Abstract
Rodent models harboring a simple yet functional human intestinal microbiota provide a valuable tool to study the relationships between mammals and their bacterial inhabitants. In this study, we aimed to develop a simplified gnotobiotic mouse model containing 10 easy-to-grow bacteria, readily available from culture repositories, and of known genome sequence, that overall reflect the dominant commensal bacterial makeup found in adult human feces. We observed that merely inoculating a mix of fresh bacterial cultures into ex-germ free mice did not guarantee a successful intestinal colonization of the entire bacterial set, as mice inoculated simultaneously with all strains only harbored 3 after 21 d. Therefore, several inoculation procedures were tested and levels of individual strains were quantified using molecular tools. Best results were obtained by inoculating single bacterial strains into individual animals followed by an interval of two weeks before allowing the animals to socialize to exchange their commensal microbes. Through this procedure, animals were colonized with almost the complete bacterial set (9/10). Differences in the intestinal composition were also reflected in the urine and plasma metabolic profiles, where changes in lipids, SCFA, and amino acids were observed. We conclude that adaptation of bacterial strains to the host’s gut environment (mono-colonization) may predict a successful establishment of a more complex microbiota in rodents.
Introduction
The quest for the ultimate mouse model to study the relationships between mammals and their bacterial inhabitants, at the laboratory scale, has been a great intellectual and technical adventure for decades. Research for an animal model capable of mimicking the “normal” state, i.e., keeping the essential microbial functionality, while remaining a simple system, has been the subject of several research groups around the globe. These models are valuable because they promise to reveal information about how animal-bacteria symbioses operate. Notwithstanding the differences in the gut microbial makeup of feral and laboratory animals and their implications on conclusions about host-bacteria cross-talk, substantial knowledge has been accumulated to date indicating a close association between the intestinal bacteria and a variety of functions: gut bacteria have been reported to shape the systemic immune systemCitation1 and the mucosal immune response,Citation2 modulate several morphological and physiological aspects of the intestine,Citation3 induce metabolic activity of mucosal epithelial cells,Citation4 ferment unused energy substrates,Citation5,Citation6 prevent the growth of harmful, pathogenic bacteria,Citation7,Citation8 produce vitamins and hormones,Citation9,Citation10 and metabolize diet carcinogens.Citation11
Gnotobiotic models, defined as ex-GF animals in which only known microorganisms are implanted, are at present considered as the best model to better understand these gut microbiota-host interactions. Originally developed to standardize the production of specific pathogen-free (SPF) animals by inoculating a few bacterial isolates originating from a healthy mouse, the altered Schaedler’s flora (ASF) reached great popularity during the late 70s and 80s.Citation12,Citation13 Over the years, breeders have used this ASF, and kept the animals for generations under strict barrier conditions to maintain their SPF status. Probably because members of this cocktail are poorly described (unknown genomes, obscure taxonomic information), are difficult to grow under laboratory conditions, and have not been deposited in any official culture repository, animals harboring this microbiota have not evolved to a widespread model to study microbial-host interactions. More recently, few reports described the development of alternative rodent models of simplified microbiota.Citation14,Citation15
Human flora-associated animals have also been used as a means to mimic more closely the interactions in the human gut. Several models have mushroomed, and it is now possible to find a wide variety of systems reflecting either the adult or infant microbiota in mice, rats or pigs with different levels of complexity.Citation16-Citation21 Unfortunately, most studies lack a detailed description of the microbial composition (especially in the more complex models), and often fail to describe how they compare with the original fecal inoculum, or how accurately they represent the human microbiota. Two recent reports, however, have addressed this caveat.Citation14,Citation22
With the advent of the functional genomics era, the understanding of ecological systems like that of the gut has been expanded through an integrated systems biology approach that incorporates a variety of “omics” techniques (metagenomics, transcriptomics, proteomics and metabonomics). Metabonomics, defined as “the quantitative measurement of the dynamic metabolic responses of living systems to pathophysiological stimuli or genetic modification,”Citation23 provides a suitable tool for the characterization of metabolites and their kinetic changes that represent actual end-points of physiological regulatory processes. Besides, parallel metabolite measurements have become a popular strategy to assess metabolic and physiological changes within a biological system and can be used to probe metabolic variations originating from the gut microbiota. “Omics” approaches have already been used in several animal studies to characterize host-microbiota interactions,Citation20,Citation24-Citation30 contributing to a better understanding of this complex ecological niche.
The purpose of the present study was (1) to evaluate different methods for obtaining a stable mouse model of simplified human microbiota colonized with easy-to-grow bacteria that are readily available from culture repositories, and of known genome sequence, reflecting as closely as possible the dominant commensal bacterial makeup found in adult human feces, and (2) to determine the impact of the resulting bacterial composition on urinary and plasma metabolic profiles of the host.
Results
Establishment of a simplified microbiota in the mouse gut by simultaneous inoculation of its members
Ten intestinal bacterial strains of human origin were selected based on numerical importance in feces, known fermentative abilities, and availability of full genome sequences (). These were grown separately until late exponential phase and used to prepare a mixed suspension containing 108–109 cells ml−1 of each strain. The freshly prepared suspension was inoculated by oral gavage to 14 GF mice housed in sterile isolators (Fig S1). Only three bacterial strains (Escherichia coli HS, Bacteroides vulgatus DSM1447 and Bacteroides thetaiotaomicron DSM2079) successfully colonized the mice until the end of the study. Colonizing bacteria were detected at 1011 cells g−1 in feces (Fig. S2). Counts corresponding to the remaining seven strains quickly dropped to undetectable levels during the first week after inoculation. Body weight of mice in group B after the introduction of a high-fat diet (day 26) increased faster than of mice in group A that remained on chow diet throughout. Maximal difference for body weight of both groups was observed at day 42 but this was not statistically significant (+3.0 g; p = 0.057). Diet modification had no impact on colonization of the only three persistent bacterial strains.
