Abstract
Curcumin is an effective and safe anticancer agent, and also known to induce vasodilation, but its hydrophobicity limits its clinical application. In this study, a simple emulsion method was developed to prepare biodegradable poly (ethylene glycol)–poly (lactic acid) (PEG–PLA) nanospheres to encapsulate curcumin to improve its solubility and stability. The nanoparticle size was around 150 nm with a narrow size distribution. Fluorescence microscopy showed that curcumin encapsulated PEG-PLA nanospheres were taken up rapidly by Hela and MDA-MB-231 cancer cells. This novel nanoparticulate carrier may improve the bioavailability of curcumin without affecting its anticancer properties.
Introduction
Curcumin is a low-molecular-weight polyphenol derived from the root of turmeric, a plant from southeast tropical Asia. Turmeric has been extensively used in kitchen as a spice and coloring agent, as well as a therapeutic agent in traditional Indian medicine (Aggarwal et al. Citation2007, Aggarwal and Sung Citation2009). Curcumin is believed to be the main bioactive component isolated from the turmeric rhizome. Recent studies indicate that curcumin possesses antioxidant, anti-inflammatory, wound healing, antimicrobial properties, and suppresses proliferation of a wide variety of tumor cells (Aggarwal et al. Citation2003, Egan et al. Citation2004, Goel et al. Citation2008, Krausz et al. Citation2015, Narayanan et al. Citation2014, Somasundaram et al. Citation2002). Some studies suggested that curcumin is a universal anticancer agent, suppressing many types of cancer, such as bladder, ovary, colon, and breast cancer (Gao et al. Citation2013, Mirakabad et al. Citation2016, Shi et al. Citation2006, Tian et al. Citation2008). Several phase-I and phase-II clinical trials indicate that curcumin may exhibit therapeutic efficacy (Cheng et al. Citation2001, Dhillon et al. Citation2008, Sharma et al. Citation2004). Very recent studies also indicated the vasodilatory influence of curcumin (Dewar et al. Citation2011, Fang et al. Citation2009, Nurullahoglu-Atalik et al. Citation2012, Rungseesantivanon et al. Citation2010). In diabetic rat models, curcumin reduced the baseline mean arterial pressure and enhanced acetylcholine-induced vasodilation as well as cilostazol-induced vasodilation (Nurullahoglu-Atalik et al. Citation2012, Rungseesantivanon et al. Citation2010). Moreover, animal or human studies have not found any toxicity associated with curcumin even when used at high doses (Lao et al. Citation2006). Despite its efficacy and safety, the clinical application of curcumin is diminished by its poor bioavailability due to its extremely low solubility in water and rapid degradation at alkaline pH (Anand et al. Citation2007, Kurien et al. Citation2007, Tonnesen and Karlsen Citation1985).
To improve the solubility and bioavailability of curcumin and increase its therapeutic efficacy, researches have concentrated on the development of alternative delivery systems. Several formulations have been developed, such as liposomes, polymer microparticles and nanoparticles, cyclodextrin, and hydrogels (Anitha et al. Citation2014, Bansal et al. Citation2011, Dhule et al. Citation2012, Kumar et al. Citation2014, Tiyaboonchai et al. Citation2007). Among these approaches, biodegradable polymeric nanoparticles offer significant advantage owing to their great biocompatibility, better encapsulation, control release, and less toxic properties. More importantly, there is tremendous versatility in the choice of polymer matrices that can be used for tailoring nanoparticle properties to meet various drug delivery needs.
Poly-(lactic acid) (PLA) is the most widely used polymer in drug delivery systems and has been approved by FDA for clinical use. However, the hydrophobicity of PLA raises concerns for further biological and biomedical applications. Polyethylene glycol (PEG) is the most popular hydrophilic polymer for surface modification of nanoparticulate drug carriers and has been used to modify hydrophobic PLA to form amphiphilic copolymer PEG–PLA. PEG has prolonged blood circulation and diminished reticuloendothelial system uptake and is also FDA approved. The biocompatible copolymer PEG–PLA has been extensively investigated as drug delivery vehicles (Gao et al. Citation2013, Vila et al. Citation2004, Xia et al. Citation2012). Some investigators have used PEG–PLA to encapsulate curcumin to improve its solubility and sustained release (Gao X et al. Citation2013, Song et al. Citation2013, Thu et al. Citation2013). Gref et al. evaluated PLA nanoparticles modified with different PEG molecular weight (Mw, 2K–20 Kg/mol) and found the maximal reduction of protein absorption with PEG Mw of 5 Kg/mol (Gref et al. Citation2000). In the present study, a simple emulsion method with a presolidification step was developed to encapsulate curcumin into PEG5K–PLA50K nanospheres. Curcumin was successfully loaded into the PEG–PLA nanospheres and delivered to HeLa and MDA-MB-231 cancer cells.
