ABSTRACT
Ultramafic rocks are rare on Earth’s surface but can provide important insights into crust and mantle evolution. The recently discovered Broughton Arm Peridotite in the mountains of central Fiordland is exposed as two 75–150 m wide by several hundred metres-long harzburgite-dunite lobes encased sequentially in hornblendite, amphibolite and then quartzofeldspathic gneiss. On the basis of the refractory harzburgitic-dunitic composition and the bulk rock Mg# (86–87) lower than most mantle peridotites, the peridotite is interpreted to have an igneous cumulate rather than an exhumed mantle peridotite origin. The marginal hornblendite and amphibolite and internal pods of edenite-diopside rock may represent metasomatised peridotite. The Broughton Arm Peridotite has been metamorphosed twice. The first event generated an anhydrous assemblage comprising olivine (Mg# = 79.4–92.8), enstatite and Cr-magnetite. This assemblage has been overprinted by an anastomosing weak to pervasive mylonitic foliation of Mg-chlorite, tremolite ± serpentinite ± talc. The age of the anhydrous assemblage is unknown but suspected from regional data to be Early Carboniferous. A titanite 208Pb/238U lower intercept age of 105.6 ± 6.8 Ma in the host Deep Cove Gneiss is correlated with the peridotite hydration-deformation event. The proximity of the Early Cretaceous (≥ 114 Ma) Western Fiordland Orthogneiss to the east, west and north means that the Broughton Arm Peridotite and surrounding rocks may have recrystallised within the extensional Doubtful Sound Shear Zone, which elsewhere is an up to several hundred meter-wide shear zone active at c. 110–100 Ma separating Western Fiordland Orthogneiss from Deep Cove Gneiss. The Broughton Arm Peridotite therefore preserves a history that involves emplacement in the Early Paleozoic, penetrative anhydrous metamorphism in the Early Carboniferous, and hydration and recrystallisation during regional extensional tectonism in the Early Cretaceous.
Introduction
Ultramafic rocks comprise a minor portion of the New Zealand geology but have yielded important information about the evolution of portions of this continent. The Dun Mountain Ophiolite Belt, for example, is narrow but > 2000 km long unit (A) that represents a fragment of Permian oceanic lithosphere accreted to Gondwana (Coombs et al. Citation1976). The next largest ultramafic body, the 30 km long by < 1 km wide orogenic Anita Peridotite in Fiordland (A), has enabled characterisation of the composition of a Cretaceous sub-arc mantle lithosphere and also shows that vertical movements of > 30 km have taken place (Czertowicz, Scott, et al. Citation2016; Czertowicz, Scott, Waight, et al. Citation2016; Czertowicz, Toy, et al. Citation2016). Ultramafic peridotite intrusions, such as portions of the mid-Paleozoic metamorphosed Black Giants Anorthosite in Fiordland and the Riwaka Complex in northwest Nelson (A), attest to sub-arc mantle melting and the formation of cumulates (Gibson and Ireland Citation1999; Turnbull et al. Citation2017). Peridotite xenoliths have yielded significant insights, such as the Os and Hf isotopic data from xenoliths in Miocene alkaline basalts in Otago (A), which revealed that portions of the underlying mantle lithosphere are billions of years older than the overlying crust (McCoy-West et al. Citation2013; Scott, Waight, et al. Citation2014; Liu et al. Citation2015). The geochemistry of the New Zealand peridotite xenoliths also shows that the mantle lithosphere was widely enriched by carbonatitic melts (Scott, Hodgkinson, et al. Citation2014; Scott, Waight, et al. Citation2014; McCoy-West et al. Citation2015; Scott et al. Citation2016), with this modified reservoir possibly providing a mantle source to the widespread Cenozoic intraplate volcanism (van der Meer et al. Citation2017).
Figure 1. A, Satellite gravity map of the South Island of New Zealand showing some features discussed in the text. B, A simplified geological map of Fiordland (after Turnbull et al. Citation2010) summarising features discussed in the text.
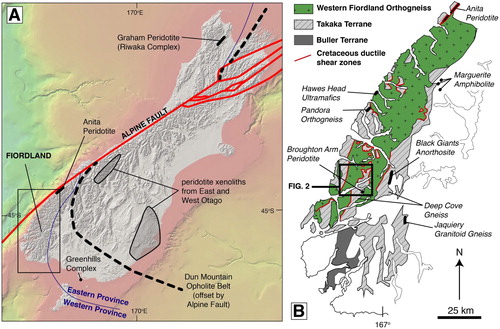
We expand the database on New Zealand ultramafic rocks by reporting a description of the Broughton Arm Peridotite in central Fiordland (A). This small body was identified during the Q-Map Fiordland mapping programme (Turnbull et al. Citation2010) but its origin, composition and evolution has not been studied. Through analysis of the peridotite petrology, coupled with U-Pb analysis of zircon and titanite in the host gneisses, this small body preserves a snapshot of probable Early Paleozoic emplacement, followed by multiple metamorphic events that have affected the western Fiordland crust.
Geological setting
The Broughton Arm Peridotite is located in western Fiordland in southwest New Zealand. Western Fiordland is part of the geological Western Province (Landis and Coombs Citation1967), which consists primarily of two terranes: the Late Cambrian–Silurian arc and sedimentary Takaka Terrane (Cooper Citation1989; Münker and Cooper Citation1999) and the Ordovician sedimentary Buller Terrane (Cooper Citation1989) (B) that have been variably invaded by igneous rocks. Most of the metasedimentary rocks of Western Fiordland are part of the Takaka Terrane. The largest meta-sedimentary unit within the Takaka Terrane in Western Fiordland is the Deep Cove Gneiss, which is a heterogeneous unit of pelites, psammites, calcareous rocks and amphibolites (Oliver Citation1980; Gibson Citation1982; Turnbull et al. Citation2010).
The rocks of Western Fiordland record multiple phases of magmatism over the last c. 500 Ma. At least two ∼500–490 Ma plutonic units (Jaquiery Granitoid Gneiss and Pandora Orthogneiss (Allibone, Jongens, Scott, et al. Citation2009; Allibone, Jongens, Turnbull, et al. Citation2009)) were emplaced into Deep Cove Gneiss (B). There was then a hiatus until the emplacement of Late Devonian to Carboniferous ultramafic through to felsic plutons (Kimbrough et al. Citation1994; Gibson and Ireland Citation1999; Muir et al. Citation1998; Allibone, Jongens, Scott, et al. Citation2009; Allibone, Milan, et al. Citation2009; Scott, Palin, et al. Citation2009; Tulloch et al. Citation2011). These represent a phase of continental arc-associated magmatism along the Gondwana margin (Gibson and Ireland Citation1999; Allibone, Jongens, Scott, et al. Citation2009; Allibone, Jongens, Turnbull, et al. Citation2009). U-Pb dating of metamorphic zircon and monazite in metasedimentary rocks indicates that this magmatic phase was accompanied by metamorphism that reached amphibolite facies conditions at ∼370–360 Ma then ∼330 Ma (Ireland and Gibson Citation1998; Daczko et al. Citation2009; Scott, Cooper, et al. Citation2009; Scott, Palin, et al. Citation2009; Schwartz et al. Citation2016).
