003; Schatz et al. Citation2004a, Wu and Delair Citation2015). Quantitatively, PECs are categorized as either stoichiometric (S-PECs; PECs prepared by polymers in equimolar ratio), or non-stoichiometric (N-PECs; one polymer in excess compared to another, so that the resulting complex becomes more hydrophilic and soluble) (Robertis et al. Citation2015).
Table 1. List of natural and synthetic polymers having PEC-forming potential.
The worldwide consensus among researchers is that the PEC formation is an entropy-driven phenomenon. The contributing force for the formation of PECs in aqueous solutions is the release of low molecular weight counterions (which were previously associated with the charged groups on the polymer chains) that results in a gain in entropy by the system (Michaels and Miekka Citation1961, Pergushov et al. Citation2012, Philipp et al. Citation1989). Kabanov and Zezin (Citation1984) first investigated the kinetics of complex formation and stop-flow measurements have demonstrated that this complex formation process takes place in less than 5 ms.
The mechanism of PEC formation can be viewed, at least in its initial stage, as the net effect of two counteracting processes (Philipp et al. Citation1989), namely:
Electrostatic charge compensation leads to the ordering of two oppositely charged polyions to a complex molecule and this occurs in connection with cooperative effects inducing conformational changes favorable for mutual charge compensation;
A “chaotic” aggregation of polyanions and polycations with only partial mutual charge compensation and a considerable number of ionic sites still charge-compensated by low molecular weight counterions.
The basic mechanism of PEC formation, as illustrated in , involves three major steps:
Primary complex formation;
New bond formation within intracomplexes;
Intercomplex aggregation.
Figure 1. Schematic representation of the aggregation of PECs. Figure depicts the process of PEC formation with three major steps involved, namely Primary complex formation; new bond formation process within intracomplexes; intercomplex aggregation process (modified after Tsuchida 1994).
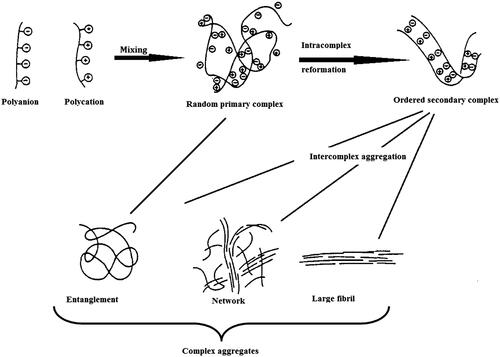
The first step proceeds with the establishment of secondary binding forces such as Coulomb’s interactions immediately after mixing oppositely charged polyelectrolyte solutions. This reaction is very rapid. The second step proceeds within the order of an hour and involves the formation of new bonds and/or the correction of the distortions of the polymer chains to define new conformation of the polymer chains. The third step involves the aggregation of secondary complexes, mainly through hydrophobic interactions. The final aggregates of the PECs are insoluble in ordinary solvents, and the molar ratio of the polymer components in the aggregates is almost unity (Tsuchida Citation1994).
PECs were proposed to consist of two models (), on the basis of supermolecular order of polymeric chains in a PEC, namely Ladder-like model with an ordered chain packing and Scrambled egg model with disordered packing (Pergushov et al. Citation2012). From all the experimental evidences available today, it has been concluded that the above-mentioned models represent the limiting cases, with the reality being existing in between these two models, but the closer to the latter than to the former model (Philipp et al. Citation1989).
Structural models of PECs
The three different types of structures can result, while in solution, from polycation–polyanion interactions, namely (i) water soluble, (ii) colloidally stable, and (iii) two phase systems (Koetz and Kosmella Citation2007).
Water-soluble aggregates can be formed at molecular scale when polyelectrolytes with weak ionic groups and large differences in molar mass are mixed in a non-stoichiometric ratio (i.e., the ratio of cationic to anionic functional groups is either >1 or <1 but = 1). Such structures consist of long host molecules sequentially complexed with shorter guest polyions of opposite charge. The concentration of soluble salts and the charge ratio of two polyelectrolytes affect the stability of water-soluble PECs, that as coexist in solution with insoluble and colloidally stable PECs, or aggregates and precipitates (Koetz and Kosmella Citation2007, Zezin and Kabanov Citation1982).
Complex formation between high or similar molecular weight polyelectrolytes produces highly aggregated and macroscopically heterogeneous systems. The complexes obtained from polyelectrolytes having significantly different molar masses, mixed in non-stoichiometric ratios, produces water-soluble aggregates at the molecular scale. However, at low or moderate ionic strengths, in extremely diluted solutions and non-stoichiometric conditions, the aggregation process can be stopped at a colloidal level resulting in colloidally stable PECs (Delair Citation2011; Gucht et al. Citation2011).
The mixing of highly concentrated solutions of high and similar molecular weight polyelectrolytes near stoichiometric ratio forms two-phase systems, consisting of a liquid phase and a PECs-rich phase. The rheological properties of PECs-rich phase depends on the properties of polyelectrolytes and salt concentrations and are similar to the either soft-solids or otherwise have liquid-like properties, being called in this case as complex coacervates (Gucht et al. Citation2011).
Interactions involved in PEC formation
The interactions established during complex formation between polyelectrolytes includes Coulomb’s interactions, hydrogen bonding, hydrophobic interactions, van der Waals interactions, and dipole interactions (Delair Citation2011, Koetz and Kosmella Citation2007, Philipp et al. Citation1989, Robertis et al. Citation2015).