Table 1. Bacterial strains used for mouse inoculation with their respective growth media and primers used for real-time PCR enumeration
Optimization of the inoculation procedure to obtain a simplified gnotobiotic mouse model
Considering the low success of bacterial colonization observed in the first experiment, we designed a second experiment to test alternative methods of inoculation that would lead to improved colonization efficiency. The experimental design is described in and . Animals from group A received the mixed bacterial suspensions prepared from frozen stocks on five occasions during the first two weeks. Although initially all strains were widely detected in feces, only three strains persisted in all five mice at the end of the study (). Notably, the detected strains were the same to those that successfully colonized mice in the first experiment (i.e., Escherichia coli HS, Bacteroides vulgatus DSM1447 and Bacteroides thetaiotaomicron DSM2079). We observed similar results among the animals in group B which were inoculated twice during the first week with mixed fresh cultures suspensions following the same protocol as for the first experiment (data not shown). Animals in group C were inoculated twice during the first week with a bacterial mix prepared from fresh cultures without the three strains showing high persistence in the first experiment. This protocol was tested in order to favor colonization by less performing bacterial strains that did not colonize the mice in the first experiment. The three strains that colonized mice in the first experiment were only given to the mice 2 weeks after the initial gavage. Although we allowed for a considerable length of time before inoculating these three “strong” strains, it was impossible to recover any of the initial seven strains after day 23. The resulting profile both in cecal contents and feces was similar to that obtained in groups A and B as well as in the mice from the first experiment (data not shown). Because this result was replicated in two separate experiments, we believe that the dominance of these outperforming colonizers is a very reproducible phenomenon.
Figure 1. Experimental set up. Five groups of mice (males in groups A–D; females in group E) were individually caged and distributed into 5 isolators. Different bacterial suspensions (mixes or pure cultures) were inoculated at several time points between days 0 and 14 (see also ). On day 14, mice from groups D and E were removed from their individual cages and mixed with the other mice from the same group. On day 56, 3 GF mice were introduced into the isolators containing groups D and E. All animals received standard chow diet. p.i., post-inoculation.

Table 2. Protocol for the second mouse inoculation experiment
Figure 2. Colonization pattern in mice from groups A and D. Individual bacterial strains were quantified by real-time PCR in feces collected between days 3 and 70 post-inoculation and values are expressed as log10(cells) g−1 of feces. Dots represent bacterial counts in individual mice. Mean values are shown as lines between dots. The detection limit is approximately 106 cells g−1.
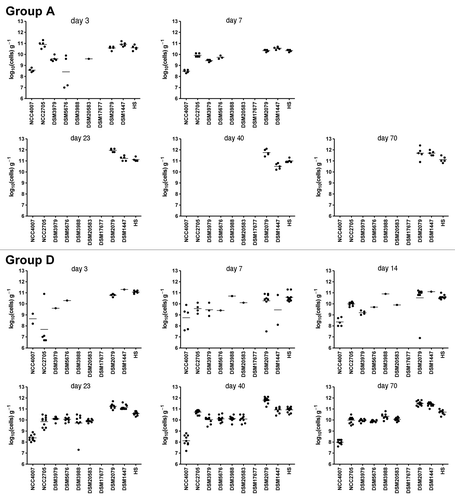
Animals in groups D and E (D, males; E, females) were inoculated twice during the first week with single-strain bacterial suspensions and on day 14 they were mixed with other mice from the same group to allow cross-transmission of bacterial strains. Both groups exhibited similar colonization patterns. Results from group D mice are summarized in . Although we inoculated pure bacterial suspensions in each animal and these were caged individually, we found initially more than one strain in some of the animals. This might be due to a cross-transmission during handling of mice that were housed in the same isolator. Before allowing all the animals to get together (day 14), some strains were not detected in the feces of the corresponding animals. However, almost all of the strains were systematically detected in all of the animals few days after the animals were put together, except for the strain Faecalibacterium prausnitzii DSM17677. In group E, the strain Collinsella aerofaciens DSM3979 was also not detected. At the end of the experiment (day 70), fecal bacteria counts ranged from 108 to 1011 cells g−1.
On day 56, three GF mice were introduced in the cages containing mice from groups D and E. One week after being in contact with their gnotobiotic counterparts, newly added mice adopted the full set of bacteria (), indicating that bacterial strains colonized more easily the new animals after they got established in the gut of the donors. Fecal bacterial counts of these receiver mice were comparable to those from the donors.
Figure 3. Transfer of the simplified microbiota from colonized mice to GF mice. Three GF male mice were introduced in the isolator containing mice from group D on day 56 post-inoculation. Individual bacterial strains were quantified by real-time PCR in feces collected at days 2, 7 and 14 after transfer of the GF mice and values are expressed as log10 (cells) g−1 of feces. Numbers in parenthesis indicate day post-inoculation of mice in group D. Dots represent values from individual mice. Mean values are shown as lines between dots. The detection limit is approximately 106 cells g−1.
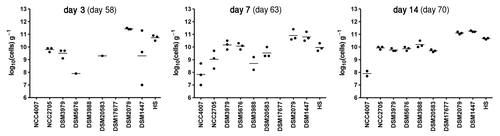
No significant difference was observed in body weight gain between different mice groups in the second experiment, indicating that there is no association between the simplified microbiota composition of the animals and their body weight. However, the cecum size was significantly larger in animals from groups A, B and C (all containing three strains; 0.96 ± 0.02 g) than those from groups D (containing 8–9 strains; 0.65 ± 0.01 g; p < 0.0001), suggesting a functional difference of the simplified microbiotas.
Assessment of viability in mixed-strain bacterial suspensions
Lack of colonization by the less performing bacterial colonizers observed in experiments where strains were inoculated as mixed-strain bacterial suspensions may suggest loss of viability during preparation of the inocula. To exclude this hypothesis, freshly prepared mixed-strain suspensions were used to inoculate two culture media which were known to promote growth of individual strains. Counts of most of the strains significantly increased 2 d after inoculation (Fig. S3). These results suggest that bacterial viability was maintained within the mixed-strain preparations.