Triassic to Jurassic rocks along the eastern side of Fiordland through the north and south of Fiordland represent a rejuvenation of Gondwana margin arc magmatism (Kimbrough et al. Citation1994; Muir et al. Citation1998; Scott and Palin Citation2008; Scott et al. Citation2008; Allibone et al. Citation2007; Allibone, Jongens, Turnbull, et al. Citation2009; Scott Citation2013). Magmatism then migrated inboard into western Fiordland and evolved into a high-flux state that resulted in emplacement of the Western Fiordland Orthogneiss. This unit comprises ultramafic to dioritic plutons outcropping over an area of c. 3000 km2 (B) and emplaced in the short period between ∼126 and 114 Ma (Oliver and Coggon Citation1979; Oliver Citation1980; Bradshaw Citation1989, Citation1990; Hollis et al. 2004; Allibone, Jongens, Turnbull, et al. Citation2009; Allibone, Milan, et al. Citation2009; Schwartz et al. Citation2017). The metamorphic history of the Western Fiordland Orthogneiss is complex, with the period 126–110 Ma involving regional moderate-P granulite facies metamorphism followed by ephemeral crustal thickening and dehydration to form garnet granulite to eclogite facies rocks (Bradshaw Citation1989; Brown Citation1996; Clarke et al. Citation2000; Daczko, Clarke, et al. Citation2001; Daczko, Klepeis, et al. Citation2001, Citation2002; De Paoli et al. Citation2009; Stowell et al. Citation2010, Citation2014). Although the Western Fiordland Orthogneiss contains rafts of metasedimentary rock, it is generally separated from metasedimentary rocks by ductile shear zones (B) (Gibson et al. Citation1988; Klepeis et al. Citation1999; Daczko et al. Citation2002; Klepeis et al. Citation2004; Scott and Cooper Citation2006; Klepeis et al. Citation2007; Betka and Klepeis Citation2013; Czertowicz, Scott, et al. Citation2016; Czertowicz, Toy, et al. Citation2016). The ≤ c. 110 Ma ductile shear zones are especially striking because they are often localised on calcareous horizons (Oliver Citation1980; Gibson et al. Citation1988; Scott and Cooper Citation2006; Negrini et al. Citation2018) and represent evidence for the initiation of thinning of the continental crust at the cessation of convergent magmatism (Gibson et al. Citation1988; Scott and Cooper Citation2006; Klepeis et al. Citation2007; Schwartz et al. Citation2016).
Fiordland contains a range of peridotitic rocks (B). The 30 km long Anita Peridotite is especially petrologically significant because it preserves a very rare insight into the composition and evolution of sub-arc mantle (Czertowicz, Scott, et al. Citation2016; Czertowicz, Scott, Waight, et al. Citation2016; Czertowicz, Toy, et al. Citation2016). Oliver (Citation1980) identified small hornblendite sills, interpreted to be metamorphosed ultramafic intrusions, within metasedimentary rocks now recognised as Deep Cove Gneiss in the mountains south of Doubtful Sound. Hornblende peridotite forms a portion of the metamorphosed stratiform Black Giants Anorthosite (Gibson Citation1979, Citation1982; Gibson and Ireland Citation1999) and probable Marguerite Amphibolite correlative (Scott, Palin, et al. Citation2009). The Early Cretaceous Western Fiordland Orthogneiss contains numerous hornblendite pods (Oliver Citation1980; Bradshaw Citation1990; Allibone, Jongens, Turnbull, et al. Citation2009), the largest of which encloses cumulate peridotite and is known as the Hawes Head Ultramafics (Daczko et al. Citation2012).
Methods
Mineral compositions in the Broughton Arm Peridotite were measured using energy-dispersive spectroscopy (EDS) on the University of Otago Zeiss Sigma Variable Pressure Field Emission Gun scanning electron microscope. The settings were an accelerating voltage of 15 kV and a 2.7 nA beam current, with an live time of > 30 s. Standardisation involved using Smithsonian natural reference materials: hornblende (USNM143965) for Na and Ti, Cr-augite (USNM164905) for Al, Si and Ca, microcline (USNM143966) for K, olivine (USNM444) for Mg, fayalite for (USNM85276) Mn and hypersthene (USNM746) for Fe.
Samples selected for whole rock analysis were first trimmed using a diamond saw, followed by sanding to remove potential saw-blade contamination. Samples were enclosed inside several plastic bags and smashed to rock chips, then further reduced in size in an agate rock mill. Spectrachem Analytical, New Zealand, then measured the powders on lithium tetraborate glass disks containing 0.5 grams of homogenised powder. Trace elements were measured on dried powders (110°C) converted to pressed powder pellets comprising 7.0 grams of powder. Elements were measured on a PANalytical 2404 X-ray fluorescence vacuum spectrometer equipped with a 4 kW Rh super sharp X-ray tube. Loss on ignition was calculated by weighing aliquots before and after being subjected to 1000°C for one hour.
Zircon grains were separated at California State University Northridge by pulverising the samples with a jaw crusher and disk mill and then undertaking density separation using a Wilfley table and heavy liquids. Grains were mounted in epoxy and polished and then imaged on a Gatan MiniCL detector attached to a FEI Quanta 600 scanning electron microscope. Analyses were conducted at the University of California Santa Barbara using a Nu Plasma multi-collector inductively coupled plasma mass spectrometer (MC-ICPMS) with a Photon Machines 193 ArF excimer laser with HelEx cell. The spot size for all analyses was ∼35 microns and the repetition rate was 4 Hz. Primary standard 91500 was analysed every 10 analyses. Uranium-Pb ratios were collected in one analytical session in November 2017 following methods described in Kylander-Clark et al. (Citation2013). U–Pb isotope ratios and their uncertainties were calculated using Iolite 3.0 (Paton et al. Citation2010). A typical ablation of 12–26 ng of material yields 2σ standard errors of 1–1.5% for 206Pb/238U and 0.3–1% on 207Pb/206Pb using down-hole elemental-fractionation correction (Paton et al. Citation2011). Temora-2 and GJ-1 standards were also analysed throughout the analytical session and yielded concordant results with 206Pb/238U ages of 417.1 ± 4.8 (n = 7; mswd = 1.2) and 601.3 ± 3.8 (n = 9; mswd = 0.5), respectively. The quoted dates in the text and tables are reported at 95% confidence interval and are assigned 2% total uncertainties, except when comparing analyses within the same sample or grain.
Methods for titanite geochronology and Zr geothermometry also closely follow those outlined in Kylander-Clark et al. (Citation2013). BHVO-2g was analysed as a compositional standard for trace element concentrations, and BIR was analysed as the primary standard for U and Pb isotope measurements. Samples were ablated in the thin section. The laser spot diameter was ∼41 μm for titanite. The laser was fired twice with a spot size of 53 μm to remove common Pb from the sample surface followed by a sample chamber wash out of 15 s. Titanite grains were ablated at 4 Hz for 20 s. Masses 204Pb + Hg, 206Pb, 207Pb, and 208Pb were measured on ion counters, and masses 232Th and 238U were measured on Faraday detectors. MKED (1517.3 Ma: Spandler et al. Citation2016) was analysed as a secondary standard. Data were reduced using Iolite (Paton et al. Citation2010). Uncertainty of individual 206Pb/238U measurements is dominated by counting statistics and signal stability. An additional 2% external error is added in quadrature to 207Pb/206Pb measurements to account for variation in ablation or transport characteristics. Isotopic ratios for each analysis are plotted on Tera-Wasserburg diagrams using Isoplot (Ludwig, Citation2012). All dates are reported at 95% confidence interval and are assigned 2% combined systematic and analytical uncertainties, which correspond to total errors of ∼ 2.0–2.5 Ma.
Broughton Arm Peridotite
The Broughton Arm Peridotite occurs at an elevation of 520–620 metres above sea level on the southern side of Broughton Arm in Breaksea Sound (A). The outcrop occurs on a ridgeline (B, ) and is exposed over an area of approximately 500 m by up to 75–150 m wide. It consists of two red-weathering ultramafic lobes enclosed in a thin zone of near-mono-mineralic hornblendite that is in turn enclosed in amphibolite (, A). Gneissic quartzofeldspathic metasedimentary horizons occur to the west of the amphibolite; a steep cliff on the eastern side prohibited examination of the rocks that occur to the east. Turnbull et al. (Citation2010) noted a small patch of peridotite on the shoreline on the north side of Broughton Arm, and our own telephoto images and a helicopter flyby indicate that peridotite likely occurs along strike to north on the ridgeline between Broughton Arm and Vancouver Arm (A).