The strong intermolecular interactions existing in between polycation and polyanion, responsible for effective complex formation are referred to as Coulomb’s interactions. In this case, PEC formation process depends on the nature of the polymer in excess. Sæther et al. (Citation2008) proposed a core-shell model of PECs based on electrostatic interactions between chitosan and alginate (). In excess of either chitosan or alginate, the zeta potential has a large positive or negative value and small, non-aggregating particles are formed. This non-aggregating behavior is suggested to arise due to an excess of the major component, which forms a stabilizing shell around the particles. At the crossover from a negative to positive zeta potential, which occurs at K∼1, the results indicated that there is an increment in the average size of PECs. These findings probably reflect the formation of flocks and aggregates. Similar findings were observed near net charge neutrality for chitosan and dextran sulfate (Schatz et al. Citation2004a). The capability to build larger aggregates or agglomerates therefore mainly occurs close to K∼1. represents the preparation methodology and structural model of PECs suggesting core-shell topography of the fabricated PECs (Sæther et al. Citation2008).
Figure 3. Schematic illustration of a core-shell model for polyelectrolyte complexes of alginate (polyanion) and chitosan (polycation) at net charge ratios less than one, equal to one and larger than one (reproduced from Sæther et al. Citation2008, with kind permission of the copyright holder, Amsterdam).
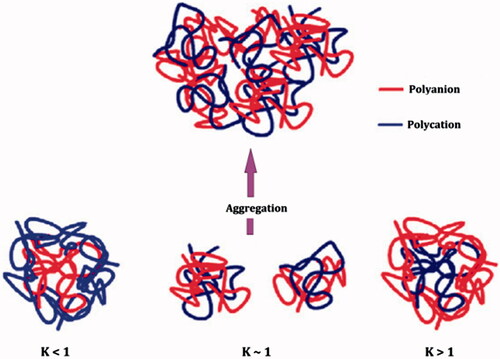
Preparation of PECs
The preparation protocol for the fabrication of PECs, in general, follows the below mentioned steps (Schatz et al. Citation2004b).
Preparation of polyelectrolyte solution
The two oppositely charged polyelectrolytes are dispersed in a suitable dispersion medium (usually deionized water) at various concentrations and in defined stoichiometric ratios, so as to obtain the solutions of both polyelectrolytes with required molar concentrations. A polysalt (like sodium chloride) can be added to obtain required ionic strength. Finally pH is adjusted to a desired value. All prepared solutions are filtered through 0.22 μ millipore membrane filter before use.
PEC formation
The PECs are generally prepared at room temperature using either polycation or polyanion as the starting solution. The complex formation process, in general, can be completed using either the slow addition of polyanionic solution dropwise at a predetermined flow rate to polycationic solution of same ionic strength or the rapid one-shot addition of one polyelectrolyte solution to oppositely charged polyelectrolyte solution of similar ionic strength under a constant speed magnetic stirring.
Particle refinement
Particle refinement is done by centrifugation process and is necessary in order to avoid physisorption of free polymers onto the surface of already formed PECs. The final product is either re-suspended in a minimum volume of deionized water for immediate characterization or is lyophilized for better stability.
Factors affecting the formation and stability of PEC particles: critical experimental aspects
The mixing of two oppositely charged polyelectrolytes usually leads to the separation of a polymer-rich phase (milky phase) from a polymer-depleted phase (clear phase) (Muller 2012). enlists various factors affecting the formation, stability of PECs.
Figure 5. Illustrative representation of various factors affecting the formation and stability of PECs.
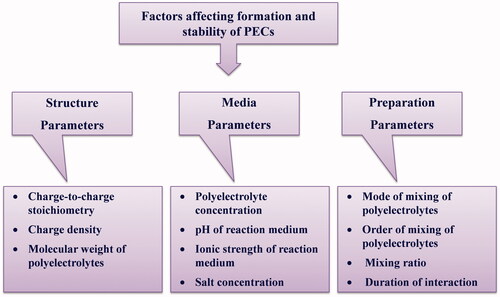
Charge-to-charge stoichiometry
While investigating polyelectrolyte complexation, what needs to be studied is the net ratio of positive to negative charges of the oppositely charged polyelectrolytes involved in fabricating PECs. This ratio is referred as charge ratio and denoted as Z, with the subscripts (+/− or −/+). The charge-to-charge stoichiometry of the formed PECs is denoted as Φ. S-PECs (Φ = 1) are sufficiently hydrophobic due to the mutual screening of the charges and precipitate from the aqueous solution. If the N-PECs (Φ = 1) are prepared, overcharging effects due to either excess polycation or polyanion can be observed (Pergushov et al. Citation2012).
Charge density
Dautzenberg et al. (Citation1982) reported the effect of charge density on PEC properties using cationic and anionic copolymers of acrylamide. They observed that for equal charge density of the polyelectrolyte components, the symplex particles adopt a compact structure, whereas in systems with strongly deviating charge density of the polyelectrolyte components a loose fluctuating structure prevails. Claesson et al. (2009) recently reported the controversial findings with a cationic methacryloxyethyl trimethylammonium (METAC) and anionic poly(styrenesulfonate) (PSS). They observed that with decreasing charge density it was possible to obtain soluble PECs with decreasing hydrodynamic radii of around 20 nm and increasing stability, even for 1:1 stoichiometry.
