ABSTRACT
In previous research, we produced a japonica rice (Oryza sativa L.) cultivar (‘Koshihikari Kan No. 1’) with nearly undetectable levels of cadmium (Cd) in the grains. In this study, we hypothesized that growing this cultivar aerobically would simultaneously reduce arsenic (As) and Cd concentrations in the grains. We grew this cultivar and ‘Koshihikari’, its parent, in paddy fields with different soil properties under three water regimes: flooded conditions (FLD), alternate wetting and drying conditions (AWD) and water-saving conditions (WAS). FLD for several weeks before and after heading significantly increased the grain As concentration in both cultivars. AWD, with the soil re-flooded just after disappearance of the ponded water, reduced grain As concentrations by an average of 27% relative to FLD for both cultivars. WAS, with irrigation after drying of the soil surface, decreased grain As concentrations by an average of 43.1% for ‘Koshihikari Kan No. 1’ and 48.2% for ‘Koshihikari’ compared to FLD. Although AWD and WAS remarkably increased grain Cd concentrations in ‘Koshihikari’, ‘Koshihikari Kan No. 1’ had nearly undetectable levels of grain Cd in all treatments. Compared with ‘Koshihikari’ in FLD, grain yield of ‘Koshihikari Kan No. 1’ and ‘Koshihikari’ decreased by averages of 2% for AWD and 4 to 6% for WAS. In addition, WAS tended to decrease grain quality slightly for both cultivars. Although aerobic conditions such as WAS have somewhat adverse effects on grain yield and quality, growing the low-Cd cultivar aerobically is the most practical way to simultaneously reduce Cd and As contents in the rice grains.
1. Introduction
Food contamination with toxic metals such as arsenic (As) and cadmium (Cd) is a serious threat to human health. Rice (Oryza sativa L.) is a major dietary source of As and Cd for populations that consume rice as a staple food (Williams et al. Citation2007; Meharg et al. Citation2013). The Codex Alimentarius Commission of the Food and Agriculture Organization of the United Nations (FAO)/World Health Organization (WHO) therefore determined maximum allowable levels of Cd and As in polished rice in 2008 and 2014, respectively: 0.4 mg kg−1 for Cd and 0.2 mg kg−1 for inorganic As (Codex Alimentarius Commission Citation2014). On the basis of the Codex level of rice Cd, the Japanese domestic standard for Cd was changed from 1.0 to 0.4 mg kg−1 for unpolished rice in 2011. At present, approximately 40,000 ha of Japanese paddy fields are subjected to countermeasures (i.e., flooding) to reduce Cd concentrations in rice. Although flooding creates anaerobic conditions that can reduce rice Cd concentrations, these conditions can increase As concentrations in rice because of the high solubility of arsenite (As[III]) in the paddy soil (Arao et al. Citation2009). According to a survey of rice As concentrations by the Ministry of Agriculture, Forestry and Fisheries of Japan (MAFF), 2.2% of polished rice cultivated in Japan in 2012 exceeded 0.2 mg kg−1 inorganic As, and the average value (n = 600) of inorganic As was 0.12 mg kg−1 for polished rice and 0.21 mg kg−1 for brown rice; the latter value represents an increase compared to the level of 0.15 mg kg−1 for brown rice from 2004 to 2006 (MAFF Citation2014). One possibility is that the As concentration in rice has recently increased in response to the flooding used to reduce Cd concentrations in rice. Thus, there appears to be a trade-off between As and Cd uptake by rice, and we urgently need to develop new techniques for simultaneously reducing As and Cd concentrations in rice.
Water management before and after heading greatly affects the concentrations of As and Cd in rice (Arao et al. Citation2009). In pot experiments with seven water regimes, Arao et al. (Citation2009) found that flooding for 3 weeks before and 3 weeks after heading was most effective in reducing rice Cd concentrations, whereas aerobic treatment during the same period reduced As concentrations in rice. Because the period from heading to flowering is the most critical stage in terms of rice’s water requirements (Garrity and O’Toole Citation1995), it is not easy to withhold irrigation from paddy fields to decrease As uptake by rice. Alternate wetting and drying, which involves re-flooding the soil after the ponding water disappears, is an effective treatment for reducing As concentrations in rice, conserving water and reducing greenhouse gas emissions. For example, Linquist et al. (Citation2015) reported that alternate wetting and drying in which the soil contained 40 or 60% of the saturated volumetric water content when the field was re-flooded reduced grain As concentrations by 56%, on average, while also decreasing methane (CH4) emission by 93% and improving water-use efficiency by 43–63% compared to the flooded treatment. However, such alternate wetting and drying treatments decreased grain yields by 5–13%. Although Linquist et al. (Citation2015) did not measure grain Cd concentrations, it is likely that they were higher than in the flooded treatment.
Sprinkler irrigation, another system that can conserve irrigation water, is also effective in reducing As concentrations in rice. Spanu et al. (Citation2012) demonstrated that 37 rice genotypes grown under sprinkler irrigation had about 2% of the total grain As of the same genotypes grown under continuous flooding. A similar reduction under sprinkler irrigation was reported by Moreno-Jiménez et al. (Citation2014), but they found significantly increased Cd in the rice grains. Thus, it may be impossible to simultaneously reduce As and Cd concentrations in the rice grains by manipulating the water regime alone, as Hu et al. (Citation2013) noted.
This suggests that it may be necessary to consider alternative strategies, such as identifying genotypes that take up less Cd and As. Kuramata et al. (Citation2013) showed that the genetic diversity of the grain As concentration is narrower than that of grain Cd concentrations in rice. In addition, several reports suggested that the rankings of rice cultivars for total grain As concentrations vary greatly across environments (Norton et al. Citation2009; Ahmed et al. Citation2011), indicating the difficulty of genotype selection for lowering As concentration in the rice grains. On the other hand, by using ion-beam mutagenesis, we produced non-transgenic rice mutants with mutations of the OsNramp5 gene, which encodes a manganese (Mn) transporter that incidentally transports Cd into rice, and the mutants had nearly undetectable levels of Cd in the grains, even when the rice was cultivated under aerobic conditions in paddy fields contaminated with high levels of Cd (Ishikawa et al. Citation2012). Therefore, we hypothesized that if the low-Cd mutants were cultivated under aerobic soil conditions, where As(III) solubility is low, it would be possible to simultaneously reduce As and Cd concentrations in the rice grains. To test this hypothesis, we examined the ability of different water regimes to simultaneously reduce Cd and As concentrations in the grains of a low-Cd mutant rice grown in paddy fields.