Figure 2. A, Geological map of the Broughton Arm area. B, Aerial photograph of Broughton Arm Peridotite looking to the southeast.
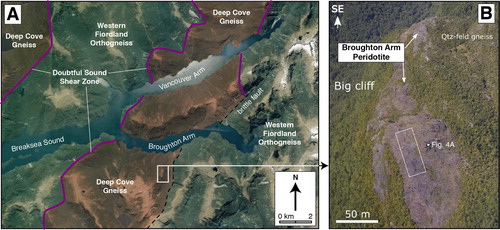
Figure 3. Geological map of the Broughton Arm Peridotite, associated rocks, and sample locations. The quartzofeldspathic gneisses samples for zircon U-Pb are from slightly southwest of the area. This map corresponds to the field of view in .
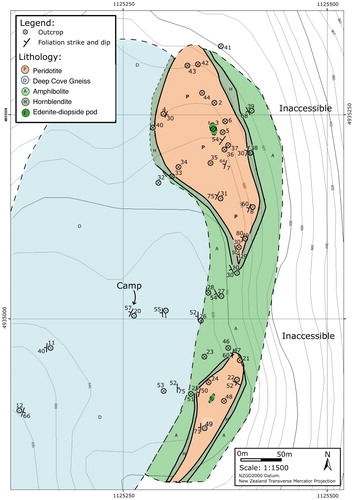
Figure 4. A, The Broughton Arm Peridotite is a distinctly orange-red body enclosing zoned patches of edenite-clinopyroxenite. The mountains in the background are Western Fiordland Orthogneiss and Broughton Arm fjord is present in the left hand side. See B for location of photograph. Hand specimens of the peridotite illustrate the variation in textural types from foliated and massive peridotite (B) to mylonitic peridotite (C).
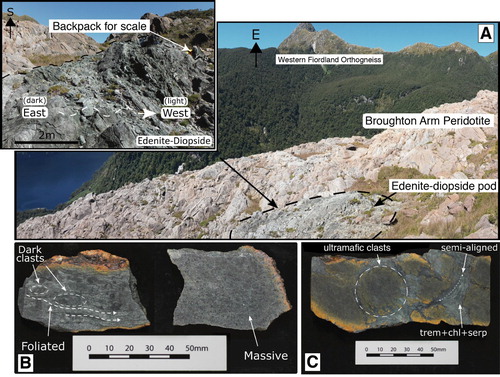
Peridotite
The ultramafic lobes have a rusty-red rind approximately 3 mm thick that, when removed, reveals a blue-greenish colour. The rocks vary from massive to deformed (B–C), with the more deformed types being paler in colour and typically containing clasts (up 5 cm) of the massive type. Massive peridotite is dominated by olivine with minor orthopyroxene, magnetite and clinopyroxene (A). These minerals are commonly wrapped by a fine-grained groundmass dominated by tremolite and chlorite with minor serpentine, with the proportions of these hydrous phases increasing with the degree of foliation in the peridotite (C, B). Back-scattered electron images show that the olivine grains are fractured, with the fractures containing chlorite, serpentinite and magnetite (C). Minor phases identified include talc (on the margins of orthopyroxene) and smectite.
Figure 5. A, and B, show cross-polarised light microscopic images of the anhydrous (BP5A, BP40B) and hydrous (B, BP22A) peridotites. C, Backscattered electron image clearly show the replacement of olivine by chlorite, serpentine and magnetite (BP24A). D, Cross-polarised image of a mylonitic quartzofeldspathic gneiss (BP1A).
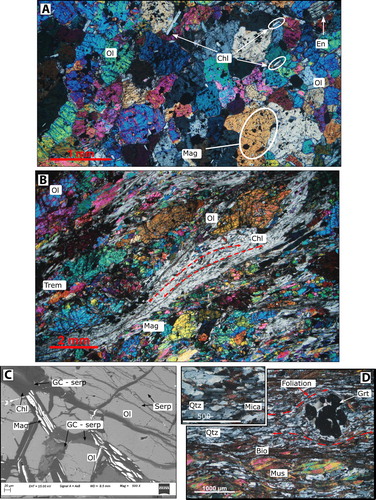
Quantitative EDS analyses show olivine within 10 peridotite samples to be dominated by the forsterite end-member, with Mg# (= 100*Mg/(Mg + Fe)) of 79.4–91.2 (). There is no obvious correlation between Mg# and spatial distribution across the peridotite. Orthopyroxene grains are enstatite and confirmed to contain no Cr, but the quantitative analyses are not of sufficient quality to be published. Oxides are dominantly magnetite with variable Cr composition (). Sample 24A is highlighted due to its extreme oxide Cr range; magnetite grains enclosed within olivine contain the highest Cr values measured (up 15 wt % Cr2O3) with the majority within the serpentinised groundmass having 0%. Clinopyroxene is an uncommon phase and found only in BP3A and BP24A. Tremolite is abundant in the groundmass that wraps around the porphyroclasts and has a fairly uniform composition () except for BP24F, which contains ∼ 0.5 wt% Cr2O3. Chlorite is Mg-rich (up to 33.9 wt.%) and contains variable amounts of Cr2O3. Serpentinite is predominantly associated with olivine, but also occurs as interstitial fibres within the tremolite-chlorite-dominated groundmass. Semi-quantified compositions of mineral phases not presented in can be provided upon request.
Table 1. Electron microbeam analytical data.*
The bulk rock composition was obtained for three peridotite samples (2A, 8A, 34B). 8A displays little or no hydrous phases and has a LOI of 1.8 wt%, whereas 2A and 34A have LOI values of 3.0 and 4.5, respectively (). From least to most hydrated, SiO2 varies from 41.5–43.1 wt. %, MgO from 41.0 to 31.5 wt.%, Fe2O3 from 12.7 – 9.9 wt.%, Al2O3 from 1.8 to 4.7 wt% and CaO from 0.1 to 5.5 wt%. After multiplying Fe2O3 by 0.8998 to convert it to FeO, the calculated bulk rock Mg# (100*Mg/(Mg + Fe2+)) covers a small range from 86 to 87. In detail, this recalculation may under-estimate the Mg#, since Fe may was unlikely to have only been in ferrous valency; however, this recalculation provides an opportunity to compare with global data. Other notable chemical features are the very low concentrations of trace elements except for Cr (6040 to 2566 ppm) and Ni (2090 to 1259 ppm).
Table 2. Whole rock X-ray fluorescence data.
Edenite-diopside lobes
Amphibole-clinopyroxene pods ranging in diameter from 0.2 m to 20 m occur inside the ultramafic body (, A). They are green, zoned, and transition into the meta-peridotite over several metres. The darker green rock on the eastern side is dominated by clinopyroxene with interstitial amphibole, which EDS analysis shows to be diopside and edenite (). A low SiO2 content and the occurrence of minor Cr2O3 (< 0.5 wt.%) in the edenite distinguishes it from tremolite in the meta-peridotite rocks. The large diopside grains (< 4 mm) exhibit prominent exsolution lamellae of magnetite and an unidentified silica-rich phase that may be orthopyroxene. Smaller diopside grains in the groundmass lack the exsolution.
Hornblendite
A rim of 5–10 m wide pale green hornblendite encases the peridotite body (). The contact between the meta-peridotite and hornblendite is complex and involves intercalated layers over several metres. The hornblendite on the eastern side of the ultramafic body is a light green colour with strongly aligned tabular grains < 1 mm in length. This hornblendite consists of highly aligned hornblende and interstitial muscovite mica. On the western side of the ultramafic body the hornblendite rim is a darker green colour and exhibits a high concentration of irregular fine grained quartz-clinozoisite veins intruding orthogonal to the foliation. The grain size of the clinozoisite is no more than 20 μm, whereas the quartz varies from 20 μm within the vein to 200 μm along the edges.