Molecular weight
Recently, Hu et al. (Citation2012) reported the effect of chitosan (CHT) molecular weight on the particle size of carboxymethylpachyman (CMP)/CHT PEC nanoparticles. Increase in the molecular weight of CHT from 12,000 to 46,000 g/mole resulted in increased particle size of PECs from 135 to 279 nm. In the conclusion, it was underlined that longer chains of positively charged CHT molecules can complex with a large number of negatively charged CMP molecules.
Polyelectrolyte concentration
Schatz et al. (Citation2004a) reported the increase in size of CHT/DS biopolyelectrolyte complex particles with increasing DS concentration, but only at high mixing ratios n+/n−. Muller et al. (Citation2011) suggested that, based on the model of aggregation of primary PEC to secondary PEC particles due to short range dispersive interactions, increasing polyelectrolyte concentration results in reduced electrostatic repulsion between like-charged primary PEC particles and thus, in their elevated dispersive attraction. Furthermore, an increase in polyelectrolyte concentration might also result in a large number of primary PEC particles per volume. Both could lead to larger secondary PEC particles. Exceeding certain polyelectrolyte concentration values can also lead to precipitation.
pH
Polyelectrolyte complexation between chitosan and dextran sulfate sodium prepared at low and high pH values have been investigated by Fukuda and Kikuchi (Citation1977), who suggested that the complexes prepared at different pH conditions were different in their molecular structures. Muller et al. (Citation2011) studied the influence of pH combination on the particle size of PEC system consisting of weak polyelectrolytes poly(ethyleneimine) (PEI) and poly(acrylic acid) (PAC) at molar mixing ratio n−/n+ = 1.5 and observed a decrease in particle size from ∼400 nm to ∼160 nm with decreasing values of pH (10, 8.5, 7, and 4) of the PEI solution at a constant pH of PAC solution. Highly charged particles of PEI/PAC at lower pH may have a lower tendency of coagulation due to mutual electrostatic repulsion, compared to the lower charged particles formed at higher pH due to electrostatic attraction.
Ionic strength
The ionic strength of the polyelectrolytes has great impact on the final size of the PECs. An increase in ionic strength induces a decrease in the average diameter, which could be related to the increase in chain flexibility (Delair Citation2011). The complexation can occur only at pH values in the vicinity of the pKa interval of the two polyelectrolytes. During complexation, polyelectrolytes can either coacervate or form a more or less compact hydrogel. Nevertheless, if ionic strength is too strong, precipitation can occur (Berger et al. Citation2004).
Mode of mixing polyelectrolyte solutions
Mixing of polycation and polyanion solutions to produce PECs is expected to be dependent on the mixing type, mixing protocol, and the device used. Ankerfors et al. (Citation2010) reported the influence of mixing procedure on the complexation of poly(allylamine) (PAH) and PAC by comparing jet mixing with the frequently used colloid titration. They obtained small PECs for both low molecular weight polyelectrolytes at short mixing times, whereas for high molecular weight polyelectrolytes PEC size first decreased with decreasing mixing time until a minimum and then increased again. This behavior was attributed to the diffusion-controlled formation of pre-complexes occurring sufficiently quickly so that stable complexes were formed. However, for larger polyelectrolytes non-equilibrium pre-complexes prone to aggregation were formed. Comparing the two mixing procedures, jet mixing gave smaller PECs, allowing mixing time to control PEC size, whereas colloid titration gave larger PECs. Sæther et al. (Citation2008) observed decrease in particle size of chitosan-alginate PECs with increasing mixing speed using an Ultra-Turrax procedure.
Order of addition
A very sensitive experimental variable was investigated by several researchers, the order of polyelectrolytes addition, while polyelectrolyte solutions are mixed slowly with one another (Mende et al. Citation2004). Schatz et al. (Citation2004a) compared the ordinary slow dropwise mixing with rapid one-shot mixing for the preparation of CHT-DS PECs. Rapid one-shot mixing procedure gave PECs with small diameter and higher stability compared to the PECs prepared by a slow dropwise mixing process. While adding the titrant solution dropwise to the starting solution, the order of mixing was essential and the polymer in default has to be added to the one in excess in order to avoid the aggregate formation. In contrast, the one-shot addition of the titrant solution was insensitive to the order of addition, confirming that the particle formation process is kinetically controlled (Schatz et al. Citation2004a).
Mixing ratio
Dautzenberg (Citation1997) studied the effect of mixing ratio (X) on the molecular weight and particle size of PEC particles between poly(diallyldimethylammonium chloride) (PDADMAC) and poly(styrenesulfonate) (PSS). A slight decrease in molecular weight and a significant decrease in particle size from 400 nm (X = 0.1) to 200 nm (X = 1.0) was found with increasing mixing ratio in a saltless system. It was qualitatively interpreted as an increase in structural density when X approaches 1:1 stoichiometry because mutual charge-compensated chains might be more compactly coiled. In a salted system, the presence of salt (Cs = 0.01 M) increased the particle size from 50 nm (X = 0.1) to about 120 nm (X = 1.0). It was interpreted by a lower aggregation number of the salted system.
Salt concentration
The influence of salt concentration on the properties of PECs can be studied either during complexation or after complexation.
Dautzenberg (Citation1997) prepared PDADMAC/PSS PEC particles at a polyelectrolyte concentration of 0.00025 M (PSS) and 0.0005 M (PDADMAC) in the presence of no salt, 0.01 M and 0.1 NaCl. For no salt, the PEC particle size dropped from around 300 nm at mixing ratio X = 0.1 to about 130 nm at X = 0.95. For 0.01 M NaCl, the size was constant at about 40 nm at X = 0.1–0.9. For 0.1 M NaCl, the PEC size was 50 nm at X = 0.1, which was slightly higher than for 0.01 M, and increased to about 120 nm at X = 0.95. In this investigation, increasing salt concentration favored coagulation of primary PECs to larger secondary PECs, while on the other hand the polyelectrolyte shells of the finally formed PEC aggregates shrink to a smaller one.