Characterization of urinary and plasma metabolic profiles
Metabolic profiles were generated from urine and plasma samples to infer implications of the microbial diversity on the physiology of the mice. At the end of the study, 1H NMR spectra from urine showed a variety of resonances corresponding to various classes of compounds including organic acids such as acetate, butyrate, citrate, hippurate, keto-isovalerate, and taurine; and aliphatic amines such as methylamine (MMA), dimethylamine (DMA), trimethylamine (TMA), and trimethylamine-N-oxide (TMAO). Other metabolites such as creatine, creatinine, succinate, phenylacetylglycine, and amino acids (alanine, arginine, and lysine) were also identified (Fig. S4). In contrast, 1H NMR spectra from plasma displayed resonances corresponding mainly to saturated and unsaturated lipids, VLDL and LDL, as well as resonances from sugars such as α- and β-glucose (Fig. S5A, standard 1D acquisition; see supplementary information for more explanations on 1H NMR acquisition methods). Additional metabolites present at lower concentrations were detected in plasma using a CPMG acquisition method, including acetate, lactate, pyruvate, amino-acids (alanine, glutamine, leucine, isoleucine and valine) and creatine (Fig. S5B).
Impact of microbiota composition on metabolic profiles
Metabolic profiles from urine and plasma were compared between mice harboring different microbiota composition using multivariate data analysis (see supplementary data). Because we did not observe any statistical differences in either matrix between groups A, B and C, which harbored the same set of bacterial strains, we decided to pool together metabolic data from these three groups for further multivariate statistical analysis. Pairwise comparisons generated in urine showed that animals colonized with three bacterial strains displayed higher levels of allantoin, dimethylamine, and creatinine compared with mice containing nine bacterial strains (). However, animals with higher bacterial biodiversity displayed higher levels of n-butyrate, formate and the amino acids glutamine and lysine. Similarly, plasma metabolites showed that mice colonized with three strains resulted in higher levels of VLDL, unsaturated lipids, and choline than animals colonized with nine strains, but lower levels of creatine and proline. It is noteworthy that discrimination between groups with low and high microbial diversity was more important in metabolic profiles from urine than those from plasma, regardless of the type of acquisition ().
Table 3. Summary of variations of plasma (P) and urinary (U) metabolite signals obtained by pairwise comparisons between animals harboring 3 (pooled data from groups A, B and C), 9 (group D; males) and 8 (group E; females) bacterial strains
Gender-associated differences in metabolic profiles
Urinary and plasma metabolic profiles obtained by 1H NMR were also compared between male and female mice harboring the almost complete microbiota set (9 and 8 strains, respectively). In urine, males showed higher levels of butyrate, trimethylamine, hydroxy-isovalerate, N- and O-acetylglycoproteins, and lysine than females, but lower levels of 2-oxo-isocaproate, allantoin and trimethylamine-N-oxide. Plasma samples from males showed higher levels of cholesterol, lipids (mainly VLDL, and unsaturated lipids), choline and proline than females, but lower levels of alanine and taurine (). Similarly to what has been described for effects dependent on microbial composition, discrimination associated to gender was more important in urine metabolic data than in plasma.
Discussion
The complex bacterial communities that inhabit our guts have a strong relationship with us at the point of being classified as our mutualistic symbionts.Citation35 Their beneficial impact on our physiology spans from protection against pathogenic attackCitation36,Citation37 to development of the immune system,Citation38,Citation39 and energy and vitamin supply,Citation9,Citation40,Citation41 while apparently benefiting themselves by controlling the composition of their microbial environment, rich in nutrients and maintained at a stable temperature. It is not only because of these attributes but also because of their remarkable biodiversity that gut microbiologists have been trying to develop methods to study this symbiotic relationship with man.
In this context, we designed our bacterial cocktail based on the criteria detailed previously. Because in our hands standard methods for inoculation did not result in a complete colonization of the microbiota, we followed a sui generis approach, i.e., unique in its characteristics, expecting to achieve high and diverse colonization levels in our rodent colonies. Through this method, each animal was initially gavaged with one single bacterial strain to allow for a successful colonization in this former GF environment. After a few weeks, all 10 animals were allowed to socialize. Taking advantage of the coprophagic habits of the rodents, we expected to promote a diversified bacterial colonization in all of the animals. This approach has been applied on males and females alike, contained in separate isolators to avoid territorial issues at the time of putting the animals together. This approach proved to be much more successful than successive inoculations of the whole bacterial mixture (either freshly prepared or from thawed frozen stocks), or a step-wise inoculation of subgroups from the mix. With the exception of one strain in males, and two strains in females, all other members of the cocktail reached over 108 cells g−1 feces or cecal contents at the end of the experiment. Moreover, GF animals introduced to these isolators on day 56 of the study, quickly acquired a similar bacterial community to that of the donor animals, suggesting that the transferred microbial community had achieved a significant level of stability and adaptation to the host gut environment.
One can speculate that in the intestinal tract of GF animals there is a limited competitive pressure for colonization to a newly inoculated microorganism, making it relatively easy to deliberately colonize these animals with a few defined microbial species. This concept, however, was challenged in this study, and prompted us to develop this specific inoculation procedure. Indeed, bacteria-bacteria interactions, even in the simplest bacterial consortia, must be considered as part of the equation, and may be the reason why it has been often difficult to create and maintain a stable human flora-associated model. In contrast to the results presented in this study, two recent reports described the development of simplified microbiota models in miceCitation15 and in ratsCitation14 that were obtained by direct inoculation of mixed-strain bacteria suspensions containing 15 and 7 strains, respectively. The discrepancy with these studies might be due to differences in the interaction of the bacterial strains composing each simplified microbiota that might lead to varying degrees of selective pressure on less performing strains. It is important to note, however, that other studies were in agreement with our results suggesting that competitive interaction between members of a simplified microbiota might prevent establishment of less performing strains.Citation42,Citation43
Three strains of the mix used in the study were systematically found to be good colonizers: B. vulgatus, B. thetaiotaomicron and E. coli. Species of the genus Bacteroides have been shown to be associated to luminal contents (food particles and shed mucus), and also embedded in the mucus layer overlying the epithelium.Citation44 It has been proposed that the gel layer overlying the intestinal epithelium is a key contributor to the structural and functional stability of the microbiota. E. coli can grow rapidly in mucus obtained from the mouse intestine but not in intestinal luminal contents.Citation45 Therefore, it is possible that those microorganisms with the ability to attach to mucus and use it as a carbon source have a competitive advantage to colonize the gut environment.