2. Materials and methods
Field experiments were conducted in farm fields or fields at local agricultural research stations at nine sites in Japan. Paddy rice was cultivated at five of the sites (A, B, C, D and E) in 2013 and at the remaining four sites (F, G, H and I) in 2014. The geographical locations have not been described, to prevent possible losses caused by people acting on harmful rumors about As concentrations in the rice grains.
2.1. Soil sample analysis
Bulk soil samples were collected from each site to a depth of 15 cm in the surface soil before planting. The samples were air-dried, passed through a 2-mm stainless steel sieve and then used for various chemical analyses. The soil type was based on a classification for cultivated soils in Japan (Classification Committee of Cultivated Soils Citation1996). Soil texture was evaluated by means of the pipette technique (Gee and Bauder Citation1986). Soil pH was determined by means of the glass-electrode method, with a ratio of soil to water of 1:2.5 weight/volume (w/v; Makino et al. Citation2006). The total carbon and total nitrogen (N) contents of the soils were measured by means of a dry combustion method (Makino et al. Citation2006). Cation exchange capacity (CEC) was measured by the continuous leaching technique, with a 1 mol L−1 ammonium acetate buffer solution (pH 7) (Sumner and Miller Citation1996). The phosphorus (P) adsorption coefficient was determined using 2.5% ammonium phosphate (Takata et al. Citation2011). Two selective dissolution experiments for iron (Fe) in soils were carried out: acid ammonium oxalate for the non-crystalline form and dithionite-citrate for free iron oxide (Blakemore et al. Citation1981). Available silicate (Nonaka and Takahashi Citation1988) and exchangeable Mn (Gambrell Citation1996) were extracted with water and 1 mol L−1 ammonium acetate solution, respectively, with minor modification of the original methods. Soil Cd and As were extracted using 0.1 M and 1 M hydrochloric acid (HCl), respectively (Ishikawa et al. Citation2006; Kuramata et al. Citation2011).
2.2. Plant materials
Two japonica rice cultivars, ‘Koshihikari’ and ‘Koshihikari Kan No. 1’, were cultivated at all sites. ‘Koshihikari’ is the most popular rice cultivar grown in Japan because of its good taste and eating quality, and it is grown in nearly 40% of the rice fields in Japan (Takeuchi et al. Citation2008). ‘Koshihikari Kan No. 1’ (line lcd-kmt2) is a mutant that we developed by irradiating seeds of ‘Koshihikari’ with accelerated carbon ions. This cultivar accumulates nearly undetectable levels of Cd in the grains because of a loss-of-function mutation of the OsNramp5 gene (Ishikawa et al. Citation2012). ‘Koshihikari Kan No. 1’ was recently accepted as a new variety by MAFF.
2.3. Field layout
Three irrigation regimes were established at all sites, as illustrated in . Approximately 1 month after sowing, seedlings of the two cultivars were transplanted into flooded paddy soils, and then mid-season drainage was applied for approximately 1 week, 30 to 40 d before heading. Because mid-season drainage has many benefits for rice cultivation, including an increased supply of oxygen (O2) to rice roots to prevent hydrogen sulfide toxicity, control of excessive tillering, and increased bearing strength of the soil during mechanical harvesting (Minamikawa et al. Citation2014), it is widely practiced in Japan. After mid-season drainage, rice plants were cultivated under one of three water regimes: flooded conditions (FLD), with flooding for 2 or 3 weeks before and after heading; alternate wetting and drying conditions (AWD), with re-flooding of the soil just after disappearance of the ponding water; and water-saving conditions (WAS), with irrigation water re-applied after drying of the soil surface. FLD is normally implemented in areas where rice could potentially exceed the maximum allowable Cd level (0.4 mg kg−1) in rice grains. AWD is the conventional regime for cultivating paddy rice in Japan. For WAS, the duration of the dry-soil period in one cycle ranged from 2 to 7 d, depending on the water-holding capacity of the soil at each site. To prevent water stress at heading under WAS, AWD was applied for 10 d after the emergence of several panicles, and then the WAS was repeated. For all fields, irrigation water was drained 7 d before harvesting. All water regimes were designed so that they could be easily applied by farmers.
Figure 1. Illustration of the three irrigation regimes that were applied to paddy fields at the nine sites after the mid-season drainage.
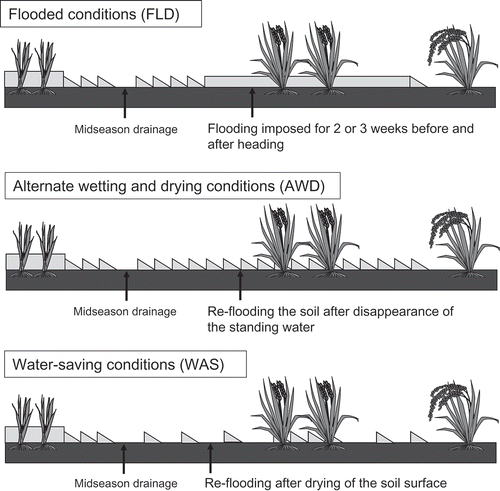
A split-plot design was used, with water regime as the main plot and rice cultivar as the sub-plot. Each main plot covered from 30 to 400 m2, depending on the size of the experimental site. There were two or three replicates in each treatment. Commercial fertilizers containing N, P and potassium (K) were applied at the conventional levels and timings at each site.