Dautzenberg and Kriz (Citation2003) evaluated the influence of addition of salts (LiCl, NaCl, and KCl) on the size of PECs between PDADMAC and acrylamide complexed by polymethacrylate. A constant mixing ratio of X = 0.6 was used. Using salt addition, after complexation, there was an increase in PEC size from around 70 nm to at CNaCl = 0 up to a sharp increase up to 370 nm at around CNaCl = ∼0.5 M and decrease to again around 80 nm at CNaCl > 0.5 M. The other salts also followed the similar trends, which were attributed in terms of salt-induced aggregation of small soluble complexes to larger aggregates at salt concentrations of 0–0.5 M, whereas the sharp drop in particle size of PECs for Csalt > 0.5 M was attributed to the dissolution of PEC aggregates to small soluble complexes or partly to the polyelectrolyte components.
Characterization of PECs
The key parameters to be examined and the techniques used for the characterization of PECs are briefly discussed in this section. The PEC characterization mainly includes the physicochemical, morphological analysis of PEC particles in dispersion, and solid state characterization of the lyophilized complexes.
Physicochemical characterization
Static or dynamic light scattering (SLS/DLS) is the most widely used technique for the estimation of size or size distribution (polydispersity index) of PEC particles. Stokes–Einstein equation is employed to determine the mean hydrodynamic diameter of the PEC particles in these techniques (Delair Citation2011). SLS measures time-average intensities (mean square fluctuations). SLS is also commonly utilized to determine the weight–average molecular weight and hydrodynamic diameter of particles in the sub-micron and supra-micro ranges. DLS (also known as photon correlation spectroscopy or quasi-elastic light scattering) measures the time-dependent fluctuations of the intensity of scattered light caused by particle movement. This fluctuation is due to the fact that the small molecules in solutions undergoing Brownian motion, and so the distance between the scatterers in the solution is constantly changing with time. SLS/DLS covers a range from a few nanometers to about 3 μm (Pardeshi et al. Citation2012). However, SLS/DLS is not able to detect larger microparticles. One can draw the predictions regarding storage stability of colloidal PEC particles from zeta potential measurements using SLS or DLS (Pardeshi et al. Citation2012).
Morphological characterization
The morphological analysis of the colloidal PEC particles can be done using scanning or transmission electron microscopy (SEM/TEM) or using scanning force microscopy (SFM). It has been noted, by various investigators, that PECs are generally polydisperse quasi-spherical particles, sometimes affected by the vacuum drying during SEM analysis (Delair Citation2011, Wu and Delair Citation2015).
Solid state characterization
The FTIR spectroscopy is used to assess the complex formation process. The formation of the new complex was evidenced by the appearance of new bands, change in intensity of already existing bands or the shift of bands from their original position (Delair Citation2011).
The alterations in the thermal properties, through DSC and TGA analysis, are also a mean of characterization of PEC particles as revealed by the Sarmento et al. (Citation2006b). The thermal stability and the effect of process parameters like pH on the properties of PEC particles can be studied using DSC/TGA analysis (Martins et al. Citation2011a, Citation2011b).
The X-ray powder diffraction (XRD) analysis can be used to estimate the nature and degree of crystallinity of the PEC particles, as a consequence of change in pH or electrostatic interactions among the charged functional groups of participating polymers in PECs (Martins et al. Citation2011b).
The qualitative structure of the PEC particles can be evaluated using nuclear magnetic resonance (NMR) spectroscopy. The selectivity offered by the chemical shifts compliments the sensitivity to molecular mobility so as to provide information on the physicochemical status of the PEC particles (Martins et al. Citation2011a).
Complex stoichiometry
The PEC formation process depends on charge neutralization, and the end point of titration can be determined by using various techniques, namely viscosimetry, potentiometry, or conductometry. These methods cannot give an idea regarding the composition of the PECs that can be obtained using elemental analysis, solid state NMR spectroscopy, and X-ray photocorrelation spectroscopy (Delair Citation2011).
Applications of PECs
Applications of PECs in drug delivery
The PEC particles find wide applications in various disease conditions, including cancer (Anitha et al. Citation2011, Martins et al. Citation2013, Wang et al. Citation2014), HIV/AIDS (Costalat et al. Citation2014, Polexe et al. Citation2013), gastric ulcer (Volodko et al. Citation2013), Staphylococci infections (Lankalapalli et al. Citation2014), and fungal infections (Lefnaoui and Moulai-Mostefa 2014). The particulars of polycations and polyanions used, therapeutic agents loaded into, and the diseases targeted using these PECs are presented in .
Table 2. Applications of PECs as drug delivery carriers.
Applications of PECs in gene delivery
The design of biocompatible PECs is a promising strategy for in vivo delivery of non-viral vectors loaded with therapeutic genes to find applications in gene therapy. The condensation of DNA by polycations, leading to the formation of so-called polyplexes, received considerable attention for its potential in gene delivery applications, where the development of safe and effective non-viral vectors remain a central challenge (Amaduzzi et al. Citation2014). The selected examples of PECs utilized as gene delivery carriers are presented in .
Table 3. Selected examples of PECs in gene delivery applications.