Materials and methods
Materials
Curcumin (95% curcuminoid content), polyvinyl alcohol (PVA; 86–89% hydrolyzed, average molecular weight, 57–66 k), and thiazolyl blue tetrazolium bromide (98%) were purchased from Alfa Aesar (Ward Hill, MA). PEG–PLA polymer with a PEG and PLA molecular weight (Mw) of 5 kDa and 50 kDa, respectively, was customer synthesized by Shandong Institute of Medical Instruments, China. Acetone, methanol, DMSO, 1-propanol and ethyl acetate (EA) were purchased from Sigma-Aldrich (St Louis, MO).
Cell lines
HeLa cells (a cervical cancer cell line) were generously provided by Dr. David Sharp (Albert Einstein College of Medicine, Bronx, NY). MDA-MB-231 cells (a breast cancer cell line) were generously provided by Dr. Anne Bresnick (Albert Einstein College of Medicine, Bronx, NY). These cells were maintained in DMEM medium (Gibco, Life technologies, Grand Island, NY) supplemented with 10% fetal bovine serum (Gibco Sera, Life technologies), 1% penicillin–streptomycin (Corning Cellgro, Manassas, VA), and 1% GlutaMax (100x) (Gibco, Life technologies, Grand Island, NY) at 37 °C in a humidified atmosphere (5% CO2).
Preparation of curcumin-loaded PEG–PLA nanospheres (Curc-NS)
Curc-NS were prepared by an oil/water (o/w) simple emulsion method with a preliminary solidification step. Briefly, 50 mg of PEG–PLA polymer and 5 mg curcumin were codissolved in 1 mL EA and then added into 2 mL of 1% PVA in water (w/v) solution. The o/w emulsion was prepared by tip sonication (Sonic Dismembrator 60, Fisher Scientific, Waltham, MA) at 15 W for 30 s in an ice bath. Afterwards, the resulting o/w emulsion was poured quickly into 10 mL of 1% PVA solution and stirred at 100 rpm to preliminarily solidify the emulsion droplets. After 4 min, the suspension containing embryonic particles was poured into 200 mL water under magnetic stirring at 400 rpm to completely solidify the embryonic particles. Finally, the nanospheres were isolated by centrifugation (Thermo Scientific, SORVALL RC 6 + Centrifuge, Thermo Scientific, Waltham, MA) at 22,000 g for 30 min and washed three times with water. The blank PEG–PLA nanospheres (Control-NS) were prepared without adding curcumin.
Determination of nanoparticle size and surface morphology
Dynamic light scattering (DLS) measurements for determining the average size and size distribution of the nanospheres were performed with a ZetaPlus/Zeta potential analyzer (Brookhaven Instruments Corporation, Holtsville, NY). Size, shape and the surface morphology of nanospheres were examined using field emission scanning electron microscope (SEM, Zeiss Supra 40, Jena, Germany). Particle size was measured with ImageJ software (Bethesda, MD). The mean diameter was calculated based on the measurements of 100 randomly chosen particles.
Determination of curcumin loading and encapsulation efficiency
Lyophilized Curc-NS (5 mg) were dissolved in 2 mL acetone to extract curcumin for the drug loading and encapsulation estimations. The samples in acetone were first gently shaken on a shaker for 30 min at room temperature and then centrifuged at 14,000 rpm (Eppendorf Centrifuge 5418, Hamberg, Germany) for 3 min. The clear supernatant was collected. Curcumin concentration in the supernatant was quantified with a UV-Vis spectrophotometer (Evolution 220, Thermo Scientific, Waltham, MA) at a wavelength of 420 nm. A standard plot of curcumin (0.1–10 mg/L) was prepared under identical conditions. The curcumin drug loading (DL) content and encapsulation efficiency (EE) were calculated as follows:
Stability of free curcumin
The stability of free curcumin in 10 mM phosphate-buffered saline (PBS) solution, pH 7.4 was measured based on the methodology of Ma et al. with slight modification (Ma et al. Citation2008). Curcumin was dissolved in PBS at 10 μg/mL with methanol (final methanol concentration is ≤5% v/v). Aliquots (1 mL) of curcumin in PBS were incubated at 37 °C, shaking gently at 130 rpm. At predetermined time points, three tubes were taken out, centrifuged at 14,000 rpm and the remained amounts of curcumin in the solution were quantified with a UV-Vis spectrophotometer.