2.4. Determination of soil redox potentials and collection of soil solution for As and Cd analyses
To determine soil redox potentials (Eh), three to five platinum electrodes were installed in the main plot at each site at a depth of approximately 5 cm below the soil surface. Soil Eh was measured using a digital multi-meter with a silver chloride reference electrode that was temporarily placed at the soil surface at the time of measurement. Voltages were recorded five times: just before mid-season drainage, after mid-season drainage, 2 weeks before heading, at heading and 2 to 3 weeks after heading.
To collect the soil solution, soil-water samplers (Rhizon MOM, Rhizosphere Research Products, Wageningen, the Netherlands) were buried at three locations in the main plot of each site at a depth of approximately 5 cm below the soil surface. Water in the soil was drawn into the sampler through its porous ceramic cup by negative pressure and collected in a sample tube. The sampling was conducted simultaneously with the soil Eh measurements. The collected soil solution was diluted immediately with 0.66 mL of 2.2 M nitric acid (HNO3; atomic-absorption grade; Kanto Chemical Inc., Tokyo, Japan) to prevent co-precipitation of As induced by the production of ferric irons in the soil solution, and was then filtered through a 0.2-µm polyethersulfone (PES) membrane in a 13-mm syringe filter (Agilent Captiva Econofilter, Agilent Technologies, Santa Clara, CA, USA). The total As and total Cd concentrations in the soil solution were analyzed by inductively coupled plasma mass spectrometry (ICP-MS, NexIONTM 300X, PerkinElmer Japan, Yokohama, Japan), as described in section 2.6, within several days after collection.
2.5. Grain yield and quality and collection of plant samples
Whole-grain samples were collected from 20 randomly selected plants per plot, bulked to provide a single sample for analysis, and dried to 15% w/w moisture content. The straw samples were air-dried and weighed. Husked grains (brown rice) were screened through a 1.8- or 1.9-mm mesh to remove immature rice and weighed to evaluate the grain yield. In this study, “grains” represents unpolished brown rice, unless otherwise noted. Grain quality and grain size were analyzed using a rice inspector (RGQI 10B, Sakate Co., Higashihiroshima, Japan). The rice inspector is a high-throughput machine for visually examining rice quality on an individual-grain basis. In Japan, the whole-grain ratio is used as one criterion for determining rice grade; it represents the percentage of intact grains remaining after removing cracked, broken, dead, immature and other unsuitable grains, using a sample of 1000 grains. A whole-grain ratio greater than 70% is considered to be first-grade rice. The whole-grain ratio was evaluated based on the data from the rice inspector.
2.6. Metal analyses
Grain and straw samples were ground into a fine powder using a ball mill (ShakeMaster Auto ver. 1.5, Bio Medical Science, Tokyo, Japan). A 1-g powdered sample was digested at 105°C for 3 h in a 65-mL polypropylene tube (DigiTUBE, GL Sciences, Tokyo, Japan) with 5 mL of a 13 M HNO3 solution (Kanto Chemical Inc.) using a heating block system (Digi-PREP LS, SPS Science, Baie d’Urfé, Quebec, Canada). After cooling to ambient temperature, 1 mL of hydrogen peroxide (H2O2; atomic-absorption grade, Kanto Chemical Inc.) was added to the solution, which was then heated at 105°C for 2 h. The digested solution was filtered through a 0.2-µm PES membrane filter and adjusted to 50 mL by adding Milli-Q water. Two certified standard materials, NIST CRM 1568a rice flour (National Institute of Standards and Technology, Gaithersburg, MD, USA) and NMIJ CRM 7503a rice flour (National Institute of Advanced Industrial Science and Technology, Tsukuba, Japan), were used for quality assurance. Concentrations of total As and Cd in the grains and shoots were determined by means of ICP-MS, as described below. The Mn concentrations were also analyzed to evaluate differences between cultivars. Indium was added to the samples as the internal standard. In this study, 75As16O was detected at m/z 91 by reaction with oxygen in a dynamic reaction cell to avoid spectral interference of 40Ar35Cl at m/z 75 for 75As. The ICP-MS signal was monitored at m/z 111 for Cd and 55 for Mn.
To identify the As species in the rice grains, a powdered sample of ground rice (0.2 g dry weight) was mixed with 1 mL of 0.15 M HNO3 in a 15-mL polyethylene tube, and the mixture was then heated on an aluminum heating block at 80°C for 2 h. The solution obtained was diluted to 5 mL with Milli-Q water and passed through a 0.2-µm PES membrane filter. The concentrations of the As species in the filtered samples were determined by means of ICP-MS combined with high-performance liquid chromatography (HPLC) in accordance with the method of Baba et al. (Citation2014). Briefly, four As species [As(III); arsenate, As(V); monomethylarsonic acid MMA(V); and dimethylarsinic acid DMA(V)] were separated using HPLC (Flexar, PerkinElmer) on a Discovery HS F5 column (5 µm i.d., 4.6 mm × 250 mm; Sigma-Aldrich, St, Louis, MO, USA) with a mobile phase of 0.1% [volume/volume (v/v)] methanoic acid containing 1% (v/v) methanol. The solution eluted from the column was directly introduced into the ICP-MS system, and the arsenic signal (m/z = 91 for 75As16O) was monitored. The concentrations of the As species were determined by means of external calibration based on the position of and area under each peak. The sum of As(III) and As(V) represented the inorganic As.
2.7. Statistical analysis
Statistical analysis was performed using version 20.0 of the IBM SPSS Statistics package (IBM Japan, Tokyo). Differences in the expected genotypic variance in grain As (G), in water management (W), and in the G × W interaction were estimated for each site by means of analysis of variance (ANOVA), and the percentage of the variation explained by each source of variation was calculated for the sum of squares of each effect to provide the total sum of squares. Tukey’s honestly significant difference (HSD) test was conducted to identify significant differences in grain As and Cd concentrations among the water regimes. Grain yield and the whole-grain ratio at each experimental site were compared with those of ‘Koshihikari’ in the FLD treatment using a one-tailed Dunnett’s test.