Applications of PECs in protein and peptide delivery
Several laboratories have reported the possible applications of PECs in the delivery of therapeutic proteins or peptides. The non-covalent association between positively charged polymer and negatively charged protein or peptide to yield a PEC is based on the principle of electrostatic or hydrophobic interactions in between participating polymers and protein/peptide (Cheng et al. Citation2010). shows the selected examples of PECs investigated for protein and peptide delivery.
Table 4. Applications of PECs in protein and peptide delivery.
Applications of PECs in vaccine delivery
Micro- or nanoparticulate formulations provide a right mode for the delivery of antigenic molecules. In addition to the improved protection, prolonged residence at the desired site, and facilitated transport of the antigen, particulate formulations could also offer more effective antigen recognition by immune cells (Csaba et al. Citation2009, Xiang et al. Citation2006).
Recently, Verheul et al. (Citation2011) developed covalently stabilized polyelectrolyte nanocomplexes composed of thiolated trimethyl chitosan (TMC-SH) and thiolated hyaluronic acid (HA-SH), with or without PEG coating, as a potential nasal and intradermal vaccine delivery system. The PEC particles were loaded with ovalbumin (OVA) as a model antigen. OVA-loaded stabilized TMC-S-S-HA particles demonstrated superior adjuvanticity and immunogenicity compared to non-stabilized particles, after both nasal and intradermal vaccination. PEGylation abolished the beneficial effects of stabilization after nasal vaccination while having similar immunogenicity as stabilized particles after intradermal vaccination. Finally, the authors concluded that stabilization of TMC-HA PEC particles greatly enhance the immunogenicity of OVA after nasal and intradermal vaccination.
Applications of PECs in tissue engineering
The biodegradable or biocompatible materials have been used as support matrices for delivery of bioactive species to target specific tissues and to promote three dimensional tissue reconstructions (Liu et al. Citation2007). There are a number of reports explaining the potential of PECs in various areas of tissue engineering like bone tissue engineering (Coimbra et al. Citation2011a), cartilage tissue engineering (Whu et al. Citation2013), cardiac tissue engineering (Ceccaldi et al. Citation2014), and dental tissue engineering (Chang et al. Citation2014, Coimbra et al. Citation2011b).
Diagnostic and imaging applications of PECs
Huang et al. (Citation2008) developed PECs capable of MRI contrast enhancement using gadolinium (Gd). PECs were 300 nm in diameter, produced by complex coacervation of chitosan with dextran sulfate. Gd was incorporated by two different mechanisms, namely First, diethylenetriamine pentaacetic acid (DTPA) with chelated Gd was grafted to chitosan before complexation with dextran sulfate. Second, Gd was incorporated into PECs by mixing them with gadolinium chloride (GdCl3), which resulted in ionic trapping of Gd ions within the PECs. In this way, three contrast enhanced PECs were produced: (i) Gd-DTPA grafted to chitosan, then complexed with dextran sulfate; (ii) ionically trapped Gd (Gd3+) in preformed PECs; and (iii) a combination including Gd-DTPA grafted to chitosan and ionically trapped Gd. To their conclusions, PECs combining grafted Gd-DTPA and ionically trapped Gd produced significant contrast enhancement in vivo. Particles were observed to accumulate in the rat kidneys. PECs modified using these techniques offer MRI contrast enhancement of a widely used drug and gene delivery vehicles as a method of quantifying particle biodistribution and pharmacokinetics.
Patents pertaining to PECs
The limitation of large-scale commercial production of PECs restricted their transfer from the laboratory to the commercial market. Only few patents have been transferred into the commercial marketed products. Some of the patents relevant to PECs are briefly outlined in .
Table 5. Patents pertinent to PECs.
Conclusions and future perspectives
PECs, being stable, biodegradable, ad biocompatible formulations, are capable of merging the unique properties of different polymers without losing their inherent characteristics. The particle formation process can be considered as an environment friendly, energy-efficient production process utilizing only aqueous solvents and can be carried out at ambient temperature conditions. Because of their versatility and above mentioned merits, PECs find utility as a subject of keen interest for the researchers.
A pharmaceutical formulation if, along with academic researchers, well accepted by the pharmaceutical industries for commercialization and clinical applications, would be considered as an effective therapeutic formulation. Since, PECs have already been proven their potential in pharmaceutical, biomedical, and other allied fields; they must engage a sizeable place in the pharmaceutical market.
Declaration of interest
The authors report no conflicts of interest. The authors alone are responsible for the content and writing of this article.
References
- Amaduzzi F, Bomboi F, Bonincontro A, Bordi F, Casciardi S, Chronopoulou L, et al. 2014. Chitosan–DNA complexes: charge inversion and DNA condensation. Colloids Surf B Biointerfaces. 114:1–10.
- Anitha A, Deepagan VG, Divya Rani VV, Menon D, Nair SV, Jayakumar R. 2011. Preparation, characterization, in vitro drug release and biological studies of curcumin loaded dextran sulphate–chitosan nanoparticles. Carbohydr Polym. 84:1158–1164.
- Ankerfors C, Ondaral S, Wagberg L, Odberg L. 2010. Using jet mixing to prepare polyelectrolyte complexes: complex properties and their interaction with silicon oxide surfaces. J Colloid Interface Sci. 351:88–95.
- Berger J, Reist M, Mayer JM, Felt O, Gurny R. 2004. Structure and interactions in chitosan hydrogels formed by complexation or aggregation for biomedical applications. Eur J Pharm Biopharm. 57:35–52.