Amphibolite
Pale green amphibolite separates hornblendite from the quartzofeldspathic gneisses of the Deep Cove Gneiss (). The texture and mineralogy of the weakly foliated amphibolite varies with distance to the hornblendite. For example, the transition from amphibolite to hornblendite is marked by the loss of plagioclase over several m. Away from the hornblendite, the mineralogy is relatively uniform and is dominated by aligned feldspar and hornblende grains accompanied by titanite. The tabular hornblende grains demonstrate a strong alignment at micro-scale, and range in size from 1 to 2 mm. Plagioclase occurs as both interstitial grains in between the hornblende grains and in plagioclase veins. Smaller interstitial grains (< 0.4 mm) are commonly twinned, in contrast to the larger interstitial grains (< 1 mm).
Quartzofeldspathic Gneiss
Quartzofeldspathic and amphibolitic gneisses forms the largest unit in the field area and occurs to the west of the meta-peridotite (). It is part of the Deep Cove Gneiss (Turnbull et al. Citation2010). It grades into banded garnet-bearing amphibolite-hornblendite gneiss to the southwest (not described in this article). The quartzofeldspathic gneiss is composed of alternating pale quartz-rich and brown mica-rich layers and is strongly foliated to mylonitic. Minerals observed include quartz + muscovite + biotite + titanite + garnet + zircon ± feldspar. The muscovite and biotite grains have ‘mica fish’ shapes, and the garnets are heavily fractured, have asymmetric tails and quartz strain shadows and are therefore porphyroclasts (D). The amphibolites contain hornblende, plagioclase, quartz, ilmenite, titanite and zircon.
Zircon and titanite U-Pb dates & zircon thermometry
Zircon was extracted from three gneisses (1B, 16A and 20A; ) with the purpose of identifying metamorphic overgrowths and dating them. Although no overgrowths could be located, the data do enable some inferences about the depositional age of these metasedimentary rocks. The 207Pb-corrected 207Pb/206Pb dates span 580.7 to 2390 Ma (n = 19) in BP1B, 543.0 to 1090.0 Ma (n = 9) in BP16A and 560.6 to 1186.0 (n = 7) in BP20A (Supplementary Table 1). A concordia plot of the compiled data shows that populations cluster at ∼580 Ma and ∼1000 Ma (A). These population ages match those of almost all detrital zircon spectra elsewhere in the Takaka Terrane and correspond to detritus from the Ross-Delamerian and Grenville orogens (e.g. Adams et al. Citation2015). The youngest populations constrain an upper age of deposition to after 580 Ma, and this too is consistent with correlation of the rocks to the Takaka Terrane.
Figure 6. A, Zircon U-Pb ages plotted on a concordia plot show the occurrence of c. 580 and 1100 Ma detrital populations. Note that three samples are plotted together. B, A discordia array enables calculation of a 105.6 ± 6.8 Ma population from titanite (n = 34 individual grains). Solid ellipses are data used to calculate the Cretaceous population and dashed ellipses are the remaining data. C, A plane polarised light microscopic image showing the distribution of titanite in BP18A.
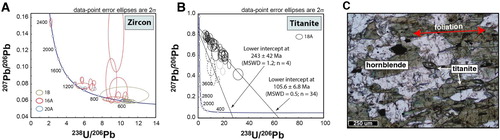
Titanite was extracted from amphibolitic gneiss BP18A. The data, as is common for titanite, are extremely discordant due to the high levels of common-Pb (B; Supplementary Table 2). However, the analyses commonly have sufficient spread in 238U/206Pb and 207Pb/206Pb to define an isochron without the need to assume a common 207Pb/206Pb value from a model (e.g. Stacey and Kramers Citation1975) or from a low-U mineral presumed to be in equilibrium with titanite. Projection of a discordia array through 34 analyses gives a lower intercept at 105.6 ± 6.8 Ma (mean squared weighted deviation of 0.5). The titanite grains form part of the foliation to the rock (C). If Zr and SiO2 saturation is assumed as indicated by the co-existence of zircon and quartz, this population gives a very tight Zr-in-titanite temperature (Hayden et al. Citation2008) of 633 ± 16°C at 0.6 GPa or 675 ± 18°C at 1.0 GPa (errors are 2 standard deviations). These pressures were chosen to reflect the published range of pressures inferred for Fiordland Cretaceous metamorphic rocks (e.g. Bradshaw Citation1989). A second array indicates a population of 243 ± 43 Ma, but the limited data makes the significance of this 4-grain population unclear.
The association of U-Pb dates to Zr-in-titanite temperatures is an important consideration in understanding titanite systematics. Experimental studies indicate that volume diffusion of Zr in titanite is approximately 2 orders of magnitude slower than Pb (Hayden et al. Citation2008). In our sample, Zr-in-titanite temperatures are ∼633–675°C, which are at or below the Pb volume diffusion closure temperature and well below temperatures at which Zr is expected to experience significant volume diffusion (e.g. Schwartz et al. Citation2016). We therefore conclude that Zr concentrations and Zr-in-titanite temperatures are probably linked to U-Pb dates in this rock. In another study of titanite from high-grade Fiordland rocks, Schwartz et al. (Citation2016) concluded that variations in U-Pb titanite dates were not due to thermally activated volume-diffusion and that they indicate preservation of complex crystallization histories.
Discussion
Protolith to the peridotite and associated rocks
The general lack of clinopyroxene and the small amount of orthopyroxene but abundance of olivine means that peridotite components to the Broughton Arm Peridotite have harzburgitic to dunitic compositions. Dunitic lithologies occur in depleted mantle peridotite suites but they can also be magmatic (or metamorphosed magmatic) rocks (e.g. Szilas et al. Citation2015). The occurrence of high bulk rock Cr (> 2500 ppm) and Ni (> 1200 ppm) coupled with common although not ubiquitous olivine Mg# > 89 means that the Broughton Arm Peridotite could be a metamorphosed mantle peridotite (e.g. Pearson et al. Citation2014). However, the low bulk rock Mg# and high Mg/Si clearly distinguish it from the general melting trajectory for global mantle peridotites, as well as from the Anita (orogenic) Peridotite in Fiordland and the ultramafic base of the Dun Mountain Ophiolite Belt (A). A further complication is that metamorphism of mantle olivine under oxidising conditions will altered the valance of Fe; because olivine does not take Fe3+ within its crystal lattice, recrystallised olivine in this environment will become more Mg-rich and the Fe3+ will enter magnetite with the result being a high olivine Mg#. Since magnetite is abundant, then it is quite possible that the olivine Mg# has increased during metamorphism. Basaltic liquids that undergo extensive fractionation can generate Mg-rich ultramafic cumulates that are high in Cr and Ni. Such igneous rocks commonly have lower bulk rock Mg# than mantle peridotite since the igneous rocks were liquids whereas peridotite in the mantle is a partial melting residue. The least altered Broughton Arm Peridotite sample, BP8A, is distinct from the Hawes Head Ultramafics in Fiordland or the Graham Peridotite (part of the Riwaka Complex in northwest Nelson) but is broadly similar to dunitic cumulates from the Permian arc-related Greenhills Complex (, ). While the bulk chemistry does not rule out the Broughton Arm Peridotite being an exhumed fragment of mantle, if it was then it records the unusual process of having experienced extreme enrichment in Fe but little else. It seems more likely that the ultramafic body is a metamorphosed ultramafic cumulate magma. Globally, cumulates with high MgO relative to FeO and Na2O + K2O are indicative of arc-related melting (Beard Citation1986).