3. Results
3.1. Soil properties at each site
summarizes the soil properties at each experimental site. The sites had three main soil types: Gley lowland soils (sites A, B, D and G), Gray lowland soils (sites C, H and I) and Andosols (sites E and F). Soil textures at the sites were sandy loam (sites B and G), loam (sites C, F and I), clay loam (site H), silty clay loam (site D), sandy clay (site A) and light clay (site E). Soil pH ranged from 5.0 to 6.6, and was therefore slightly acidic at all sites. The two Andosols had the highest total carbon, total N, CEC and P adsorption coefficient values. Free Fe oxide and non-crystalline Fe contents varied widely, ranging from 5.3 to 37.4 g kg−1 and from 1.5 to 19.9 g kg−1, respectively; the Andosols had the highest and third highest values for these Fe fractions, with one Humic Gley lowland soil (site D) having the second highest value. The available silicon (Si) concentration ranged from 16.4 to 39.0 mg kg−1. The exchangeable Mn concentration varied widely, ranging from 3.0 to 50.0 mg kg−1, and the Humic Gley lowland soil at site D had the highest value. The HCl-extractable soil As and Cd concentrations also varied widely, from 1.26 to 6.72 mg kg−1 and from 0.12 to 3.62 mg kg−1, respectively, with sites D and I having the highest levels of both As and Cd.
Table 1. Soil properties in the nine experimental fields.
3.2. Changes in soil Eh
Changes in soil Eh among the five stages of rice cultivation were monitored at each experimental site (). Although the Eh values fluctuated widely, there was an overall trend in how soil Eh differed among the water regimes. Soil Eh values in all regimes were negative or close to zero before mid-season drainage at all sites except site D in the AWD regime and site E in all three regimes; their values then increased remarkably after mid-season drainage, reaching positive values at many sites. The different irrigation regimes began after mid-season drainage (). The input of irrigation water decreased soil Eh values again, and the FLD regime maintained negative values or values near zero at all sites except F and I. Changes in soil Eh in the AWD regime followed similar patterns to those of FLD, but the values were often higher. Compared with the FLD and AWD regimes, the WAS regime showed a shift to aerobic conditions after mid-season drainage at all sites except D and E.
3.3. As and Cd concentrations in the soil solution
shows the changes in total As concentrations in the soil solution over time. The changes at sites E, F and I (the two Andosols and a Gray lowland soil, respectively) were too small to be apparent, so we will focus our discussion on the remaining sites. All paddy fields were kept under flooded (anaerobic, reducing) conditions before mid-season drainage, and these conditions induced the release of As into the soil solution. However, As concentrations decreased greatly after mid-season drainage at all sites and in all regimes. After the input of irrigation water, the As concentrations increased again at most sites, although the increase was not apparent for some site–treatment combinations. Arsenic concentrations were generally highest in FLD, followed by AWD (except at site D). WAS had remarkably lower As concentrations than the other two irrigation regimes after mid-season drainage at all sites except D, and As levels in WAS were below the detection limit (< 1 ng mL−1) at most sites except D and G. At sites E and F (both Andosols), the As concentrations were below the detection limit, even in the FLD regime. Inorganic As [As(III) and As(V)] dominated the As species detected in the soil solution (data not shown). The Cd concentrations were one or two orders of magnitude lower than the As concentrations in the soil solution (, Fig. S1). In the WAS regime, concentrations of Cd increased greatly in the soil solution after mid-season drainage at sites A and B and at heading at sites H and I.
Figure 3. Effects of the three water regimes (FLD, flooding; AWD, alternate wetting and drying; WAS, water-saving) on arsenic (As) concentrations in the soil solution at the nine experimental sites. The soil solution was collected at the same time as the soil redox potential (Eh; ) was determined. Values are means ± standard deviation (SD; n = 3).
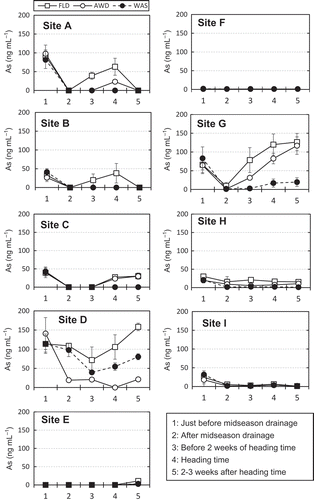
3.4. Concentrations of As and Cd in rice grains
Concentrations of As and Cd in the rice grains varied widely among the sites and the water regimes (). The grain As concentrations of ‘Koshihikari’ and ‘Koshihikari Kan No. 1’ ranged from 0.046 to 0.656 mg kg−1 and from 0.045 to 0.653 mg kg−1, respectively, across sites and water treatments. The highest grain As concentration was found in FLD at site B in both cultivars. Arsenic concentrations were highest in FLD, followed by AWD and WAS, at all sites except D and F. Sites E and F showed remarkably lower grain As concentrations in both cultivars than at the other sites; As concentrations were less than 0.1 mg kg−1 even in the FLD regime. The mean grain As concentrations of ‘Koshihikari’ and ‘Koshihikari Kan No. 1’, except at sites D and F, were 27.3 and 27.4% less in the AWD regime and 48.2 and 43.1% less in the WAS regime, respectively, than in the FLD regime. The grain As concentrations for both cultivars grown at site B were especially drastically decreased by the AWD and WAS regimes: the concentrations were 53.3 and 69.8% lower in the AWD and WAS regimes, respectively, than in the FLD regime. Inorganic As was the dominant species in the rice grains, irrespective of sites, cultivars and water regimes (Fig. S2).
Figure 4. Effects of the three water regimes (FLD, flooding; AWD, alternate wetting and drying; WAS, water-saving) on the concentrations of arsenic (As) and cadmium (Cd) in grains (brown rice) of (A) ‘Koshihikari’ and (B) ‘Koshihikari Kan No. 1’ grown at the nine experimental sites. Values are means ± standard deviation (SD; n = 3 to 4). Bars at a site labeled with different letters differ significantly (Tukey’s honestly significant difference test, p < 0.05).