Any loss of viability during the preparation of the inocula was ruled out because freshly-prepared mixed bacterial suspensions were able to grow in vitro using two culture media which were known to promote growth of individual strains. A change in the diet did not result in any modification of the bacterial composition in our first experiment, and discouraged us to test this hypothesis again in the following experiment.
We looked into whether the different bacterial compositions affected metabolic profiles of the animals in plasma and urine. In fact, we observed significant differences in plasma and urine metabolites between mice harboring 3 and 9 bacterial strains. First, mice harboring three bacterial strains showed higher levels of lipids and lipoproteins, especially VLDL, compared with those colonized with nine bacterial strains. These results suggest that either the presence/absence of certain members of the microbiota or the different microbial diversity, or a combination of both, are associated to a more efficient regulation of lipoprotein levels and circulating lipids, which may have implications for weight management. GF animals, for instance, have higher levels of VLDL compared with conventional animals (Mestdagh R, personal communication). Variations in lipoprotein levels in plasma matched those of SCFA, n-butyrate, and formate in urine. SCFA are formed by microbial fermentation of dietary polysaccharides and they have been previously demonstrated to have a beneficial effect on the host. In humans, specific SCFA may help to reduce developments of gastrointestinal disorders, cancer and cardiovascular disease.Citation46-Citation48 Butyrate is considered the preferred source of energy for colonocytes.Citation49 When butyrate is added to germ free colonocytes, it rescues their deficit in mitochondrial respiration and prevents them from undergoing autophagy.Citation50 In addition, butyrate is known to promote efficient absorption of nutrients and to prevent colon cancer and adenoma development in animal and cell line studies. Faecalibacterium prausnitzii DSM17677, which failed to establish in the inoculated mice, has been reported as one of the most predominant butyrate producer.Citation51,Citation52 The ability to produce butyrate is widely distributed among colonic Gram-positive anaerobic bacteria. Interestingly, butyrate was detected in urine from mice colonized with 3 and 9 bacterial strains in the absence of F. prausnitzii indicating that other bacteria are able to produce butyrate. Animals harboring 9 strains displayed higher levels of butyrate when compared with mice colonized with three strains, suggesting that colonization with those 9 strains results in a more efficient butyrate production.Citation49,Citation53,Citation54 This phenomenon could be attributed to an improvement or gain of function due to the bacteria association and bacteria-bacteria interactions. It has been reported that many butyrate producers from the gut environment can also produce hydrogen and formate, with a direct dependence on the environmental conditions.
Unlike butyrate, the metabolic function of formate has not been well documented and its potential beneficial role is not known up to date. Nevertheless, Macfarlane and Macfarlane reported that formate was one of the principal products of dietary polysaccharides fermentation by the species Bifidobacterium breve under carbohydrate-limited conditions.Citation53 The difference in formate production observed in this study could be due to the lower amount of carbohydrates digested by the gut microbial species in mice harboring three bacteria compared with those harboring nine bacteria. Nevertheless, this result would have to be complemented in the future by the comparison with formate production in conventional C3H animals. Finally, it is noteworthy that urinary and plasma metabolic profiles of this strain of mice highlighted major changes in metabolites linked to energy metabolism and amino acids pool regulation. Indeed, we observed variations of creatine and creatinine, possibly indicating a perturbation of energy metabolism in mice with the simplest microbiota, as well as higher levels of amino acids such as proline, lysine and glutamine in mice colonized with nine bacteria compared with those containing only three bacteria in their gut.
Although differences in metabolic profiles were more important between males and females than between mice with different microbial diversity, our results suggest that high intestinal microbial biodiversity is associated with better metabolic regulation than animals harboring a simpler gut microbial makeup which show metabolic signatures that could be associated with pre-pathological conditions associated to the development of obesity, diabetes, and metabolic disorders.Citation55,Citation56
We observed comparable weight gain in all of the animals regardless of their microbiota, but we noticed that animals with low bacterial diversity had larger ceca. Large cecum sizes have been systematically reported for GF or mono-colonized rodents since the first production of a GF mouse in the late 1950s,Citation42,Citation57-Citation59 and it has been associated to the absence of bacterial components stimulating the cecal muscle tone,Citation60 and accumulation of secretion of water and electrolytes,Citation61 among other causes (reviewed in ref. Citation62). The reduction in cecum size associated with increasing microbiota diversity is indicative of a clear difference in functional properties between the two microbiotas.
To conclude, we have been able to generate, maintain and metabolically characterize a gnotobiotic mouse of human simplified microbiota. In particular, we showed that previous adaptation of bacterial strains to the host’s gut environment (mono-colonization) may predict a successful establishment of a more complex microbiota in rodents. We have demonstrated that microbial composition affects urinary and plasma metabolic profiles, which may have implications for the physiology of the host.
Materials and Methods
Bacterial strains and culture conditions
shows the list of bacterial strains used in this study. All strains were of human intestinal origin and were selected to mimic proportion of bacterial phyla found in healthy human feces. B. longum NCC2705 and L. rhamnosus NCC4007 were obtained from the Nestlé Culture Collection; E. coli HS was kindly provided by D. Rasko (University of Maryland, School of Medicine),Citation63 and the remaining strains were obtained from the German Collection of Microorganisms and Cell Cultures (Deutsche Sammlung von Mikroorganismen und Zellculturen GmbH, DSMZ). Growth media used to grow individual bacterial strains are shown in . Brain Heart Infusion (BHI) medium was prepared as previously described.Citation31 Yeast extract Casitone Fatty Acids medium (YCFA) was prepared as previously described,Citation33 except for minor modifications in the concentration of SCFA. These were added at final concentrations of 18.4 mM acetate, 5 mM propionate, 0.67 mM iso-butyrate, 0.57 mM iso-valerate, and 0.57 mM valerate. E. coli HS was incubated aerobically at 37°C under continuous stirring. B. longum NCC2705 and L. rhamnosus NCC4007 were incubated at 37°C in an anaerobic chamber. The remaining bacteria were grown under strictly anaerobic conditions according to Hungate technique.Citation64
Preparation of bacterial inocula
Cultures of individual bacterial strains were synchronized in order to obtain early stationary phase cultures on the day of inoculation. For inoculation from fresh cultures (groups A and B in experiment 1 and groups B–E in experiment 2), 50 ml cultures of each strain were centrifuged at 4°C for 5 min at 4600 × g, followed by suspension of bacterial pellets under anoxic conditions in 5 ml pre-reduced ¼ strength Ringer solution (Oxoid). Inocula were prepared under anoxic conditions by mixing 500 µl of each concentrated bacterial suspension and adjustment of the final to 5 ml with pre-reduced Ringer solution. Aliquots of these suspensions were immediately frozen until analysis of bacterial counts. For inoculation of mice of group A in experiment 2, 10-fold concentrated frozen stocks of each bacterial strain were prepared under anoxic conditions by suspension of bacterial pellets in ¼ strength pre-reduced Ringer solution containing 10% glycerol and freezing at -80°C. Shortly before administration to mice, frozen stocks were thawed and inoculation suspensions were prepared under anoxic conditions by mixing 200 µl of each strain followed by adjustment of final volumes to 2 ml with pre-reduced ¼ strength Ringer solution.