In vitro release of curcumin from Curc-NS
Curc-NS were dispersed in PBS at a final curcumin concentration around 10 μg/mL. Aliquots (1 mL) of this sample were incubated at 37 °C, shaking gently at 130 rpm. At predetermined time intervals, three tubes were taken out and centrifuged to collect the particles. The remained amounts of curcumin in the particles were first extracted in acetone and then quantified spectrophotometrically.
The release was quantified as follows:
Cellular uptake studies
MDA-MB-231 and HeLa cells were grown in 35-mm culture plates at a seeding density of 5 × 104 cells per well in 2 mL of growth medium. After incubation at 37 °C for 24 h, the cells were treated with 30 μM Curc-NS and Control-NS. After 2 h, the treated cells were examined under an inverted fluorescence microscope (Nikon ECLIPSE, TE2000, Melville, NY) to confirm intracellular localization of encapsulated curcumin. Fluorescence photographs were taken with a charge-coupled device camera under green channel.
Cytotoxic studies
MDA-MB-231 and HeLa cells were seeded at a seeding density of 5 × 104 per well/1 mL medium in 12-well flat-bottom plates. After 24 h, the cultured cells were treated with different concentrations of Curc-NS and free curcumin (dissolved in DMSO, the final DMSO concentration < 0.1%). Medium with 0.1% DMSO and Control-NS were used as controls. After 48 h, the antiproliferative effect was determined using MTT (thiazolyl blue tetrazolium bromide) dye assay. In brief, MTT (1 mg/mL) was added to each well and the plates were incubated at 37 °C for 1 h. Then, MTT medium was removed and 800 μL of 1-propanol was added to each well. The color intensity was measured at 560 nm using a microplate spectrophotometer (Epoch, BioTek Instruments Inc., Winooski, VT). The antiproliferative effects of free curcumin and Curc-NS were calculated as a percentage of cell growth with respect to the DMSO and Control-NS. Just before adding MTT reagents, representative phase contrast microscope images of cells were taken using a microscope (Nikon ECLIPSE, TE2000, Metville, NY).
Results
Physiochemical characterization of nanoparticles
Free curcumin is poorly soluble in aqueous solution, about 0.6 μg/mL at neutral pH (Kurien et al. Citation2007). shows the dispersion of curcumin at 1.5 mg/mL in water. Most of the curcumin is not dissolved and settled at the bottom within few minutes. Dispersion of Curc-NS carrying an equal amount of curcumin is displayed in . Curc-NS are well dispersed with the natural yellow color of curcumin. The nanoparticles stayed dispersed for several days without visible changes. This kind of distribution of nanoparticles is desired for the administration of curcumin or any therapeutic drugs into circulation.
Figure 1. Particle size distribution, dispersibility, and morphology of Curc-NS. (A) Free curcumin in water. (B) Curc-NS in water. (C) Dynamic light scattering size measurement. (D) SEM images of Curc-NS, and (E) Magnification of (D).
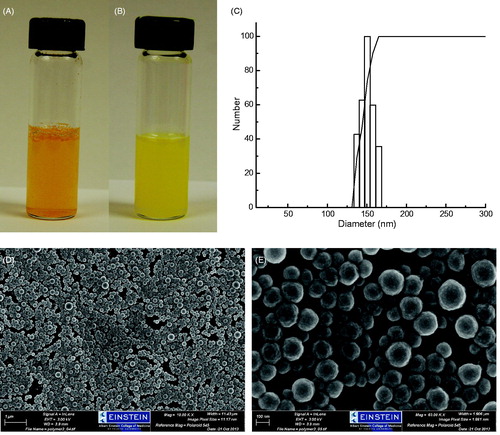
The size distribution of the polymeric nanoparticles was measured by DLS. The results are illustrated in . The average particle diameter is 152 nm at 25 °C with a narrow size distribution. show the SEM images of Curc-NS. The nanoparticles had a rough surface and a spherical shape without the presence of curcumin crystals on the surface. The nanoparticles are homogeneous with an average size of around 147 nm, which is comparable to the size obtained from DLS measurements.
A UV-vis absorbance spectrum of free curcumin (6 μg/mL) in acetone gave a major peak centered at 420 nm (), whereas Control-NS (1 mg/mL) showed no absorbance in the measuring range (). The major absorbance peak for Curc-NS in water was red shifted to 424 nm, with a shoulder on the right side (), indicating that curcumin present in Curc-NS exists in a relatively polar environment. The peak intensity is less than that of free curcumin in acetone, indicating curcumin was encapsulated in the PEG–PLA shells.