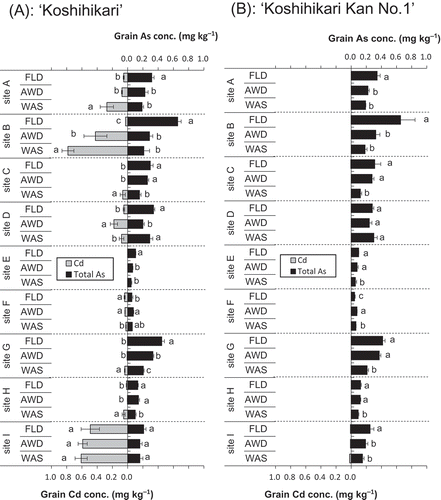
In ‘Koshihikari’, grain Cd concentrations were highest in the WAS regime at most sites, followed by AWD and FLD, indicating a trade-off between As and Cd in rice that depended on the water regimes. On the other hand, the Cd concentrations in ‘Koshihikari Kan No. 1’ were not detected at most sites and in most water regimes. There was a strong and significant positive correlation between the grain and straw As concentrations (Fig. S3). Two-way ANOVA indicated that the total grain As concentrations differed significantly among the water regimes (W); it explained 46.3 to 93.8% of the total variance (). Genotype (G) and the W × G interaction did not contribute significantly to the variance, except for a significant 6% contribution of genotype at site H, which indicates that the differences in grain As concentrations observed in this study depended mostly on the water regime.
Table 2. Two-way analysis of variance (ANOVA) for the effects of water regime, genotype and their interaction on grain arsenic (As) concentrations in rice (Oryza sativa L.) grown at each of the nine sites.
3.5. Mn concentrations in grains and straw
In most cases, Mn concentrations were much higher in the straw than in the grains (). The grain Mn concentration in ‘Koshihikari’ and ‘Koshihikari Kan No. 1’ averaged 25.9 and 17.3 mg kg−1, respectively, with ranges of 19.6 to 35.0 and 9.0 to 27.1 mg kg−1, respectively (). ‘Koshihikari Kan No. 1’ had a grain Mn concentration that was 30% lower on average than that in ‘Koshihikari’. The straw Mn concentrations decreased drastically in ‘Koshihikari Kan No. 1’ compared with those in ‘Koshihikari’ (from 621 to 139 mg kg−1, a decrease of 77.6%; ); values varied widely, ranging from 304 to 1172 mg kg−1 and from 25 to 378 mg kg−1, respectively. WAS had an especially strong tendency to decrease the Mn concentrations in ‘Koshihikari Kan No. 1’ compared with ‘Koshihikari’: Mn concentrations of the grains and straw in ‘Koshihikari Kan No. 1’ decreased by an average of 19 and 36%, respectively, compared with the values in FLD, whereas WAS did not decrease the Mn concentrations of ‘Koshihikari’.
3.6. Grain yields
Grain yields of ‘Koshihikari’ in FLD served as the control group. The grain yields varied across the experimental sites (). The highest and lowest yields of ‘Koshihikari’ grown in the FLD regime were found at sites B and I, respectively. In the FLD regime, the average yield of ‘Koshihikari Kan No. 1’ was similar to (i.e., 98% of) that of ‘Koshihikari’. Although the AWD regime significantly decreased the yields of ‘Koshihikari Kan No. 1’ at site B and ‘Koshihikari’ at site D, the average yield for the nine sites did not differ between the cultivars and was similar to that in the control. WAS significantly decreased the yield of ‘Koshihikari Kan No. 1’ at sites B and E. Although the average yield of ‘Koshihikari Kan No. 1’ in the WAS treatment was 6% lower than that of the control, this decrease was not significantly different from the decrease for ‘Koshihikari’.
Table 3. Grain yields (t ha−1) of the two rice (Oryza sativa L.) cultivars grown under different water regimes (FLD, flooding; AWD, alternate wetting and drying; WAS, water-saving) at each of the nine sites.
3.7. Grain quality
presents the whole-grain ratio data. Due to a long heat wave in 2013, rice grain quality was worse than usual at most of our study sites. The whole-grain ratio of both cultivars in 2013 was less than 40%, even in the FLD regime, at all sites except D, which is located in northeastern Japan (a cooler region). WAS significantly decreased the whole-grain ratio of ‘Koshihikari Kan No. 1’ at sites B, C and E. Rice grain quality in 2014 was similar to the usual value, with the whole-grain ratio greater than 60% at all sites. There were no significant differences in whole-grain ratio between the rice cultivars grown under the different water regimes in 2014. The average whole-grain ratio was 3.0 and 2.6% lower in ‘Koshihikari Kan No. 1’ than in ‘Koshihikari’ in the FLD and AWD regimes, respectively. The WAS regime decreased the average whole-grain ratio in both cultivars compared with the FLD and AWD regimes, with whole-grain ratio values 7.2 percentage points lower in ‘Koshihikari Kan No. 1’ than in the ‘Koshihikari’ control.
Table 4. Whole-grain ratio of rice (Oryza sativa L.) cultivars grown under different water conditions (FLD, flooding; AWD, alternate wetting and drying; WAS, water-saving conditions) at each of the nine sites.