Animals, diets and husbandry
The study was approved by the Veterinary Office of Canton de Vaud (Lausanne, Switzerland). GF 6-week-old C3H/HeN mice were purchased from Centre National de la Recherche Scientifique—Cryopréservation, distribution, typage et archivage animal (CNRS-CDTA; Orléans, France). Immediately after arrival at our facility, mice were housed in GF isolators, on a 12h/12h day/night cycle, room temperature of 20–24°C, relative humidity of 50–60%, with free access to sterile chow diet (R03, SAFE, France). After one week of acclimatization, mice were randomized by weight and transferred in different isolators according to treatment group where they were housed individually and fed ad libitum sterile chow diet or high-fat diet (D12492, Research Diets, USA) according to the study design (see below).
Study design
A schematic representation of the experiments performed in this study is shown in , Figure S1 and . Briefly, in the first experiment, 7-week-old GF C3H/HeN mice were inoculated with 300 µl of a freshly prepared bacterial suspension containing the 10 selected strains that were administered twice on days 0 and 2 by intragastric oral gavage, using sterile stainless-steel stomach tubes. On day 26 post-inoculation, animals in group B were switched to a high-fat diet, whereas animals in group A continued to receive chow diet for the rest of the experiment. Body weight, food and water intakes were recorded weekly throughout the study. Animals were sacrificed on day 42 post-inoculation by exhaustive bleeding under isoflurane anesthesia.
Based on results from the first experiment a second experiment was designed where alternative inoculation procedures were tested. Five groups of 7-week-old C3H/HeN male (groups A–D) and female (group E) mice were inoculated by intragastric oral gavage of 300 µl of the different bacterial suspensions inoculated at various time points, using sterile stainless-steel stomach tubes. Mice from group A received on days 0, 2, 5 and 7 a bacterial cocktail prepared from frozen glycerol stocks of the ten strains that were thawed shortly before inoculation. The four strains that did not persist according to fecal measurements performed at the end of week 1 were inoculated again on day 14 following the same procedure. Mice of group B received on days 0 and 2 a mixture of the 10 bacterial strains prepared from fresh cultures, followed by a third inoculation on day 14 with the seven less performing strains that were prepared following the same procedure. Mice from group C received on days 0 and 2 a mixture of the seven bacterial strains that failed to persist in the first experiment, prepared from fresh cultures. On day 14, mice were inoculated again with the strains that did not persist according to fecal measurements performed at the end of week 1, together with the successful colonizers of the first experiment. Finally, mice in groups D and E were inoculated on days 0 and 2 with single-strain bacterial suspensions (one strain per mouse) prepared from fresh cultures. From day 14 onwards, mice from each group were allowed to socialize in order to favor cross-transmission of individual bacterial strains. On day 56, three GF mice were introduced in isolators containing mice from groups D and E to evaluate whether the established microbiota from donor animals would be transferred to GF mice. All mice in the second experiment were given free access to standard chow diet. Body weight, food and water intake were recorded weekly throughout the study. Mice were sacrificed on day 70 post-inoculation by exhaustive bleeding under isoflurane anesthesia.
Sample collection and storage
In both experiments, fresh fecal samples were collected from individual mice every 2–3 d and feces were stored at -80°C until analysis. In the second experiment, spot urine samples from individual mice were collected at day 70 post-inoculation and frozen at -80°C until analysis. Animals were euthanized under isoflurane anesthesia and blood was collected from the vena cava into chilled heparin-coated tubes and centrifuged at 4°C for 10 min at 2,500 × g. Plasma was aliquoted and frozen at -80°C until analysis. The GIT was collected and the cecum removed and weighted. Cecal contents were transferred to a sterile tube and stored at -80°C until analysis.
Metabolic profiling of biofluids
The method for 1H NMR-based metabonomic analysis of urine and plasma samples is described in supplementary data.
Statistical analysis
Statistical analyses were performed using the softwares GraphPad Prism 5.02 and R 2.6.1 (Foundation for Statistical Computing, Vienna, Austria). Significance tests for body weight measurements were performed by Wilcoxon exact tests. Significance tests for cecum weights were performed by Mann-Whitney tests. Multivariate statistical analyses of metabolic data were performed as described in the supplementary data.
Abbreviations: | ||
ASF | = | altered Schaedler’s flora |
GF | = | germ-free |
NCC | = | Nestlé culture collection |
SCFA | = | short chain fatty acids |
VLDL | = | very low-density lipoprotein |
LDL | = | low-density lipoprotein |
Additional material
Download Zip (453.8 KB)Disclosure of Potential Conflict of Interest
No potential conflicts of interest were disclosed.
Acknowledgments
The authors would like to acknowledge the assistance of Massimo Marchesini, Christophe Maubert, Aurore Deschamps and Muriel Briand at the animal facility; Mireille Moser for the statistical analysis; Francis Foata and Mathieu Membrez for technical assistance; Sandrine Claus, Olaf Beckonert and John Lindon for assistance in the acquisition of metabolic profiles and multivariate analysis.