Figure 2. UV-vis absorbance spectra of cucumin. (A) Free curcumin in acetone at 6 μg/mL, (B) Curc-NS in water at 15 μg/mL, (C) Control-NS in acetone at 1 mg/mL.
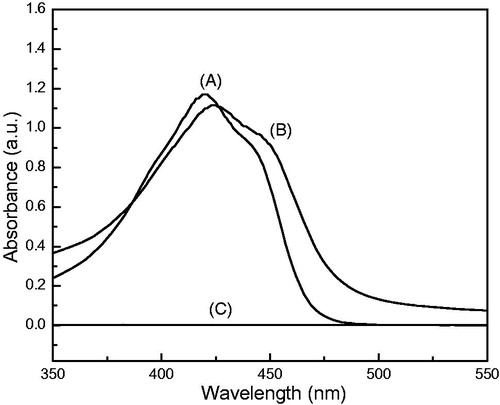
The amount of encapsulated drug in the nanoparticles is an important factor for determining the release profile of a drug delivery system. The entrapped curcumin contents were estimated by UV-vis spectroscopy. Acetone was used as solvent to dissolve the nanoparticles and extract curcumin out. Curcumin shows a strong absorbance peak at 420 nm in acetone, while PEG–PLA shows no absorbance in the measuring range (). Curcumin was efficiently loaded into the nanoparticles with the DL of 8.2% (w/w) and the EE of 56.1%.
In vitro release of curcumin from Curc-NS
Curcumin degrades rapidly in aqueous buffers at physiological pH. Free curcumin dissolved in PBS with methanol (<5%) underwent a rapid degradation; 75.6% of curcumin degraded after incubation for 15 min at 37 °C, and 82.6% of free curcumin decomposed in 30 min ().
Figure 3. Curcumin release profile of Curc-NS. (A) Stability of free curcumin in PBS at 37 °C. (B) Release of curcumin from Curc-NS in PBS at 37 °C. Data are presented as mean ± standard error of experiments performed in triplicate.
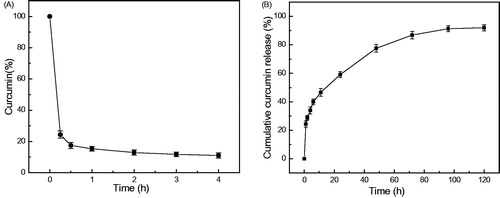
In vitro release of curcumin from Curc-NS into PBS is shown in . A biphasic release profile was observed; an initial burst release on the first day followed by a slow and sustained release for a prolonged time period (120 h). After 1 h and 6 h incubation in PBS, 24.3% and 40.0% of curcumin was released from Curc-NS, respectively. Overall, about 93% curcumin was released in 5 days.
Cellular uptake studies
Cellular uptake and localization of curcumin is an important parameter of its biological activity. Curcumin is a fluorescent molecule with an excitation maximum at 420 nm and emission range 470–520 nm. Therefore, fluorescence microscopy studies were carried out to monitor the cellular uptake of curcumin. displays the microscopic images of fluorescence studies. Both HeLa and MDA-MB-231 cells showed green fluorescence after treating with Curc-NS as seen in . The cells treated with Control-NS did not show any fluorescence ().
Figure 4. Cellular uptake of Curc-NS. Fluorescence microscopic images of HeLa cells treated with (A) Curc-NS, (B) Control-NS, and MDA-MB-231 cells treated with (C) Curc-NS, (D) Control-NS.
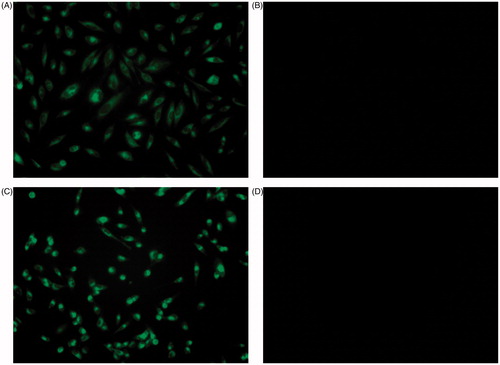
Curc-NS were formulated with an amphiphilic copolymer; therefore, these particles are expected to be preferably attached to the membrane. However, both MDA-MB-231 and HeLa cells show emission from the entire cells. Since the majority of the cell volume is occupied by the nucleus with very little cytoplasm, the emission could be from both the membrane and the nucleus.
Cytotoxic studies
The cytotoxicity of Curc-NS was studied in two human cancer cell lines, HeLa cells and MDA-MB-231 cells. Cells were incubated with different concentrations of free curcumin and Curc-NS for 2 days and then examined by a MTT assay for cell viability.