Figure 7. Comparison of bulk rock properties of the Broughton Arm Peridotite with global mantle xenoliths (Pearson et al. Citation2014), Anita Peridotite (Czertowicz, Scott, Waight, et al. Citation2016), peridotite at the base of the Dun Mountain Ophiolite Belt (Stewart et al. Citation2016; Scott unpublished data), Greenhills Complex (Spandler et al. Citation2003), Hawes Head Ultramafics peridotite (Daczko et al. Citation2012) and Graham Peridotite (Turnbull et al. Citation2017). Fe2O3 in all samples was multiplied by 0.8999 to give FeO.
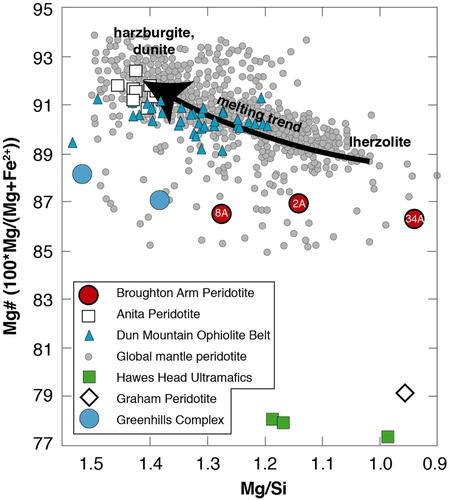
Metamorphic history and timing of emplacement
Although metamorphism of the Broughton Arm Peridotite likely affected olivine protolith compositions by making them more Mg-rich whilst forming magnetite, there are other prominent metamorphic changes. Steep chemical gradients at the interface between Mg-rich ultramafic and adjacent siliceous rocks during metamorphism result in formation of hybrid lithologies. An excellent New Zealand example is the tremolite-fuchsite rim to a meta-gabbroic pod enclosed in siliceous Haast Schist (Cooper Citation1995). In the Broughton Arm Peridotite, the thin horizon of hornblendite and then amphibolite that separate the harzburgite and dunite from the quartzofeldspathic (and hence silica-rich) gneisses may also be metasomatic since this lithological configuration shows an increase in SiO2 with distance from the peridotites. The edenite-diopside pods within the ultramafic body (A). may also represent zones of metasomatised peridotite.
The texturally earliest identifiable metamorphic event in the Broughton Arm Peridotite is recorded in the relict patches of olivine-enstatite-magnetite-tremolite in the peridotitic core, which typically display triple point boundaries (B). This assemblage has been variably overprinted by an episode of fluid infiltration, which resulted in the widespread formation of Mg-chlorite, tremolite and serpentinite (C) during formation of a weak to mylonitic fabric (D, 5B). This fabric is parallel to the pervasive gneissic to mylonitic fabric in the surrounding Deep Cove Gneiss (e.g. C). Titanite forming part of the gneiss foliation yielded a U-Pb age of 105.6 ± 6.8 Ma, and therefore this age is interpreted to also date the formation of the hydrous assemblages in the peridotite. Zr-in-titanite saturation temperatures (Hayden et al. Citation2008) are 633°C to 675°C for 0.6 GPa or 1.0 GPa, respectively. Regardless of the actual pressure of recrystallisation – which cannot be defined from the mineral assemblages – the hydrous foliation in the peridotite most likely formed at amphibolite facies conditions during ductile deformation in the Early Cretaceous.
An important feature of the studied area is the proximity (∼500 m) to the Western Fiordland Orthogneiss (Allibone, Jongens, Turnbull, et al. Citation2009; Allibone, Milan, et al. Citation2009; Turnbull et al. Citation2010) (). The contact between the granulite facies Western Fiordland Orthogneiss and the dominantly metasedimentary Deep Cove Gneiss is usually a several hundred-meter thick decollement localised on a calcareous horizon in the Deep Cove Gneiss (Oliver and Coggon Citation1979) known as the Doubtful Sound Shear Zone (A) (Gibson et al. Citation1988; Klepeis et al. Citation2007). This shear zone and related structures elsewhere in Fiordland were the sites of extensive fluid mobility at the cessation of arc magmatism and the initiation of regional extension at c. 110–100 Ma (Gibson et al. Citation1988; Scott and Cooper Citation2006; Klepeis et al. Citation2007; Schwartz et al. Citation2016). Schwartz et al. (Citation2016) found that titanite in a Doubtful Sound Shear Zone calc-mylonite from Vancouver Arm ∼4 km from the Broughton Arm Peridotite contained an inherited 330.1 ± 6.6 Ma population and fabric-dating 104.9 ± 7.4 Ma population, and another nearby calc-mylonite yielded dates from 111.4 to 107.6 Ma (). Therefore, considering the present proximity of the Broughton Arm Peridotite to the Western Fiordland Orthogneiss, the 105.6 ± 6.8 Ma age of the Broughton Arm Peridotite hydrous fabric, and the associated infiltration of fluid is interpreted to show that the recrystallisation of the peridotite and associated gneisses was associated with the Doubtful Sound Shear Zone. It should be noted that calc-mylonites have not been mapped to occur east of the Broughton Arm Peridotite; instead, a brittle fault is inferred to separate the Western Fiordland Orthogneiss and Deep Cove Gneiss. This young brittle fault may have excised the calc-mylonites from this region, leaving little prominent field evidence for the Doubtful Sound Shear Zone. An alternative is that the hydrous recrystallisation in the Broughton Arm Peridotite is associated with the aureole of the Western Fiordland Orthogneiss. However, this unit has an igneous protolith zircon emplacement age of 116.4 ± 1.3 Ma in Vancouver Arm () (Schwartz et al. Citation2017), which means that the Deep Cove Gneiss titanite U-Pb ages are too young to be associated with Western Fiordland Orthogneiss emplacement.
Figure 8. This oblique Google Earth-derived image shows the location of the Broughton Arm Peridotite, the Deep Cove Gneiss, the Western Fiordland Orthogneiss (WFO) and the Doubtful Sound Shear Zone (DSSZ). Note that the boundary between the Western Fiordland Orthogneiss and the Deep Cove Gneiss in the east of the Broughton Arm (BA) is mapped as a brittle fault by Turnbull et al. (Citation2010); this structure is younger than the ductile Doubtful Sound Shear Zone, and appears to have excised the shear zone. Ultramafic lithologies to the north have been located on the shoreline (Turnbull et al. Citation2010) and inferred by us from telephoto images and a helicopter flyby. Stars indicate proximal locations of published information discussed in more detail in the text; data are from 1, Allibone, Milan, et al. (Citation2009), 2, Schwartz et al. (Citation2016), 3, Schwartz et al. (Citation2017).