- Boeris V, Farruggia B, Nerlia B, Romanini D, Pico G. 2007. Protein-flexible chain polymer interactions to explain protein partition in aqueous two-phase systems and the protein-polyelectrolyte complex formation. Int J Biol Macromol. 41:286–294.
- Borbely J, Hajdu I, Bodnar M, Denyicska IS. Novel targeted paramagnetic contrast agent. US Patent 2013; US 2013/0302255 A1.
- Bui L, Abbou S, Ibarboure E, Guidolin N, Staedel C, Toulme JJ, et al. 2012. Encapsidation of RNA polyelectrolyte complexes with amphiphilic block copolymers: toward a new self-assembly route. J Am Chem Soc. 134:20189–20196.
- Ceccaldi C, Bushkalova R, Alfarano C, Lairez O, Calise D, Bourin P, et al. 2014. Evaluation of polyelectrolyte complex-based scaffolds for mesenchymal stem cell therapy in cardiac ischemia treatment. Acta Biomater. 10:901–911.
- Cegnar M, Miklavzin A, Kerc J. 2011. Freeze-drying and release characteristics of polyelectrolyte nanocarriers for the mucosal delivery of ovalbumin. Acta Chim Slov. 58:241–250.
- Chang HH, Wang YL, Chiang YC, Chen YL, Chuang YH, Tsai SJ, et al. 2014. A novel chitosan-γ PGA polyelectrolyte complex hydrogel promotes early new bone formation in the alveolar socket following tooth extraction. PLoS One. 9:e92362.
- Cheng WP, Thompson C, Ryan SM, Aguirre T, Tetley L, Brayden DJ. 2010. In vitro and in vivo characterisation of a novel peptide delivery system: amphiphilic polyelectrolyte–salmon calcitonin nanocomplexes. J Control Release. 147:289–297.
- Cifani N, Chronopoulou L, Pompili B, Martino AD, Bordi F, Sennato S, et al. 2015. Improved stability and efficacy of chitosan/pDNA complexes for gene delivery. Biotechnol Lett. 37:557–565.
- Coimbra P, Alves P, Valente TA, Santos R, Correia IJ, Ferreira P. 2011a. Sodium hyaluronate/chitosan polyelectrolyte complex scaffolds for dental pulp regeneration: synthesis and characterization. Int J Biol Macromol. 49:573–579.
- Coimbra P, Ferreira P, de Sousa HC, Batista P, Rodrigues MA, Correia IJ, et al. 2011b. Preparation and chemical and biological characterization of a pectin/chitosan polyelectrolyte complex scaffold for possible bone tissue engineering applications. Int J Biol Macromol. 48:112–118.
- Costalat M, Alcouffe P, David L, Delair T. 2014. Controlling the complexation of polysaccharides into multi-functional colloidal assemblies for nanomedicine. J Colloid Interface Sci. 430:147–156.
- Csaba N, Garcia-Fuentes M, Alonso MJ. 2009. Nanoparticles for nasal vaccination. Adv Drug Deliv Rev. 61:140–157.
- Dautzenberg H. 1997. Polyelectrolyte complex formation in highly aggregating systems. 1. Effect of salt: polyelectrolyte complex formation in the presence of NaCl. Macromolecules. 30:7810–7815.
- Dautzenberg H, Kriz J. 2003. Response of polyelectrolyte complexes to subsequent addition of salts with different cations. Langmuir. 19:5204–5211.
- Dautzenberg H, Linow KJ, Philipp B. 1982. Anionic to form water-soluble polysalts (symplexes) and cationic copolymers of acrylamide. Acta Polymerica. 33:619–623.
- Delair T. 2011. Colloidal polyelectrolyte complexes of chitosan and dextran sulfate towards versatile nanocarriers of bioactive molecules. Eur J Pharm Biopharm. 78:10–18.
- Fukuda H, Kikuchi Y. 1977. Polyelectrolyte complexes of sodium dextran sulfate with chitosan. Macromol Chem Phys. 178:2895.
- Giannotti MI, Sanz CF, Schwartz NS. Polyelectrolyte complex, process for its manufacturing and use thereof. World Patent 2012; WO 2012085888 A2.
- Goncalves RM, Antunes JC, Barbosa MA. 2012. Mesenchymal stem cell recruitment by stromal derived factor-1-delivery systems based on chitosan/poly(γ-glutamic acid) polyelectrolyte complexes. Eur Cell Mater. 23:249–261.
- Gucht J, Spruijt E, Lemmers M, Stuart MAC. 2011. Polyelectrolyte complexes: bulk phases and colloidal systems. J Colloid Interface Sci. 361:407–422.
- Ho YC, Mi FL, Sung HW, Kuo PL. 2009. Heparin-functionalized chitosan-alginate scaffolds for controlled release of growth factor. Int J Pharm. 376:69–75.
- Hu Y, Yang T, Hu X. 2012. Novel polysaccharides-based nanoparticle carriers prepared by polyelectrolyte complexation for protein drug delivery. Polym Bull. 68:1183–1199.
- Huang M, Berkland C. 2008. Controlled release of repifermin1 from polyelectrolyte complexes stimulates endothelial cell proliferation. J Pharm Sci. 98:268–280.
- Huang M, Huang ZL, Bilgen M, Berkland C. 2008. Magnetic resonance imaging of contrast-enhanced polyelectrolyte complexes. Nanomedicine. 4:30–40.