Curc-NS exhibited cytotoxicity in a dose-dependent manner in both cell lines studied (). As the curcumin concentration in Curc-NS increased from 10 μM to 20 μM, the viable cells number decreased drastically from 80% to 40% in both cell lines; at 40 μM concentration, only 20% of the cells survived (P < 0.05). The results are comparable to that of free curcumin at almost all the doses studied (). These results are in accordance with the microscopic studies, which show an almost equal population of viable cells in both cell lines treated with Curc-NS and free curcumin at curcumin concentration of 30 μM (). Like free curcumin, Curc-NS caused morphological changes in both cell lines. HeLa cells showed elongated shape, while MDA-MB-231 cells showed round shape. Both cells exhibited apoptotic features. In the control studies, cells treated with 0.1% DMSO or Control-NS did not show any effect on cell viability (), indicating that PEG–PLA nanoparticle system is an ideal drug delivery carrier with low toxicity on these cells.
Figure 5. Cytotoxicity of free curcumin and Curc-NS. Cell viability of (A) Hela cells and (B) MDA-MB-231 cells exposed to different concentration of free curcumin (open bars) and Curc-NS (filled bars). Data are presented as mean and standard deviations of six repeats of each treatment group (P < 0.05).
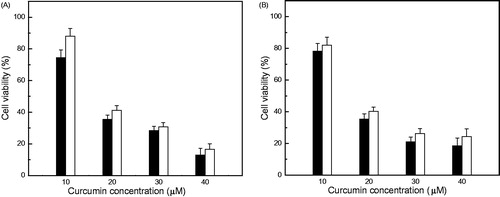
Discussion
Curcumin is recognized as an excellent molecule among many naturally occurring compounds for cancer therapeutics, but its low solubility in water (∼0.6 μg/mL at neutral pH) and extreme sensitivity at physiological pH limit its clinical application (Kurien et al. Citation2007, Tonnesen and Karlsen Citation1985). It is very desirable to develop an aqueous formulation of curcumin to improve its clinical application.
Amphiphilic copolymers are multibeneficial in drug delivery. At one end, they provide a hydrophilic surface that can mask the hydrophobic core and prevent reticuloendothelial uptake, whereas on the other, they provide a hydrophobic core that can be a portal for hydrophobic drugs. In this study, PLA was chosen as the hydrophobic core material and PEG was chosen as the hydrophilic surface material. Curcumin was encapsulated into PEG–PLA nanospheres by a simple emulsion method. First, PEG–PLA was dissolved in EA. Then, an emulsion (o/w) was formed in 1% PVA/water aqueous phase by sonication. PEG is soluble in water but not in EA, while PLA is soluble in EA but not in water. Consequently, PEG migrates from the droplets' inner core to the water interface, and PLA remains inside the droplets during emulsification (Xia et al. Citation2012). As EA diffuses to the outer aqueous phase, nanosphere core solidifies. When an o/w emulsion is directly poured into a large amount of aqueous solution, the fast diffusion of EA from oil droplets to the outer aqueous phase will cause the droplets coalescence, thus some polymer agglomerates formed (Meng et al. Citation2003). In order to overcome the coalescence, Meng et al. introduced a preliminary solidification step to solidify the particles step by step (Meng et al. Citation2003). Here, we adopted this presolidification step. The o/w emulsion was first poured into a small amount of 1% PVA solution to extract EA slowly out of the oil phase, partially solidify the embryonic nanoparticles, and then the suspension was poured into a big amount of water to further remove the residual EA within the semi-solid nanoparticles, thus completely solidify the nanoparticles. As seen from , the obtained Curc-NS were monodisperse with a spherical shape. DLS and SEM results indicated the average particles size is around 150 nm with a narrow size distribution.
In previous reports, some amphiphilic polymer nanoparticles have been used to deliver curcumin (Anand et al. Citation2010, Gao et al. Citation2013, Ma et al. Citation2008, Mohanty et al. Citation2010, Song et al. Citation2013, Thu et al. Citation2013, Yang et al. Citation2014). Curcumin-encapusalted nanoparticles were prepared by simple emulsion, nanoprecipitation, dialysis, and thin-film hydration methods. Dichloromethane, acetone, and acetonitrile were used as organic solvents in these studies. The curcumin drug loading in these nanoparticles ranged from 0.4–8%. In this study, we used EA as organic solvent, which is nontoxic and environment friendly. We also introduced a presolidification step in our simple emulsion method, which slowed down the extraction of EA out of the oil phase, prevented the fast precipitation of curcumin in water phase, and partially solidified nanoparticles further limited the diffusion of curcumin out of the oil phase, thus enabled a better entrapment of hydrophobic drug curcumin. Such obtained Curc-NS are homogeneous with a narrow size distribution at 152 nm, and could be re-dispersed well in water (). Compared with previous reports, our product has a relatively high encapsulation efficiency of 56.1%, and high drug loading of 8.2%. More importantly, our method is very simple and environmentally friendly.