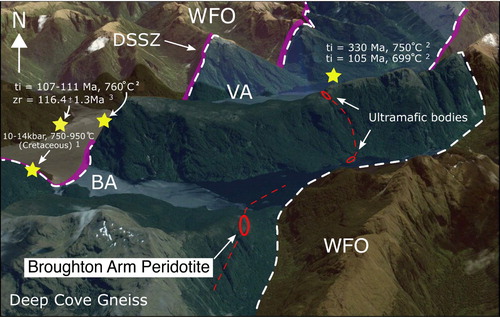
If the hydrous assemblage in the Broughton Arm Peridotite formed at c. 106 Ma, then the anhydrous olivine-enstatite-Cr-magnetite assemblage must be older. A further significance of the nearby calc-mylonite with bimodal ∼330 and 105 Ma titanite populations established by Schwartz et al. (Citation2016) is that the region has evidently experienced Carboniferous metamorphism. Dating of metamorphic monazite dating of Deep Cove Gneisses has also revealed Carboniferous recrystallisation (Ireland and Gibson Citation1998). Our poor four-grain 243 ± 43 Ma titanite population in the quartzofeldspathic gneiss surrounding the peridotite (B) could therefore represent mixing of Carboniferous metamorphic domains with Cretaceous titanite domains during ablation. In this case, the anhydrous metamorphism that formed the olivine-enstatite-magnetite assemblage in the peridotite requires that the protolith to the peridotite was emplaced even earlier. The new detrital ages of zircons in the host gneisses require peridotite emplacement after 580 Ma, although, in detail, Western Fiordland metasedimentary gneisses were mostly deposited ∼500–480 Ma (Gibson and Ireland Citation1999; Hollis et al. 2004; Scott, Cooper, et al. Citation2009) and therefore 500 Ma is a more probably upper limit. Other Paleozoic ultramafic intrusions in Fiordland include the Black Giants Anorthosite, which contains meta-peridotite and has been dated to have been emplaced at 349 ± 5 Ma during arc magmatism (Gibson and Ireland Citation1999), and the Marguerite Amphibolite, which was emplaced before metamorphism at 340–350 Ma (Scott, Palin, et al. Citation2009) (B). The Broughton Arm Peridotite is therefore interpreted to preserve evidence for Paleozoic emplacement of a ultramafic cumulate probably during mid Paleozoic arc magmatism, followed by Devonian metamorphism during region-wide recrystallisation, and then hydration and recrystallisation during Early Cretaceous ductile deformation at the collapse of the Cretaceous arc magmatism.
Conclusions
The Broughton Arm Peridotite adds to the growing database of ultramafic rocks in New Zealand. Composed of several variably metamorphosed and recrystallised rocks outcropping over an area of 500 m (although it may extend several km to the north), it provides a record of the complex igneous and metamorphic history of central Fiordland from the mid-Paleozoic to the Cretaceous. The protolith to the Broughton Arm Peridotite is most likely to have been an ultramafic cumulate magma emplaced in the early to mid Paleozoic. A first metamorphic event, probably in the Carboniferous at c. 330 Ma, formed olivine–enstatite–Cr magnetite-dominated dunite and harzburgite assemblages. The margins of the peridotite have been subsequently converted to near mono-mineralic hornblendite and then amphibolite, and the harzburgitic-dunitic core has partially recrystallised to Mg-chlorite, tremolite, serpentinite-dominated assemblages. The growth of the hydrous phases was associated with grain size reduction, and in-situ titanite U-Pb dating of deformed host gneisses indicates that this amphibolite facies assemblage formed c. 106 Ma concurrent with deformation along the extensional Doubtful Sound Shear Zone.
Supplementary tables
Download MS Excel (53.9 KB)Acknowlegments
Mo Turnbull is thanked for initial information on the topic. The Bob Carter Memorial Fund supported helicopter travel via Te Anau Helicopters. Financial support for zircon and titanite geochemical analysis was provided by NSF grant EAR-1352021 to Schwartz, with analytical support from Andrew Kylander-Clark. The Department of Conservation permitted sampling. Marco Brenna commented on a manuscript draft and we appreciate John Gamble and Tod Waight for reviewing the text.
Disclosure statement
No potential conflict of interest was reported by the authors.
Additional information
Funding
References
- Adams CJ, Mortimer N, Campbell HJ, Griffin WL. 2015. Detrital zircon ages in Buller and Takaka terranes, New Zealand: constraints on early Zealandia history. New Zealand Journal of Geology and Geophysics. 58:176–201. doi: 10.1080/00288306.2015.1025798
- Allibone AH, Jongens R, Scott JM, Tulloch AJ, Turnbull IM, Cooper AF, Powell NG, Ladley EB, King RP, Rattenbury MS. 2009. Plutonic rocks of the Median Batholith in eastern and central Fiordland, New Zealand: field relations, geochemistry, correlation, and nomenclature. New Zealand Journal of Geology and Geophysics. 52:101–148. doi: 10.1080/00288300909509882
- Allibone AH, Jongens R, Turnbull IM, Milan LA, Daczko NR, De Paoli MC, Tulloch AJ. 2009. Plutonic rocks of Western Fiordland, New Zealand: field relations, geochemistry, correlation, and nomenclature. New Zealand Journal of Geology and Geophysics. 52:379–415. doi: 10.1080/00288306.2009.9518465
- Allibone AH, Milan LA, Daczko NR, Turnbull IM. 2009. Granulite facies thermal aureoles and metastable amphibolite facies assemblages adjacent to the Western Fiordland Orthogneiss in southwest Fiordland, New Zealand. Journal of Metamorphic Geology. 27:349–369. doi: 10.1111/j.1525-1314.2009.00822.x
- Allibone AH, Turnbull IM, Tulloch AJ, Cooper AF. 2007. Plutonic rocks of the Median Batholith in southwest Fiordland, New Zealand: field relations, geochemistry, and correlation. New Zealand Journal of Geology and Geophysics. 50:283–314. doi: 10.1080/00288300709509838
- Beard JS. 1986. Characteristic mineralogy of arc-related cumulate gabbros: implications for the tectonic setting of gabbroic plutons and for andesite genesis. Geology. 14:848–851. doi: 10.1130/0091-7613(1986)14<848:CMOACG>2.0.CO;2
- Betka PM, Klepeis KA. 2013. Three-stage evolution of lower crustal gneiss domes at Breaksea entrance, Fiordland, New Zealand. Tectonics. 32:1084–1106. doi: 10.1002/tect.20068
- Bradshaw JY. 1989. Origin and metamorphic history of an early Cretaceous polybaric granulite terrain, Fiordland, southwest New Zealand. Contributions to Mineralogy and Petrology. 103:346–360. doi: 10.1007/BF00402921
- Bradshaw JY. 1990. Geology of crystalline rocks of northern Fiordland: details of the granulite facies Western Fiordland Orthogneiss and associated rock units. New Zealand Journal of Geology and Geophysics. 33:465–484. doi: 10.1080/00288306.1990.10425702
- Brown EH. 1996. High-pressure metamorphism caused by magma loading in Fiordland, New Zealand. Journal of Metamorphic Geology. 14:441–452. doi: 10.1046/j.1525-1314.1996.06024.x
- Clarke GL, Klepeis KA, Daczko NR. 2000. Cretaceous high-P granulites at Milford Sound, New Zealand: metamorphic history and emplacement in a convergent margin setting. Journal of Metamorphic Geology. 18:359–374. doi: 10.1046/j.1525-1314.2000.00259.x
- Coombs DS, Landis CA, Norris RJ, Sinton JM, Borns DJ, Craw D. 1976. The Dun Mountain ophiolite belt, New Zealand, its tectonic setting, constitution, and origin, with special reference to the southern portion. American Journal of Science. 276:561–603. doi: 10.2475/ajs.276.5.561
- Cooper AF. 1995. Nephrite and metagabbro in the Haast Schist at Muddy Creek, Northwest Otago, New Zealand. New Zealand Journal of Geology and Geophysics. 38:325–332. doi: 10.1080/00288306.1995.9514660
- Cooper RA. 1989. Early Paleozoic terranes of New Zealand. Journal of the Royal Society of New Zealand. 19:73–112. doi: 10.1080/03036758.1989.10426457