- Huang M, Vitharana SN, Peek LJ, Coop T, Berkland C. 2007. Polyelectrolyte complexes stabilize and controllably release vascular endothelial growth factor. Biomacromolecules. 8:1607–1614.
- Jean M, Smaoui F, Lavertu M, Methot S, Bouhdoud L, Buschmann MD, et al. 2009. Chitosan-plasmid nanoparticle formulations for IM and SC delivery of recombine FGF-2 and PDGF-BB or generation of antibodies. Gene Ther. 16:1097–1110.
- Jiang R, Lu X, Yang M, Deng W, Fan Q, Huang W. 2013. Monodispersed brush-like conjugated polyelectrolyte nanoparticles with efficient and visualized siRNA delivery for gene silencing. Biomacromolecules. 14:3643–3652.
- Johnson JH. Heparin polyelectrolyte polymer complex. US Patent 1984; 4471112.
- Kabanov AV, Zezin AB. 1984. Soluble interpolymeric complexes as a new class of synthetic polyelectrolytes. Pure Appl Chem. 56:343–354.
- Kim SH, Lee SH, Tian H, Chen X, Park TG. 2009. Prostate cancer cell-specific VEGF siRNA delivery system using cell targeting peptide conjugated polyplexes. J Drug Target. 17:311–317.
- Koetz J, Kosmella S. 2007. Polyelectrolytes and Nanoparticles. Berlin: Springer.
- Lankalapalli S, Kolapalli VRM. 2009. Polyelectrolyte complexes: a review of their applicability in drug delivery technology. Indian J Pharm Sci. 5:481–487.
- Lankalapalli S, Routhu KC, Tenneti VSVK. 2014. Preparation and evaluation of vancomycin polyelectrolyte complex nanoparticles. Indian J Nanosci. 2:10–18.
- Laue C, Kauper P. Chitosan based particles. US Patent 2008; US2008/0254078A1.
- Laue C, Kauper P. Hydrophilic particles based on cationic chitosan derivatives. World Patent 2006; WO 2006064331 A1.
- Lee D, Kim D, Mok H, Jeong JH, Choi D, Kim SH. 2012. Bioreducible crosslinked polyelectrolyte complexes for MMP-2 siRNA delivery into human vascular smooth muscle cells. Pharm Res. 29:2213–2224.
- Lefnaoui S, Moulai-Mostefa N. 2015. Polyelectrolyte complex based on carboxymethyl-kappa-carrageenan and Eudragit RL 30D as prospective carriers for sustained drug delivery. Chem Eng Res Des. 97:165–174.
- Liu C, Xia Z, Czernuszka JT. 2007. Design and development of three-dimensional scaffolds for tissue engineering. Chem Eng Res Des. 85:1051–1064.
- Liu W, Sun S, Cao Z, Zhang X, Yao K, Lu WW, et al. 2005. An investigation on the physicochemical properties of chitosan/DNA polyelectrolyte complexes. Biomaterials. 26:2705–2711.
- Luppi B, Bigucci F, Mercolini L, Musenga A, Sorrenti M, Catenacci L, et al. 2009. Novel mucoadhesive nasal inserts based on chitosan/hyaluronate polyelectrolyte complexes for peptide and protein delivery. J Pharm Pharmacol. 61:151–157.
- Mao S, Bakowsky U, Jintapattanakit A, Kissel T. 2006. Self-assembled polyelectrolyte nanocomplexes between chitosan derivatives and insulin. J Pharm Sci. 95:1035–1048.
- Martins AF, Bueno PVA, Almeida EAMS, Rodrigues FHA, Rubira AF, Muniz EC. 2013. Characterization of N-trimethyl chitosan/alginate complexes and curcumin release. Int J Biol Macromol. 57:174–184.
- Martins AF, Pereira AGB, Fajardo AR, Rubira AF, Muniz EC. 2011b. Characterization of polyelectrolytes complexes based on N,N,N-trimethyl chitosan/heparin prepared at different pH conditions. Carbohydr Polym. 86:1266–1272.
- Martins AF, Piai JF, Schuquel ITA, Rubira AF, Muniz EC. 2011a. Polyelectrolyte complexes of chitosan/heparin and N,N,N-trimethyl chitosan/heparin obtained at different pH: I. Preparation, characterization, and controlled release of heparin. Colloid Polym Sci. 289:1133–1144.
- Mende M, Buchhammer HM, Schwarz S, Petzold G, Jaeger W. 2004. The stability of polyelectrolyte complex systems of PDADMAC with different polyanions. Macromol Symp. 211:121–133.
- Michaels AS, Miekka RG. 1961. Polycation-polyanion complexes: preparation and properties of poly-(vinylbenzyltrimethylammonium) poly-(styrenesulfonate). J Phys Chem. 65:1765–1773.
- Muller M. 2014. Sizing, shaping and pharmaceutical applications of polyelectrolyte complex nanoparticles. Adv Polym Sci. 256:197–260.
- Muller M, Keßler B, Frohlich J, Poeschla S, Torgeret B. 2011. Polyelctrolyte complex nanoparticles of poly(ethyleneimine) and poly(acrylic acid): preparation and applications. Polymers. 3:762–778.
- Pardeshi CV, Rajput PV, Belgamwar VS, Tekade AR, Patil GB, Chaudhary KS, et al. 2012. Solid lipid based nanocarriers: an overview. Acta Pharm. 62:433–472.
- Pergushov DV, Muller AHE, Schacher FH. 2012. Micellar interpolyelectrolyte complexes. Chem Soc Rev. 41:6888–6901.