In addition to increasing the water solubility of curcumin, another objective of using PEG–PLA nanospheres is to protect the encapsulated curcumin against hydrolysis. Free curcumin experienced high degradation in PBS as shown in . Such high degradation and instability properties of curcumin in PBS were previously observed by other researchers (Ma et al. Citation2008). The high degradation has been considered another potential limitation for its therapeutic use. The in vitro release study indicated that our Curc-NS could release curcumin slowly into PBS (). It showed a typical biphasic release profile. The early-phase rapid release may be due to the release of curcumin adsorbed on the surface of the nanospheres and the later sustained release may involve diffusion of curcumin from the inner core of the particles as well as degradation of the polymeric matrix. With the protection of PEG–PLA polymer shell, encapsulated curcumin in our nanoparticles is supposed to be more stable than free curcumin; sustained release of curcumin in buffers should further extend its life.
Although free curcumin is not as stable as encapsulated curcumin, it may have diffused directly into the neoplastic cells and was able to show its cytotoxic effect instantly; while nanospheres prevent the direct interaction between encapsulated curcumin and HeLa and MDA-MB-231 cells. This explains the little lower cytotoxicity of encapsulated Curc-NS compared with free curcumin (). For Curc-NS, curcumin release was only 77% in 2 days, but it exhibited comparable cell growth inhibition at most of the concentrations with respect to free curcumin (). When curcumin is taken orally, poor absorption and poor bioavailability severely limit its clinical utility. The data presented here show that curcumin can be totally dispersible in water by encapsulating into PEG–PLA nanospheres. Curc-NS not only stabilize but also retain the biological activity of curcumin in HeLa cells and MDA-MB-231 cells, indicating that curcumin itself has not been altered during formulation of nanospheres. The enhanced dispersibility of curcumin and no usage of organic solvent make Curc-NS a better candidate for disease therapy than free curcumin. Since curcumin has vasodilation property, Curc-NS can be potential therapeutic for vasodilation, particularly in situations with endothelial dysfunction. The small size and the hydrophilic PEG shell may allow Curc-NS to circulate for a long time in vivo after systemic application. Our future studies will focus on the efficacy of Curc-NS for vasodilation in animal models as well as a supplement for hemoglobin-based blood substitutes.
In summary, a facile emulsion method was developed to prepare biodegradable PEG–PLA nanospheres to encapsulate hydrophobic anticancer drug, curcumin. Compared with previously reported nanocurcumin formulations, our Curc-NS have advantages, such as homogeneous, narrow size distribution, high encapsulation efficiency, high drug loading, and environmental friendly preparation method. The cytotoxicity results show that Curc-NS represent a new method for delivering curcumin without compromising its toxicity against Hela and MDA-MB-231 cancer cells. Such nanotechnology-based delivery vehicle serves as an effective answer to one of the major challenges in current biomedical research: delivering any hydrophobic drug (such as curcumin) at a desired therapeutic amount to the target cells.
Funding information
This study was supported by a grant [P01 HL1,10900] from National Institutes of Health.
Acknowledgements
The authors would like to express great appreciation to Dr. Fantao Meng (Department of Medicine, Albert Einstein College of Medicine) for his instructive discussion; Dr. Brian P. O’Rourke (Department of Physiology & Biophysics, Albert Einstein College of Medicine) for his assistance with cell culture incubation; Dr. Enpeng Zhao (Department of Medicine, Albert Einstein College of Medicine) for his technical help with cytotoxic study and helpful discussion.
Disclosure statement
The authors report no conflicts of interest. The authors alone are responsible for the content and writing of the article.
References
- Aggarwal BB, Kumar A, Bharti AC. 2003. Anticancer potential of curcumin: preclinical and clinical studies. Anticancer Res. 23:363–398.
- Aggarwal BB, Sundaram C, Malani N, Ichikawa H. 2007. Curcumin: the Indian solid gold. Adv Exp Med Biol. 595:1–75.
- Aggarwal BB, Sung B. 2009. Pharmacological basis for the role of curcumin in chronic diseases: an age-old spice with modern targets. Trends Pharmacol Sci. 30:85–94.
- Anand P, Kunnumakkara AB, Newman RA, Aggarwal BB. 2007. Bioavailability of curcumin: problems and promises. Mol Pharm. 4:807–818.