- Czertowicz TA, Scott JM, Piazolo S. 2016. Coupled extrusion of sub-arc lithospheric mantle and lower crust during orogen collapse: a case study from Fiordland, New Zealand. Journal of Metamorphic Geology. 34:501–524. doi: 10.1111/jmg.12191
- Czertowicz TA, Scott JM, Waight TE, Palin JM, van der Meer QHA, Le Roux P, Münker C, Piazolo S. 2016. The Anita Peridotite, New Zealand: ultra-depletion and subtle enrichment in sub-arc mantle. Journal of Petrology. 57:717–750. doi: 10.1093/petrology/egw001
- Czertowicz TA, Toy VG, Scott JM. 2016. Recrystallisation, phase mixing and strain localisation in peridotite during rapid extrusion of sub-arc mantle lithosphere. Journal of Structural Geology. 88:1–19. doi: 10.1016/j.jsg.2016.04.011
- Daczko NR, Clarke GL, Klepeis KA. 2001. Transformation of two-pyroxene hornblende granulite to garnet granulite involving simultaneous melting and fracturing of the lower crust, Fiordland, New Zealand. Journal of Metamorphic Geology. 19:549–562. doi: 10.1046/j.0263-4929.2001.00328.x
- Daczko NR, Emami S, Allibone AH, Turnbull IM. 2012. Petrogenesis and geochemical characterisation of ultramafic cumulate rocks from Hawes Head, Fiordland, New Zealand. New Zealand Journal of Geology and Geophysics. 55:361–374. doi: 10.1080/00288306.2012.719910
- Daczko NR, Klepeis KA, Clarke GL. 2001. Evidence of early Cretaceous collisional-style orogenesis in northern Fiordland, New Zealand and its effects on the evolution of the lower crust. Journal of Structural Geology. 23:693–713. doi: 10.1016/S0191-8141(00)00130-9
- Daczko NR, Klepeis KA, Clarke GL. 2002. Thermomechanical evolution of the crust during convergence and deep crustal pluton emplacement in the Western Province of Fiordland, New Zealand. Tectonics. 21:4–18 p. doi: 10.1029/2001TC001282
- Daczko NR, Milan LA, Halpin JA. 2009. Metastable persistence of pelitic metamorphic assemblages at the root of a Cretaceous magmatic arc - Fiordland, New Zealand. Journal of Metamorphic Geology. 27:233–247. doi: 10.1111/j.1525-1314.2009.00815.x
- De Paoli MC, Clarke GL, Klepeis KA, Allibone AH, Turnbull IM. 2009. The eclogite–granulite transition: Mafic and intermediate assemblages at Breaksea Sound, New Zealand. Journal of Petrology. 50:2307–2343. doi: 10.1093/petrology/egp078
- Gibson GM. 1979. Margarite in kyanite- and corundum-bearing anorthosite, amphibolite, and hornblendite from central Fiordland, New Zealand. Contributions to Mineralogy and Petrology. 68:171–179. doi: 10.1007/BF00371898
- Gibson GM. 1982. Stratigraphy and petrography of some metasediments and associated intrusive rocks from central Fiordland, New Zealand. New Zealand Journal of Geology and Geophysics. 25:21–43. doi: 10.1080/00288306.1982.10422503
- Gibson GM, Ireland TR. 1999. Black giants Anorthosite, New Zealand: a Paleozoic analogue of Archean stratiform anorthosites and implications for the formation of Archean high-grade gneiss terranes. Geology. 27:131–134. doi: 10.1130/0091-7613(1999)027<0131:BGANZA>2.3.CO;2
- Gibson GM, McDougall I, Ireland TR. 1988. Age constraints on metamorphism and the development of a metamorphic core complex in Fiordland, southern New Zealand. Geology. 16:405–408. doi: 10.1130/0091-7613(1988)016<0405:ACOMAT>2.3.CO;2
- Hayden LA, Watson EB, Wark DA. 2008. A thermobarometer for sphene (titanite). Contributions to Mineralogy and Petrology. 155:529–540. doi: 10.1007/s00410-007-0256-y
- Hollis JA, Clarke GL, Klepeis KA, Daczko NR, Ireland TR. 2003. Geochronology and geochemistry of high-pressure granulites of the Arthur River Complex, Fiordland, New Zealand: Cretaceous magmatism and metamorphism on the palaeo-Pacific margin. Journal of Metamorphic Geology. 21:299–313. doi: 10.1046/j.1525-1314.2003.00443.x
- Ireland TR, Gibson GM. 1998. SHRIMP monazite and zircon geochronology of high-grade metamorphism in New Zealand. Journal of Metamorphic Geology. 16:149–167. doi: 10.1111/j.1525-1314.1998.00112.x
- Kimbrough DL, Tulloch AJ, Coombs DS, Landis CA, Johnston MR, Mattinson JM. 1994. Uranium-lead zircon ages from the Median Tectonic Zone, New Zealand. New Zealand Journal of Geology and Geophysics. 37:393–419. doi: 10.1080/00288306.1994.9514630
- Klepeis KA, Clarke GL, Gehrels G, Vervoort J. 2004. Processes controlling vertical coupling and decoupling between the upper and lower crust of orogens: results from Fiordland, New Zealand. Journal of Structural Geology. 26:765–791. doi: 10.1016/j.jsg.2003.08.012
- Klepeis KA, Daczko NR, Clarke GL 1999. Kinematic vorticity and tectonic significance of superposed mylonites in a major lower crustal shear zone, northern Fiordland, New Zealand. Journal of Structural Geology. 21(10):1385–1405. doi: 10.1016/S0191-8141(99)00091-7
- Klepeis KA, King D, De Paoli M, Clarke GL, Gehrels G. 2007. Interaction of strong lower and weak middle crust during lithospheric extension in western New Zealand. Tectonics. 26(4):27 p. doi: 10.1029/2006TC002003
- Kylander-Clark ARC, Hacker BR, Cottle JM. 2013. Laser-ablation split-stream ICP petrochronology. Chemical Geology. 345:99–112. doi: 10.1016/j.chemgeo.2013.02.019
- Landis CA, Coombs DS. 1967. Metamorphic belts and orogenesis in southern New Zealand. Tectonophysics. 4(4–6):501–518. doi: 10.1016/0040-1951(67)90014-5
- Liu J, Scott JM, Martin CE, Pearson DG. 2015. The longevity of Archean mantle residues in the convecting upper mantle and their role in young continent formation. Earth and Planetary Science Letters. 424:109–118. doi: 10.1016/j.epsl.2015.05.027
- Ludwig KR. 2012. User's Manual for Isoplot 3.70, Berkeley Geochronology Center. Special Publication no. 4.
- McCoy-West AJ, Bennett VC, O’Neill HSC, Hermann J, Puchtel IS. 2015. The interplay between melting, refertilization and carbonatite metasomatism in off-cratonic lithospheric mantle under Zealandia: an integrated major, trace and platinum group element study. Journal of Petrology. 56:563–604. doi: 10.1093/petrology/egv011
- McCoy-West AJ, Bennett VC, Puchtel IS, Walker RJ. 2013. Extreme persistence of cratonic lithosphere in the southwest Pacific: Paleoproterozoic Os isotopic signatures in Zealandia. Geology. 41:231–234. doi: 10.1130/G33626.1
- Muir RJ, Ireland TR, Weaver SD, Bradshaw JD, Evans JA, Eby GN, Shelley D. 1998. Geochronology and geochemistry of a Mesozoic magmatic arc system, Fiordland, New Zealand. Journal of the Geological Society, London. 155:1037–1053. doi: 10.1144/gsjgs.155.6.1037
- Münker C, Cooper R. 1999. The Cambrian arc complex of the Takaka Terrane, New Zealand: an integrated stratigraphical, paleontological and geochemical approach. New Zealand Journal of Geology and Geophysics. 42:415–445. doi: 10.1080/00288306.1999.9514854
- Negrini M, Smith SA, Scott JM, Tarling MS. 2018. Microstructural and rheological evolution of calcite mylonites during shear zone thinning: constraints from the Mount Irene shear zone, Fiordland, New Zealand. Journal of Structural Geology. 106:86–102. doi: 10.1016/j.jsg.2017.11.013
- Oliver GJH. 1980. Geology of the granulite and amphibolite facies gneisses of Doubtful Sound, Fiordland, New Zealand. New Zealand Journal of Geology and Geophysics. 23:27–41. doi: 10.1080/00288306.1980.10424190
- Oliver GJH, Coggon JH. 1979. Crustal structure of Fiordland, New Zealand. Tectonophysics. 54:253–292. doi: 10.1016/0040-1951(79)90371-8
- Paton C, Hellstrom J, Paul B, Woodhead J, Hergt J. 2011. Iolite: freeware for the visualisation and processing of mass spectrometric data. Journal of Analytical Atomic Spectrometry. 26:2508. doi:10.1039/c1ja10172b.