4. Discussion
Our results clearly demonstrate that the low-Cd rice cultivar, ‘Koshihikari Kan No. 1’, offers the possibility of simultaneously decreasing As and Cd concentrations in the rice grains when this cultivar is grown under the AWD regime, with even better results under the WAS regime. Although the WAS regime significantly increased grain Cd concentrations in ‘Koshihikari’, to levels that exceeded the maximum allowable level of 0.4 mg kg−1 at sites B and I, grain Cd concentrations in ‘Koshihikari Kan No. 1’ were at or below the limit of quantification at all experimental sites (), indicating that under all of the water conditions, ‘Koshihikari Kan No. 1’ took up only small quantities of Cd from the soil types we studied. Previous studies have shown that the activity of Cd in soils and its availability for plant uptake are greatly influenced by soil properties such as soil pH, clay content, CEC, organic carbon content and the coexistence of other heavy metals (Grant et al. Citation1999; Bolan et al. Citation2013). In our study, grain Cd concentrations in ‘Koshihikari’ did not necessarily correspond to the HCl-extractable Cd concentrations in soils. The highest grain Cd concentration in ‘Koshihikari’ grown in the WAS treatment was at site B; this may have been because this site had the lowest pH and lowest CEC. However, Cd uptake by ‘Koshihikari Kan No. 1’ does not seem to have been affected by soil properties at any site; that is, it remained low at all sites and under all water regimes. There was no significant difference in grain As concentrations between ‘Koshihikari’ and ‘Koshihikari Kan No. 1’ except at site H (). This suggests that the OsNramp5 mutation in ‘Koshihikari Kan No. 1’ did not affect As accumulation in the grains. As in previous research (Arao et al. Citation2009; Hu et al. Citation2013), we also found high variation in grain As concentrations among the different water regimes ().
The changes in soil Eh over time in the different water regimes () would have directly affected the solubility of As in the soil solution. Arsenic’s behavior in paddy soils is well understood (Takahashi et al. Citation2004). Under aerobic conditions, As is less mobile because arsenate [As(V)] is strongly adsorbed to Fe oxides and hydroxides. Under anaerobic conditions, as in the FLD regime, As(V) is released from Fe oxides and hydroxides by means of reductive dissolution, and is reduced to As(III) through biological processes mediated by microbes (Islam et al. Citation2004), resulting in greater As uptake by rice via a silicon transporter (Ma et al. Citation2008). Mid-season drainage was very effective at decreasing As concentrations in the soil solution (), and it is likely that the appearance of cracking of the soil surface after mid-season drainage (which increased oxygen infiltration into the soil and created more aerobic conditions) delayed the increase of the As concentration in the soil solution that was induced by irrigation. We expected that after mid-season drainage, soil Eh would shift toward stronger reducing conditions in the FLD regime, stronger oxidizing conditions in the WAS regime and intermediate conditions in the AWD regime at all sites. However, it was difficult to control the water regime at some sites. Because of high rainfall at site D, the paddy soil remained wet from mid-season drainage to heading, and even in the WAS regime the soil Eh remained negative (), resulting in no difference in the grain As concentrations between WAS and FLD for both cultivars (). On the other hand, severe water leakage from the topsoil into the subsoil occurred after mid-season drainage at sites E, F and I, and the FLD regime was maintained by a constant flow of irrigation water. Such a water regime prevented a remarkable decrease in soil Eh () and decreased the solubility of As in the soils (). Thus, we must account for the effects of weather conditions and soil physical and chemical properties on water management when the goal is to reduce As concentrations in rice grains.
Our results appear to show geographical variation in the grain As concentrations. The soil texture may be one of the key factors that explains the observed differences. The highest grain As concentration occurred in the FLD regime at site B (a sandy loam) in both cultivars, and the AWD and WAS regimes drastically and significantly decreased their grain As concentrations (). Site G (a sandy loam) also exhibited higher grain As concentrations in both cultivars than in most other soil textures. In general, soils with a finer texture (i.e., with high contents of silts and clays) have a much greater surface area than soils with a coarse texture (i.e., sandy soils; Sahoo and Kim Citation2013). For the particle-size fractions in a soil, the total As concentration was highest in the clay fraction, followed by the silt and sand fractions (Lombi et al. Citation2000). Lombi et al. (Citation2000) also noted that most As in the clay fraction can be extracted by ammonium oxalate, which is the procedure to dissolve amorphous Fe oxide, suggesting that soils with a higher clay content generally have a higher As-scavenging potential than sandy soils. However, we did not examine As concentrations in non-crystalline Fe forms that are present in the clay fraction of the tested soils.
Site B contained lower amounts of non-crystalline Fe in the soil than did the other sites (). Non-crystalline Fe, including ferrihydrite and amorphous Fe oxide, may act as a sink for As because there is a negative correlation between As bound to amorphous Fe oxide in the soil and As in rice grains (Liu et al. Citation2015). The paddy soil at site B may have a lower capacity for re-adsorption of solubilized As, and this would lead to greater As concentrations in rice grains. Arsenic was not detected in the soil solution at sites E and F (both Andosols; ) and the grain As concentrations were also remarkably lower at these sites than at other sites, even in the FLD regime (). These results appear to be due mainly to higher amounts of non-crystalline Fe, which may act as a sink for As. The formation of Fe plaque on the root surface is one of the factors that affects As uptake by rice because this plaque, mainly composed of ferrihydrite, strongly adsorbs As (Seyfferth et al. Citation2011). Although we did not determine the As associated with this plaque, the soil at site B may form less of the plaque than the other soils we tested do, and this may result in the elevated As concentrations in the rice grains. A significant portion of As adsorbed on the Fe plaque in rice roots grown in flooded soils is present in the form of As(III), which is more easily desorbed than As(V) (Yamaguchi et al. Citation2014). In addition, Fe plaque does not cover many of the younger portions of roots that are most responsible for the active transport of solutes. These findings indicate that Fe plaque may not function as a barrier to As absorption by the rice roots grown in flooded soils, and that their effects on root As uptake should be further investigated.
In addition to high amounts of non-crystalline Fe, Andosols generally have high organic matter contents, as was the case in the present study (high total carbon values in ), and organic matter may have a greater affinity for As sorption, leading to decreased solubility of As and decreased bioavailability for soils and plants (Sahoo and Kim Citation2013). Thus, rice grown in Andosols is likely to have lower grain As concentrations than rice grown in other soil types.