- Anand P, Nair HB, Sung B, Kunnumakkara AB, Yadav VR, Tekmal RR, Aggarwal BB. 2010. Design of curcumin-loaded PLGA nanoparticles formulation with enhanced cellular uptake, and increased bioactivity in vitro and superior bioavailability in vivo. Biochem Pharmacol. 79:330–338.
- Anitha A, Deepa N, Chennazhi KP, Lakshmanan VK, Jayakumar R. 2014. Combinatorial anticancer effects of curcumin and 5-fluorouracil loaded thiolated chitosan nanoparticles towards colon cancer treatment. Biochim Biophys Acta. 1840:2730–2743.
- Bansal SS, Goel M, Aqil F, Vadhanam MV, Gupta RC. 2011. Advanced drug delivery systems of curcumin for cancer chemoprevention. Cancer Prev Res (Phila). 4:1158–1171.
- Cheng AL, Hsu CH, Lin JK, Hsu MM, Ho YF, Shen TS, et al. 2001. Phase I clinical trial of curcumin, a chemopreventive agent, in patients with high-risk or pre-malignant lesions. Anticancer Res. 21:2895–2900.
- Dewar AM, Clark RA, Singer AJ, Frame MD. 2011. Curcumin mediates both dilation and constriction of peripheral arterioles via adrenergic receptors. J Invest Dermatol. 131:1754–1760.
- Dhillon N, Aggarwal BB, Newman RA, Wolff RA, Kunnumakkara AB, Abbruzzese JL, et al. 2008. Phase II trial of curcumin in patients with advanced pancreatic cancer. Clin Cancer Res. 14:4491–4499.
- Dhule SS, Penfornis P, Frazier T, Walker R, Feldman J, Tan G, et al. 2012. Curcumin-loaded γ-cyclodextrin liposomal nanoparticles as delivery vehicles for osteosarcoma. Nanomedicine. 8:440–451.
- Egan ME, Pearson M, Weiner SA, Rajendran V, Rubin D, Glockner-Pagel J, et al. 2004. Curcumin, a major constituent of turmeric, corrects cystic fibrosis defects. Science. 304:600–602.
- Fang XD, Yang F, Zhu L, Shen YL, Wang LL, Chen YY. 2009. Curcumin ameliorates high glucose-induced acute vascular endothelial dysfunction in rat thoracic aorta. Clin Exp Pharmacol Physiol. 36:1177–1182.
- Gao W, Bian Y, Chang TMS. 2013. Novel nanodimension artificial red blood cells that act as O2 and CO2 carrier with enhanced antioxidant activity: PLA-PEG nanoencapsulated polySFHb-superoxide dismutase-catalase-carbonic anhydrase. Artif Cells Nanomed Biotechnol. 41:232–239.
- Gao X, Zheng F, Guo G, Liu X, Fan R, Qian Z, et al. 2013. Improving the anti-colon cancer activity of curcumin with biodegradable nano-micelles. J Mater Chem B. 1:5778–5790.
- Goel A, Kunnumakkara AB, Aggarwal BB. 2008. Curcumin as “Curecumin: from kitchen to clinic. Biochem Pharmacol. 75:787–809.
- Gref R, Luck M, Quellec P, Marchand M, Dellacherie E, Harnisch S, Blunk T, Muller RH. 2000. 'Stealth' corona-core nanoparticles surface modified by polyethylene glycol (PEG): influences of the corona (PEG chain length and surface density) and of the core composition on phagocytic uptake and plasma protein adsorption. Colloids Surf B Biointerfaces. 18:301–313.
- Krausz AE, Adler BL, Cabral V, Navati M, Doerner J, Charafeddine RA, et al. 2015. Curcumin-encapsulated nanoparticles as innovative antimicrobial and wound healing agent. Nanomedicine. 11:195–206.
- Kumar SS, Mahesh A, Mahadevan S, Mandal AB. 2014. Synthesis and characterization of curcumin loaded polymer/lipid based nanoparticles and evaluation of their antitumor effects on MCF-7 cells. Biochim Biophys Acta. 1840:1913–1922.
- Kurien BT, Singh A, Matsumoto H, Scofield RH. 2007. Improving the solubility and pharmacological efficacy of curcumin by heat treatment. Assay Drug Dev Technol. 5:567–576.
- Lao CD, Ruffin MTT, Normolle D, Heath DD, Murray SI, Bailey JM, et al. 2006. Dose escalation of a curcuminoid formulation. BMC Complement Altern Med. 6:10.