- Paton C, Woodhead J, Hellstrom J, Hergt J, Greig A, Maas R. 2010. Improved laser ablation U-Pb zircon geochronology through robust downhole fractionation correction. Geochemistry, Geophysics, Geosystems. 11:36 p. doi:10.1029/2009GC002618.
- Pearson DG, Canil D, Shirey SB. 2014. Mantle samples included in volcanic rocks: xenoliths and diamonds. Treatise in Geochemistry 2nd Edition. 169–253. doi:10.1016/B978-0-08-095975-7.00216-3.
- Schwartz JJ, Klepeis KA, Sadorski JF, Stowell HH, Tulloch AJ, Coble MA. 2017. The tempo of continental arc construction in the Mesozoic Median Batholith, Fiordland, New Zealand. Lithosphere. 9:343–365. doi: 10.1130/L610.1
- Schwartz JJ, Stowell HH, Klepeis KA, Tulloch AJ, Kylander-Clark AR, Hacker BR, Coble MA. 2016. Thermochronology of extensional orogenic collapse in the deep crust of Zealandia. Geosphere. 12:647–677. doi: 10.1130/GES01232.1
- Scott JM, Brenna M, Crase JA, Waight TE, van der Meer QHA, Cooper AF, Palin JM, Le Roux P, Münker C. 2016. Peridotitic lithosphere metasomatized by volatile-bearing melts, and its association with intraplate alkaline HIMU-like magmatism. Journal of Petrology. 57:2053–2078. doi: 10.1093/petrology/egw069
- Scott JM, Cooper AF. 2006. Early Cretaceous extensional exhumation of the lower crust of a magmatic arc: evidence from the Mount Irene Shear Zone, Fiordland, New Zealand. Tectonics. 25:15. doi: 10.1029/2005TC001890
- Scott JM, Cooper AF, Palin JM, Tulloch AJ, Kula J, Jongens R, Spell TL, Pearson NJ. 2009. Tracking the influence of a continental margin on growth of a magmatic arc, Fiordland, New Zealand, using thermobarometry, thermochronology, and zircon U-Pb and Hf isotopes. Tectonics. 28:20. doi: 10.1029/2009TC002489
- Scott JM, Hodgkinson A, Palin JM, Waight TE, van der Meer QHA, Cooper AF. 2014. Ancient melt depletion overprinted by young carbonatitic metasomatism in the New Zealand lithospheric mantle. Contributions to Mineralogy and Petrology. 167:17. doi: 10.1007/s00410-014-0963-0
- Scott JM, Palin JM. 2008. LA-ICP-MS U-Pb zircon ages from Mesozoic plutonic rocks in eastern Fiordland, New Zealand. New Zealand Journal of Geology and Geophysics. 51:105–113. doi: 10.1080/00288300809509853
- Scott JM, Palin JM, Cooper AF, Fagereng Å, King RP. 2009. Polymetamorphism, zircon growth and retention of early assemblages through the dynamic evolution of a continental arc in Fiordland, New Zealand. Journal of Metamorphic Geology. 27:281–294. doi: 10.1111/j.1525-1314.2009.00817.x
- Scott JM, Turnbull IM, Ewing TA, Allibone AH, Palin JM, Cooper AF. 2008. Petrology and geochronology of the volcaniclastic and volcanogenic Mesozoic Loch burn formation in eastern Fiordland, New Zealand. New Zealand Journal of Geology and Geophysics. 51:89–103. doi: 10.1080/00288300809509852
- Scott JM, Waight TE, van der Meer QHA, Palin JM, Cooper AF, Münker C. 2014. Metasomatized ancient lithospheric mantle beneath the young Zealandia microcontinent and its role in HIMU-like intraplate magmatism. Geochemistry, Geophysics, Geosystems. 15:3477–3501. doi: 10.1002/2014GC005300
- Scott JM. 2013. A review of the location and significance of the boundary between the Western Province and Eastern Province, New Zealand. New Zealand Journal of Geology and Geophysics. 56:276–293. doi: 10.1080/00288306.2013.812971
- Spandler C, Hammerli J, Sha P, Hilbert-Wolf H, Hu Y, Roberts E, Schmitz M. 2016. MKED1: a new titanite standard for in Situ analysis of Sm–Nd isotopes and U–Pb geochronology. Chemical Geology. 425:110–126. doi: 10.1016/j.chemgeo.2016.01.002
- Spandler CJ, Arculus RJ, Eggins SM, Mavrogenes JA, Price RC, Reay AJ. 2003. Petrogenesis of the Greenhills Complex, Southland, New Zealand: magmatic differentiation and cumulate formation at the roots of a Permian island-arc volcano. Contributions to Mineralogy and Petrology. 144:703–721. doi: 10.1007/s00410-002-0424-z
- Stacey J, Kramers JD. 1975. Approximation of terrestrial lead isotope evolution by a two-stage model. Earth and Planetary Science Letters. 26:207–221. doi: 10.1016/0012-821X(75)90088-6
- Stewart E, Lamb W, Newman J, Tikoff B. 2016. The petrological and geochemical evolution of early forearc mantle lithosphere: an example from the Red Hills Ultramafic Massif, New Zealand. Journal of Petrology. 57:751–776. doi: 10.1093/petrology/egw017
- Stowell H, Parker KO, Gatewood M, Tulloch A, Koenig A. 2014. Temporal links between pluton emplacement, garnet granulite metamorphism, partial melting and extensional collapse in the lower crust of a Cretaceous magmatic arc, Fiordland, New Zealand. Journal of Metamorphic Geology. 32:151–175. doi: 10.1111/jmg.12064
- Stowell H, Tulloch A, Zuluaga C, Koenig A. 2010. Timing and duration of garnet granulite metamorphism in magmatic arc crust, Fiordland, New Zealand. Chemical Geology. 273:91–110. doi: 10.1016/j.chemgeo.2010.02.015
- Szilas K, Kelemen PB, Bernstein S. 2015. Peridotite enclaves hosted by Mesoarchaean TTG-suite orthogneisses in the Fiskefjord region of southern West Greenland. Geo Res J. 7:22–34.
- Tulloch AJ, Ireland TR, Kimbrough DL, Griffin WL, Ramezani J. 2011. Autochthonous inheritance of zircon through Cretaceous partial melting of Carboniferous plutons: the Arthur River Complex, Fiordland, New Zealand. Contributions to Mineralogy and Petrology. 161:401–421. doi: 10.1007/s00410-010-0539-6
- Turnbull IM, Allibone AH, Jongens R. 2010. Geology of the Fiordland area: scale 1:250,000. Lower Hutt: GNS Science. Institute of Geological & Nuclear Sciences 1:250,000 geological map 17. 97 p. + 1 folded map.
- Turnbull RE, Size WB, Tulloch AJ, Christie AB. 2017. The ultramafic–intermediate Riwaka Complex, New Zealand: summary of the petrology, geochemistry and related Ni–Cu–PGE mineralisation. New Zealand Journal of Geology and Geophysics. 60:270–295. doi: 10.1080/00288306.2017.1316747
- van der Meer QHA, Waight TE, Scott JM, Münker C. 2017. Variable sources for Cretaceous to recent HIMU and HIMU-like intraplate magmatism in New Zealand. Earth and Planetary Science Letters. 469:27–41. doi: 10.1016/j.epsl.2017.03.037