Although ‘Koshihikari Kan No. 1’ has the excellent property of having low Cd uptake, Mn concentrations in its grains and straw were significantly lower than those in ‘Koshihikari’ () because OsNramp5 is a major transporter of Cd and Mn, and ‘Koshihikari Kan No. 1’ has a mutation in its OsNramp5 gene (Ishikawa et al. Citation2012). Mn is an essential microelement for plants and is involved in redox processes in the photosynthetic electron-transport system (Jones Citation1998). Paddy rice is well known to accumulate high amount of Mn. This suggests that rice has a good system for tolerating excess Mn under submerged (reducing) conditions, which increase the solubility of Mn. Yamaji et al. (Citation2013) revealed the function of the OsNramp3 transporter protein at the nodes that plays a role in adaptation to wide changes in Mn levels in the environment. The leaf sufficiency content of Mn ranges from 10 to 50 mg Mn kg−1 in the dry matter in mature leaves (Jones Citation1998). The straw Mn concentrations in ‘Koshihikari Kan No. 1’ grown at the nine sites were within this range or higher. Since the average grain yield did not differ significantly between ‘Koshihikari’ and ‘Koshihikari Kan No. 1’ in any of the water regimes (), despite large differences in their grain and straw Mn contents (), we hypothesize that rice may not require high amounts of Mn for normal growth.
Relative to the yield of ‘Koshihikari’ in the FLD regime, the grain yield of ‘Koshihikari Kan No. 1’ decreased significantly (by 14.8%) in the AWD treatment at site B and by 14.4% and 22.3% in the WAS treatment at sites B and E, respectively (). The average yield decreased by 2% in the AWD regime and by 4–6% in the WAS regime, although these reductions were not statistically significant (), suggesting that the AWD and WAS regimes might have a negative effect on grain yield at some sites. Linquist et al. (Citation2015) observed a similar trend, with a 5–13% reduction in yield, depending on the AWD treatment. The reductions in grain yield are likely to be accompanied by decreases in grain quality, which we evaluated using the whole-grain ratio (). Due to the effects of high temperatures in 2013, ‘Koshihikari Kan No. 1’ had a high ratio of chalky and immature grains in the AWD treatment at site B and in the WAS treatment at sites B and E (data not shown). On the other hand, ‘Koshihikari Kan No. 1’ had a larger yield than ‘Koshihikari’ in all water regimes at sites D and G. The grain yield in rice is affected by many environmental factors, including soil texture, soil fertility, temperature, the quantity of solar radiation, and rainfall. The geographical differences in yield can be explained by complex interactions among these factors. It is important to note that our results were obtained in field tests in a single year at each site. Since grain yield is a highly variable agronomic trait that responds strongly to climate characteristics, interannual variation is likely to be high, and the effects of the present water regimes and experimental sites on grain yields should be confirmed by continuing the tests for multiple years.
Grain quality is an important trait for rice production. First-grade rice requires a whole-grain ratio greater than 70% in Japan. The FLD regime can prevent or mitigate the effects of high temperature on ripening of rice grains and can therefore preserve the grain quality (Chiba et al. Citation2011). Unfortunately, ‘Koshihikari’ is sensitive to high temperatures, and the grain quality in the present study () was considerably worse than usual in 2013 due to a long heat wave. Because site D is located in northeastern Japan, the effect of this heat wave was considerably less than at the other sites. The average whole-grain ratio in the AWD regime was similar to that in the FLD regime, but the WAS regime produced a lower whole-grain ratio in both cultivars: by 2.8 percentage points for ‘Koshihikari’ and 7.2 percentage points for ‘Koshihikari Kan No. 1’ compared with the control (‘Koshihikari’ in FLD). These results may indicate a deterioration of grain quality caused by water stress. Since the whole-grain ratio of ‘Koshihikari Kan No. 1’ is somewhat lower than that of ‘Koshihikari’ even in the FLD regime, some mutation in an allele involved in grain quality may exist in ‘Koshihikari Kan No. 1’.
We found that inorganic As accounted for an average of 94.5% of the total As in brown rice (Fig. S2). This was comparable to the value obtained during an MAFF survey in Japan, in which inorganic As accounted for an average of 92% of total As in brown rice (n = 600; MAFF Citation2014). In addition, the survey showed that the average ratio of As in polished rice to that in brown rice was 63% for total As and 61% for inorganic As. Although we did not measure inorganic As in the polished rice, the survey data suggest that all treatments and sites except the FLD regime at site B would not exceed 0.2 mg kg−1 of inorganic As in the polished rice. However, we should nonetheless try to reduce As in rice grains to the lowest levels possible to prevent the associated health risks.
To simultaneously reduce As and Cd concentrations in rice grains, our results suggest three feasible choices based on using the low-Cd cultivar ‘Koshihikari Kan No. 1’: (1) For rice cultivation in Andosols, the water regime may not be very important because of the low grain As concentrations in rice even in the FLD regime; (2) At many sites, the AWD regime will be preferable because it decreases the grain As concentrations while minimizing the effect on grain yield and grain quality compared to the FLD regime; (3) The sites where the grain As concentration is likely to be high, such as site B, should be cultivated using the WAS regime, but this treatment may inevitably reduce grain yield and quality.
In conclusion, our study is the first to demonstrate that the low-Cd rice cultivar ‘Koshihikari Kan No. 1’ can be grown in the AWD and WAS regimes, allowing farmers to simultaneously decrease As and Cd concentrations in the rice grains. Our findings provide an important tool for reducing As and Cd concentrations in rice and thereby reducing exposure to both elements via the food chain.
TSSP_A_1144452_Supplementary_material.docx
Download MS Word (373.6 KB)Acknowledgments
This work was supported by a grant from the MAFF of Japan (Research project for improving food safety and animal health, As-110). We thank H. Tanikawa, H. Yamaguchi, H. Iino, T. Kamata, M. Iino, A. Arai, Y. Yamanaka and M. Chiba of the National Institute for Agro-Environmental Sciences for their field and laboratory assistance.