- Ma Z, Haddadi A, Molavi O, Lavasanifar A, Lai R, Samuel J. 2008. Micelles of poly(ethylene oxide)-b-poly(epsilon-caprolactone) as vehicles for the solubilization, stabilization, and controlled delivery of curcumin. J Biomed Mater Res A. 86:300–310.
- Meng FT, Ma GH, Qiu W, Su ZG. 2003. W/O/W double emulsion technique using ethyl acetate as organic solvent: effects of its diffusion rate on the characteristics of microparticles. J Control Rel. 91:407–416.
- Mirakabad FST, Akbarzadeh A, Milani M, Zarghami N, Taheri-Anganeh M, Zeighamian V, Badrzadeh F, Rahmati-Yamchi M. 2016. A Comparison between the cytotoxic effects of pure curcumin and curcumin-loaded PLGA-PEG nanoparticles on the MCF-7 human breast cancer cell line. Artif Cells Nanomed Biotechnol. 44:423–430.
- Mohanty C, Acharya S, Mohanty AK, Dilnawaz F, Sahoo SK. 2010. Curcumin-encapsulated MePEG/PCL diblock copolymeric micelles: a novel controlled delivery vehicle for cancer therapy. Nanomedicine (Lond). 5:433–449.
- Narayanan A, Kehn-Hall K, Senina S, Lundberg L, Van Duyne R, Guendel I, et al. 2014. Curcumin inhibits Rift Valley fever virus replication in human cells. J Biol Chem. 287:33198–33214.
- Nurullahoglu-Atalik KE, Okudan N, Belviranli M, Gokbel H, Simsek L. 2012. Curcumin increases vasodilatory effect of cilostazol in diabetic rat aorta. Indian J Exp Biol. 50:128–132.
- Rungseesantivanon S, Thenchaisri N, Ruangvejvorachai P, Patumraj S. 2010. Curcumin supplementation could improve diabetes-induced endothelial dysfunction associated with decreased vascular superoxide production and PKC inhibition. BMC Complement Altern Med. 10:57.
- Sharma RA, Euden SA, Platton SL, Cooke DN, Shafayat A, Hewitt HR, et al. 2004. Phase I clinical trial of oral curcumin: biomarkers of systemic activity and compliance. Clin Cancer Res. 10:6847–6854.
- Shi M, Cai Q, Yao L, Mao Y, Ming Y, Ouyang G. 2006. Antiproliferation and apoptosis induced by curcumin in human ovarian cancer cells. Cell Biol Int. 30:221–226.
- Somasundaram S, Edmund NA, Moore DT, Small GW, Shi YY, Orlowski RZ. 2002. Dietary curcumin inhibits chemotherapy-induced apoptosis in models of human breast cancer. Cancer Res. 62:3868–3875.
- Song Q, Wang X, Hu Q, Huang M, Yao L, Qi H, et al. 2013. Cellular internalization pathway and transcellular transport of pegylated polyester nanoparticles in Caco-2 cells. Int J Pharm. 445:58–68.
- Thu HP, Quang DT, Trang MTT, Ha TTH, Nam NH, Phuc NX, et al. 2013. Vitro apoptosis enhancement of Hep-G2 cells by PLA–TPGS and PLA–PEG block copolymer encapsulated curcumin nanoparticles. Chem Lett. 42:255–257.
- Tian B, Wang Z, Zhao Y, Wang D, Li Y, Ma L, et al. 2008. Effects of curcumin on bladder cancer cells and development of urothelial tumors in a rat bladder carcinogenesis model. Cancer Lett. 264:299–308.
- Tiyaboonchai W, Tungpradit W, Plianbangchang P. 2007. Formulation and characterization of curcuminoids loaded solid lipid nanoparticles. Int J Pharm. 337:299–306.
- Tonnesen HH, Karlsen J. 1985. Studies on curcumin and curcuminoids. VI. Kinetics of curcumin degradation in aqueous solution. Z Lebensm Unters Forsch. 180:402–404.
- Vila A, Gill H, McCallion O, Alonso MJ. 2004. Transport of PLA-PEG particles across the nasal mucosa: effect of particle size and PEG coating density. J Control Rel. 98:231–244.
- Xia H, Gao X, Gu G, Liu Z, Hu Q, Tu Y, et al. 2012. Penetratin-functionalized PEG-PLA nanoparticles for brain drug delivery. Int J Pharm. 436:840–850.
- Yang C, Chen H, Zhao J, Pang X, Xi Y, Zhai G. 2014. Development of a folate-modified curcumin loaded micelle delivery system for cancer targeting. Colloids Surf B Biointerfaces. 121:206–213.