References
- Kawaguchi-Miyashita M, Nanno M, Shimada S, Nagaoka N, Okada Y, Matsumoto S, et al. A step-wise expansion of intestinal intraepithelial T lymphocytes in association with microbial colonization is defined by sensitivity to cyclosporin A. Immunology 1997; 91:628 - 34; http://dx.doi.org/10.1046/j.1365-2567.1997.00292.x; PMID: 9378505
- Shroff KE, Meslin K, Cebra JJ. Commensal enteric bacteria engender a self-limited humoral mucosal immune response wile permanently colonizing the gut. Infect Immun 1995; 63:3904 - 13; PMID: 7558298
- Syed SA, Abrams GD, Freter R. Efficiency of various intestinal bacteria in assuming normal functions of enteric flora after association with germfree mice. Infect Immun 1970; 2:376 - 86; PMID: 16557849
- Hooper LV, Xu J, Falk PG, Midtvedt T, Gordon JI. A molecular sensor that allows a gut commensal to control its nutrient foundation in a competitive ecosystem. Proc Natl Acad Sci USA 1999; 96:9833 - 8; http://dx.doi.org/10.1073/pnas.96.17.9833; PMID: 10449780
- Salyers AA, West SE, Vercellotti JR, Wilkins TD. Fermentation of mucins and plant polysaccharides by anaerobic bacteria from the human colon. Appl Environ Microbiol 1977; 34:529 - 33; PMID: 563214
- Englyst HN, Macfarlane GT. Breakdown of resistant and readily digestible starch by human gut bacteria. J Sci Food Agric 1986; 37:699 - 706; http://dx.doi.org/10.1002/jsfa.2740370717
- Lupp C, Robertson ML, Wickham ME, Sekirov I, Champion OL, Gaynor EC, et al. Host-mediated inflammation disrupts the intestinal microbiota and promotes the overgrowth of Enterobacteriaceae. Cell Host Microbe 2007; 2:204; http://dx.doi.org/10.1016/j.chom.2007.08.002; PMID: 18030708
- Mazmanian SK, Round JL, Kasper DL. A microbial symbiosis factor prevents intestinal inflammatory disease. Nature 2008; 453:620 - 5; http://dx.doi.org/10.1038/nature07008; PMID: 18509436
- Hill MJ. Intestinal flora and endogenous vitamin synthesis. Eur J Cancer Prev 1997; 6:S43 - 5; http://dx.doi.org/10.1097/00008469-199703001-00009; PMID: 9167138
- Cani PD, Possemiers S, Van de Wiele T, Guiot Y, Everard A, Rottier O, et al. Changes in gut microbiota controls inflammation in obese mice through a mechanism involving GLP-2 driven-changes in gut permeability. Gut 2009; 58:1091 - 103; http://dx.doi.org/10.1136/gut.2008.165886; PMID: 19240062
- Kassie F, Rabot S, Kundi M, Chabicovsky M, Qin HM, Knasmuller S. Intestinal microflora plays a crucial role in the genotoxicity of the cooked food mutagen 2-amino-3-methylimidazo [4,5-f]quinoline. Carcinogenesis 2001; 22:1721 - 5; http://dx.doi.org/10.1093/carcin/22.10.1721; PMID: 11577015
- Schaedler RW, Dubos R, Costello R. Association of germfree mice with bacteria isolated from normal mice. J Exp Med 1965; 122:77 - 82; http://dx.doi.org/10.1084/jem.122.1.77; PMID: 14325475
- Orcutt RP, Gianni FJ, Judge RJ. Development of an ‘Altered Schaedler flora’ for NCI gnotobiotic rodents. Microecol Ther 1987; 17:59
- Becker N, Kunath J, Loh G, Blaut M. Human intestinal microbiota: characterization of a simplified and stable gnotobiotic rat model. Gut Microbes 2011; 2:25-33; PMID: 21637015
- Goodman AL, McNulty NP, Zhao Y, Leip D, Mitra RD, Lozupone CA, et al. Identifying genetic determinants needed to establish a human gut symbiont in its habitat. Cell Host Microbe 2009; 6:279 - 89; http://dx.doi.org/10.1016/j.chom.2009.08.003; PMID: 19748469
- Barc M-C, Charrin-Sarnel C, Rochet V, Bourlioux F, Sandre C, Boureau H, et al. Molecular analysis of the digestive microbiota in a gnotobiotic mouse model during antibiotic treatment: influence of Saccharomyces boulardii. Anaerobe 2008; 14:229 - 33; http://dx.doi.org/10.1016/j.anaerobe.2008.04.003; PMID: 18511310
- Hirayama K, Miyaji K, Kawamura S, Itoh K, Takahashi E, Mitsuoka T. Development of intestinal flora of human-flora-associated (HFA) mice in the intestine of their offspring. Exp Anim 1995; 44:219 - 22; http://dx.doi.org/10.1538/expanim.44.219; PMID: 7556423
- Imaoka A, Setoyama H, Takagi A, Matsumoto S, Umesaki Y. Improvement of human faecal flora-associated mouse model for evaluation of the functional foods. J Appl Microbiol 2004; 96:656 - 63; http://dx.doi.org/10.1111/j.1365-2672.2004.02189.x; PMID: 15012802
- Mahowald MA, Rey FE, Seedorf H, Turnbaugh PJ, Fulton RS, Wollam A, et al. Characterizing a model huma gut microbiota composed of members of its two dominant bacteria phyla. Proc Natl Acad Sci USA 2009; 106:5859 - 64; http://dx.doi.org/10.1073/pnas.0901529106; PMID: 19321416
- Martin FP, Dumas ME, Wang Y, Legido-Quigley C, Yap IK, Tang H, et al. A top-down systems biology view of microbiome-mammalian metabolic interactions in a mouse model. Mol Syst Biol 2007; 3:112; http://dx.doi.org/10.1038/msb4100153; PMID: 17515922
- Pang X, Hua X, Yang Q, Ding D, Che C, Cui L, et al. Inter-species transplantation of gut microbiota form human to pigs. ISME J 2007; 1:156 - 62; http://dx.doi.org/10.1038/ismej.2007.23; PMID: 18043625