- Philipp B, Dautzenberg H, Linow KJ, Kotz J, Dawydoff W. 1989. Polyelectrolyte complexes – recent developments and open problems. Prog Polym Sci. 14:91–172.
- Polexe RC, Terrat C, Verrier B, Cuvilier A, Champier G, Delair T. 2013. Elaboration of targeted nanodelivery systems based on colloidal polyelectrolyte complexes (PEC) of chitosan (CH)-dextran sulphate (DS). Eur J Nanomed. 5:39–49.
- Prokop A. Micro-particulate and nano-particulate polymeric delivery system. US Patent 2003; US 2003/0170313A1.
- Robertis SD, Bonferoni MC, Elviri L, Sandri G, Caramella C, Bettini R. 2015. Advances in oral controlled drug delivery: the role of drug–polymer and interpolymer non-covalent interactions. Expert Opin Drug Deliv. 12:441–453.
- Sæther HV, Holme HK, Maurstad G, Smidsrod O, Stokke BT. 2008. Polyelectrolyte complex formation using alginate and chitosan. Carbohydr Polym. 74:813–821.
- Sajeesh S, Sharma CP. 2007. Novel polyelectrolyte complexes based on poly(methacrylic acid)-bis(2-aminopropyl)poly(ethylene glycol) for oral protein delivery. J Biomater Sci Polym Ed. 18:1125–1139.
- Sarmento B, Martins S, Ribeiro A, Veiga F, Neufeld R, Ferreira D. 2006a. Development and comparison of different nanoparticulate polyelectrolyte complexes as insulin carriers. Int J Pept Res Ther. 12:131–138.
- Sarmento B, Ribeiro A, Veiga F, Ferreira D. 2006b. Development and characterization of new insulin containing polysaccharide nanoparticles. Coll Surf B Biointerfaces. 53:193–202.
- Schatz C, Domard A, Viton C, Pichot C, Delair T. 2004b. Versatile and efficient formation of colloids of biopolymer-based polyelectrolyte complexes. Biomacromol. 5:1882–1892.
- Schatz C, Lucas J, Viton C, Domard A, Pichot C, Delair T. 2004a. Formation and properties of positively charged colloids based on polyelectrolyte complexes of biopolymers. Langmuir. 20:7766–7778.
- Scheuing D, Lestage DJ, Bennett CW, Knock MM, Scales CW, Smith WL, Zhang R. Polyelectrolyte complexes. US Patent 2013; US 2013/0273174 A1.
- Sun W, Mao S, Mei D, Kissel T. 2008. Self-assembled polyelectrolyte nanocomplexes between chitosan derivatives and enoxaparin. Eur J Pharm Biopharm. 69:417–425.
- Toreki W, Moore D. Method of attaching an antimicrobial cationic polyelectrolyte to the surface of substrate. US Patent 2012; US 8,092,854 B2.
- Tsuchida E. 1994. Formation of polyelectrolyte complexes and their structures. J Macromol Sci Pure Appl Chem. 31:1–15.
- Verheul RJ, Slutter B, Bal SM, Bouwstra JA, Jiskoot W, Hennink WE. 2011. Covalently stabilized trimethyl chitosan-hyaluronic acid nanoparticles for nasal and intradermal vaccination. J Control Release. 156:46–52.
- Volodko AV, Davydova VN, Chusovitin EA, Sorokina IV, Dolgikh MP, Tolstikova TG, et al. 2013. Soluble chitosan-carrageenan polyelectrolyte complexes and their gastroprotective activity. Carbohydr Polym. 101:1087–1093.
- Wang J, Ni C, Zhang Y, Zhang M, Li W, Yao B, et al. 2014. Preparation and pH controlled release of polyelectrolyte complex of poly(l-malic acid-co-d,l-lactic acid) and chitosan. Colloids Surf B Biointerfaces. 115:275–279.
- Whu SW, Hung KC, Hsieh KH, Chen CH, Tsai CL, Hsu SH. 2013. In vitro and in vivo evaluation of chitosan-gelatin scaffolds for cartilage tissue engineering. Mater Sci Eng C Mater Biol Appl. 33:2855–2863.
- Wu D, Delair T. 2015. Stabilization of chitosan/hyaluronan colloidal polyelectrolyte complexes in physiological conditions. Carbohydr Polym. 119:149–158.
- Wu QX, Zhang QL, Lin DQ, Yao SJ. 2013. Characterization of novel lactoferrin loaded capsules prepared with polyelectrolyte complexes. Int J Pharm. 455:124–131.
- Xiang SD, Scholzen A, Minigo G, David C, Apostolopoulos V, Mottram PL, et al. 2006. Pathogen recognition and development of particulate vaccines: does size matter? Methods. 40:1–9.
- Ying JY, Wan ACA. Cell-adhesive polyelectrolyte material for use as membrane and coating. World Patent 2007; WO 2007108775 A1.
- Ying JY, Wan ACA. Cell-adhesive polyelectrolyte material for use as membrane and coating. US Patent 2010; US 2010/0111917 A1.
- Zaitsev S, Cartier R, Vyborov O, Sukhorukov G, Paulke BR, Haberland A, et al. 2004. Polyelectrolyte nanoparticles mediate vascular gene delivery. Pharm Res. 21:1656–1661.
- Zezin AB, Kabanov VA. 1982. A new class of complex water-soluble polyelectrolytes. Russ Chem Rev. 51:833–855.