- Turnbaugh PJ, Ridaura VK, Faith JJ, Rey FE, Knight R, Gordon JI. The effect of diet on the human gut microbiome: a metagenomic analysis in humanized gnotobiotic mice. Sci Transl Med 2009; 1:ra14; http://dx.doi.org/10.1126/scitranslmed.3000322; PMID: 20368178
- Nicholson JK, Lindon JC, Holmes E. ‘Metabonomics': understanding the metabolic responses of living systems to pathophysiological stimuli via multivariate statistical analysis of biological NMR spectroscopic data. Xenobiotica 1999; 29:1181 - 9; http://dx.doi.org/10.1080/004982599238047; PMID: 10598751
- Claus SP, Tsang TM, Wang Y, Cloarec O, Skordi E, Martin FP, et al. Systemic multicompartmental effects of the gut microbiome on mouse metabolic phenotypes. Mol Syst Biol 2008; 4:219; http://dx.doi.org/10.1038/msb.2008.56; PMID: 18854818
- Claus SP, Ellero SL, Berger B, Krause L, Bruttin A, Molina J, et al. Colonization-induced host-gut microbial metabolic interaction. MBio 2011; 2:e00271 - 10; http://dx.doi.org/10.1128/mBio.00271-10; PMID: 21363910
- Dumas ME, Barton RH, Toye A, Cloarec O, Blancher C, Rothwell A, et al. Metabolic profiling reveals a contribution of gut microbiota to fatty liver phenotype in insulin-resistant mice. Proc Natl Acad Sci USA 2006; 103:12511 - 6; http://dx.doi.org/10.1073/pnas.0601056103; PMID: 16895997
- Gill SR, Pop M, DeBoy RT, Eckburg PB, Turnbaugh PJ, Samuel BS, et al. Metagenomic analysis of the human distal gut microbiome. Science 2006; 312:1355 - 9; http://dx.doi.org/10.1126/science.1124234; PMID: 16741115
- Martin FP, Wang Y, Sprenger N, Yap IK, Lundstedt T, Lek P, et al. Probiotic modulation of symbiotic gut microbial-host metabolic interactions in a humanized microbiome mouse model. Mol Syst Biol 2008; 4:157; http://dx.doi.org/10.1038/msb4100190; PMID: 18197175
- Martin FP, Wang Y, Sprenger N, Yap IK, Rezzi S, Ramadan Z, et al. Top-down systems biology integration of conditional prebiotic modulated transgenomic interactions in a humanized microbiome mouse model. Mol Syst Biol 2008; 4:205; http://dx.doi.org/10.1038/msb.2008.40; PMID: 18628745
- Yap IK, Li JV, Saric J, Martin FP, Davies H, Wang Y, et al. Metabonomic and microbiological analysis of the dynamic effect of vancomycin-induced gut microbiota modification in the mouse. J Proteome Res 2008; 7:3718 - 28; http://dx.doi.org/10.1021/pr700864x; PMID: 18698804
- Le Blay G, Lacroix C, Zihler A, Fliss I. In vitro inhibition activity of nisin A, nisin Z, pediocin PA-1 and antibiotics against common intestinal bacteria. Lett Appl Microbiol 2007; 45:252 - 7; http://dx.doi.org/10.1111/j.1472-765X.2007.02178.x; PMID: 17718835
- Penders J, Thijs C, van den Brandt PA, Kummeling I, Snijders B, Stelma F, et al. Gut microbiota composition and development of atopic manifestations in infancy: the KOALA Birth Cohort Study. Gut 2007; 56:661 - 7; http://dx.doi.org/10.1136/gut.2006.100164; PMID: 17047098
- Duncan SH, Barcenilla A, Stewart CS, Pryde SE, Flint HJ. Acetate utilization and butyryl coenzyme A (CoA):acetate-CoA transferase in butyrate-producing bacteria from the human large intestine. Appl Environ Microbiol 2002; 68:5186 - 90; http://dx.doi.org/10.1128/AEM.68.10.5186-5190.2002; PMID: 12324374
- Matsuki T, Watanabe K, Fujimoto J, Takada T, Tanaka R. Use of 16S rRNA gene-targeted group-specific primers for real-time PCR analysis of predominant bacteria in human feces. Appl Environ Microbiol 2004; 70:7220 - 8; http://dx.doi.org/10.1128/AEM.70.12.7220-7228.2004; PMID: 15574920
- Xu J, Gordon JI. Honor thy symbionts. Proc Natl Acad Sci USA 2003; 100:10452 - 9; http://dx.doi.org/10.1073/pnas.1734063100; PMID: 12923294
- Dubos RJ, Schaedler RW. The effect of the intestinal flora on the growth rate of mice, and on their susceptibility to experimental infections. J Exp Med 1960; 111:407 - 17; http://dx.doi.org/10.1084/jem.111.3.407; PMID: 13724628
- van der Waaij D. The ecology of the human intestine and its consequences for overgrowth by pathogens such as Clostridium difficile. Annu Rev Microbiol 1989; 43:69 - 87; http://dx.doi.org/10.1146/annurev.micro.43.1.69; PMID: 2679366
- Mazmanian SK, Liu CH, Tzianabos AO, Kasper DL. An immunomodulatory molecule of symbiotic bacteria directs maturation of the host immune system. Cell 2005; 122:107 - 18; http://dx.doi.org/10.1016/j.cell.2005.05.007; PMID: 16009137
- Stappenbeck TS, Hooper LV, Gordon JI. Developmental regulation of intestinal angiogenesis by indigenous microbes via Paneth cells. Proc Natl Acad Sci USA 2002; 99:15451 - 5; http://dx.doi.org/10.1073/pnas.202604299; PMID: 12432102
- Bäckhed F, Ding H, Wang T, Hooper LV, Koh GY, Nagy A, et al. The gut microbiota as an environmental factor that regulates fat storage. Proc Natl Acad Sci USA 2004; 101:15718 - 23; http://dx.doi.org/10.1073/pnas.0407076101; PMID: 15505215
- Gustafsson BE. Vitamin K deficiency in germfree rats. Ann N Y Acad Sci 1959; 78:166 - 74; http://dx.doi.org/10.1111/j.1749-6632.1959.tb53101.x; PMID: 13830426
- Denou E, Rezzonico E, Panoff JM, Arigoni F, Brüssow H. A mesocosm of Lactobacillus johnsonii, Bifidobacterium longum, and Escherichia coli in the mouse gut. DNA Cell Biol 2009; 28:413 - 22; http://dx.doi.org/10.1089/dna.2009.0873; PMID: 19534605
- Leatham MP, Banerjee S, Autieri SM, Mercado-Lubo R, Conway T, Cohen PS. Precolonized human commensal Escherichia coli strains serve as a barrier to E. coli O157:H7 growth in the streptomycin-treated mouse intestine. Infect Immun 2009; 77:2876 - 86; http://dx.doi.org/10.1128/IAI.00059-09; PMID: 19364832
- Sonnenburg JL, Xu J, Leip DD, Chen C-H, Westover BP, Weatherford J, et al. Glycan foraging in vivo by an intestine-adapted bacterial symbiont. Science 2005; 307:1955 - 9; http://dx.doi.org/10.1126/science.1109051; PMID: 15790854
- Sonnenburg JL, Angenent LT, Gordon JI. Getting a grip on things: how do communities of bacterial symbionts become established in our intestine?. Nat Immunol 2004; 5:569 - 73; http://dx.doi.org/10.1038/ni1079; PMID: 15164016
- Bugaut M, Bentejac M. Biological effects of short-chain fatty acids in nonruminant mammals. Annu Rev Nutr 1993; 13:217 - 41; http://dx.doi.org/10.1146/annurev.nu.13.070193.001245; PMID: 8369146
- Wong JM, de Souza R, Kendall CW, Emam A, Jenkins DJ. Colonic health: fermentation and short chain fatty acids. J Clin Gastroenterol 2006; 40:235 - 43; http://dx.doi.org/10.1097/00004836-200603000-00015; PMID: 16633129
- Guarner F, Malagelada JR. Gut flora in health and disease. Lancet 2003; 361:512 - 9; http://dx.doi.org/10.1016/S0140-6736(03)12489-0; PMID: 12583961
- Velázquez OC, Lederer HM, Rombeau JL. Butyrate and the colonocyte. production, absorption, metabolism, and therapeutic implications. Adv Exp Med Biol 1997; 427:123 - 34; PMID: 9361838
- Donohoe DR, Garge N, Zhang X, Sun W, O'Connell TM, Bunger MK, et al. The microbiome and butyrate regulate energy metabolism and autophagy in the mammalian colon. Cell Metab 2011; 13:517 - 26; http://dx.doi.org/10.1016/j.cmet.2011.02.018; PMID: 21531334
- Suau A, Bonnet R, Sutren M, Godon JJ, Gibson GR, Collins MD, et al. Direct analysis of genes encoding 16S rRNA from complex communities reveals many novel molecular species within the human gut. Appl Environ Microbiol 1999; 65:4799 - 807; PMID: 10543789
- Louis P, Flint HJ. Diversity, metabolism and microbial ecology of butyrate-producing bacteria from the human large intestine. FEMS Microbiol Lett 2009; 294:1 - 8; http://dx.doi.org/10.1111/j.1574-6968.2009.01514.x; PMID: 19222573
- Macfarlane S, Macfarlane GT. Regulation of short-chain fatty acid production. Proc Nutr Soc 2003; 62:67 - 72; http://dx.doi.org/10.1079/PNS2002207; PMID: 12740060
- Roediger WE. Oxidative and synthetic functions of n-butyrate in colonocytes. Dis Colon Rectum 1992; 35:511 - 2; http://dx.doi.org/10.1007/BF02049412; PMID: 1568404
- Li H, Xie Z, Lin J, Song H, Wang Q, Wang K, et al. Transcriptomic and metabonomic profiling of obesity-prone and obesity-resistant rats under high fat diet. J Proteome Res 2008; 7:4775 - 83; http://dx.doi.org/10.1021/pr800352k; PMID: 18828625
- Musso G, Gambino R, Cassader M. Interactions between gut microbiota and host metabolism predisposing to obesity and diabetes. Annu Rev Med 2011; 62:361 - 80; http://dx.doi.org/10.1146/annurev-med-012510-175505; PMID: 21226616
- Loesche WJ. Effect of bacterial contamination on cecal size and cecal contents of gnotobiotic rodents. J Bacteriol 1969; 99:520 - 6; PMID: 5808078
- Pleasants JR. Rearing germfree caesarean-born rats, mice, and rabbits through weaning. Ann N Y Acad Sci 1959; 78:116 - 26; http://dx.doi.org/10.1111/j.1749-6632.1959.tb53099.x; PMID: 14433463
- Strandberg K, Sedval G, Midtvedt T, Gustafsson BE. Effect of some biologically active amines on the cecum wall of germfree rats. Proc Soc Exp Biol Med 1966; 121:699 - 702; PMID: 5935571
- Gordon HA, Pesti L. The gnotobiotic animal as a tool in the study of host microbial relationships. Bacteriol Rev 1971; 35:390 - 429; PMID: 4945725
- Donowitz M, Binder HJ. Mechanism of fluid and electrolyte secretion in the GF rat cecum. Dig Dis Sci 1979; 24:551 - 9; http://dx.doi.org/10.1007/BF01489325; PMID: 456242
- Smith K, McCoy KD, Macpherson AJ. Use of axenic animals in studying the adaptation of mammals to their commensal intestinal microbiota. Semin Immunol 2007; 19:59 - 69; http://dx.doi.org/10.1016/j.smim.2006.10.002; PMID: 17118672
- Rasko DA, Rosovitz MJ, Myers GS, Mongodin EF, Fricke WF, Gajer P, et al. The pangenome structure of Escherichia coli: comparative genomic analysis of E. coli commensal and pathogenic isolates. J Bacteriol 2008; 190:6881 - 93; http://dx.doi.org/10.1128/JB.00619-08; PMID: 18676672
- Hungate RE. A Roll Tube Method for Cultivation of Strict Anaerobes. In: Norris JR, Ribbons DW, eds. Methods in Microbiology. New York: Academic Press, 1969: 117-132.