Supplementary material
Supplementary material for this article is available online from http://dx.doi.org/10.1080/00380768.2016.1144452
References
- Ahmed ZU, Panaullah GM, Gauch H, McCouch SR, Tyagi W, Kabir MS, Duxbury JM 2011: Genotype and environment effects on rice (Oryza sativa L.) grain arsenic concentration in Bangladesh. Plant Soil, 338, 367–382. doi:10.1007/s11104-010-0551-7
- Arao T, Kawasaki A, Baba K, Mori S, Matsumoto S 2009: Effects of water management on cadmium and arsenic accumulation and dimethylarsinic acid concentrations in Japanese rice. Environ. Sci. Technol., 43, 9361–9367. doi:10.1021/es9022738
- Baba K, Arao T, Yamaguchi N, Watanabe E, Eun H, Ishizaka M 2014: Chromatographic separation of arsenic species with pentafluorophenyl column and application to rice. J. Chromatogr. A, 1354, 109–116. doi:10.1016/j.chroma.2014.05.072
- Blakemore L, Searle P, Daly B 1981: Methods for chemical analysis of soils. New Zealand Soil Bureau Scientific Report 10A, New Zealand.
- Bolan NS, Makino T, Kunhikrishnan A, Kim PJ, Ishikawa S, Murakami M, Naidu R, Kirkham MB 2013: Cadmium contamination and its risk management in rice ecosystems. Adv. Agron., 119, 183–273. doi:10.1016/B978-0-12-407247-3.00004-4
- Chiba M, Matsumura O, Terao T, Takahashi Y, Watanabe H 2011: Mechanism of high quality rice production in deep-flood irrigation. J. J. Crop Sci., 80, 13–20. doi:10.1626/jcs.80.13 (in Japanese with English summary).
- Classification Committee of Cultivated Soils 1996: Classification of Cultivated Soils in Japan — third Approximation. National Institute for Agro-Environmental Science, Tsukuba.
- Codex Alimentarius Commission 2014: CODEX STAN 193-1995, General standard for contaminants and toxins in food and feed. http://foodnara.go.kr/codex/download.do?addPath=/hubfiles/codex/data2&fileName=STAN%20193-1995.pdf&fileNameOri=STAN%20193-1995.pdf ( Available at October 2015)
- Gambrell RP 1996: Manganese. In Methods of Soil Analysis Part3, Chemical methods, eds. Sparks, DL et al., pp. 665–682. Soil Science Society of America, Inc., Madison.
- Garrity DP, O’Toole JC 1995: Selection for reproductive stage drought avoidance in rice, using infrared thermometry. Agron. J., 87, 773–779. doi:10.2134/agronj1995.00021962008700040027x
- Gee GW, Bauder JW 1986: Particle size analysis. In Methods of Soil Analysis, Part 1, Physical and Mineralogical Methods, ed. Klute, A, pp. 383–411. American Society of Agronomy, Soil Science Society of America, Madison.
- Grant CA, Bailey LD, McLaughlin MJ, Singh BR 1999: Management factors which influence cadmium concentrations in crops. In Cadmium in Soil and Plants, eds. McLaughlin, MJ, Singh, BR, pp. 151–198. Kluwer Academic Publishers, the Netherlands.
- Hu PJ, Huang JX, Ouyang YNet al. 2013: Water management affects arsenic and cadmium accumulation in different rice cultivars. Environ. Geochem. Health, 35, 767–778. doi:10.1007/s10653-013-9533-z
- Ishikawa S, Ae N, Murakami M, Wagatsuma T 2006: Is Brassica juncea a suitable plant for phytoremediation of cadmium in soils with moderately low cadmium contamination? Possibility of using other plant species for Cd-phytoextraction. Soil Sci. Plant Nutr, 52, 32–42. doi:10.1111/j.1747-0765.2006.00008.x
- Ishikawa S, Ishimaru Y, Igura M, Kuramata M, Abe T, Senoura T, Hase Y, Arao T, Nishizawa NK, Nakanishi H 2012: Ion-beam irradiation, gene identification, and marker-assisted breeding in the development of low-cadmium rice. Proc. Natl. Acad. Sci. U. S. A., 109, 19166–19171. doi:10.1073/pnas.1211132109
- Islam FS, Gault AG, Boothman C, Polya DA, Charnock JM, Chatterjee D, Lloyd JR 2004: Role of metal-reducing bacteria in arsenic release from Bengal delta sediments. Nature, 430, 68–71. doi:10.1038/nature02638
- Jones JB Jr 1998: The Micronutrients. In PLANT NUTRITION Manual, ed. Jones Jr., JB, pp. 55–76. CRC Press, New York.
- Kuramata M, Abe T, Kawasaki A, Ebana K, Shibaya T, Yano M, Ishikawa S 2013: Genetic diversity of arsenic accumulation in rice and QTL analysis of methylated arsenic in rice grains. Rice, 6, 3. doi:10.1186/1939-8433-6-3
- Kuramata M, Abe T, Matsumoto S, Ishikawa S 2011: Arsenic accumulation and speciation in Japanese paddy rice cultivars. Soil Sci. Plant Nutr, 57, 248–258. doi:10.1080/00380768.2011.565479
- Linquist BA, Anders MM, Adviento-Borbe MAA, Chaney RL, Nalley LL, Da Rosa EFF, Van Kessel C 2015: Reducing greenhouse gas emissions, water use, and grain arsenic levels in rice systems. Global Change Biol, 21, 407–417. doi:10.1111/gcb.12701
- Liu CP, Yu H-Y, Liu CS, Lie FB, Xu XH, Wang Q 2015: Arsenic availability in rice from a mining area: is amorphous iron oxide-bound arsenic a source or sink? Environ. Pollut, 199, 95–101. doi:10.1016/j.envpol.2015.01.025
- Lombi E, Sletten RS, Wenzel WW 2000: Sequentially extracted arsenic from different size fractions of contaminated soils. Water Air Soil Poll, 124, 319–332. doi:10.1023/A:1005230628958
- Ma JF, Yamaji N, Mitani N, Xu XY, Su YH, McGrath SP, Zhao FJ 2008: Transporters of arsenite in rice and their role in arsenic accumulation in rice grain. Proc. Natl. Acad. Sci. U. S. A., 105, 9931–9935. doi:10.1073/pnas.0802361105
- MAFF (Ministry of Agriculture, Forestry and Fisheries of Japan) 2014: Survey of arsenic levels in brown rice and polished rice produced in Japan. http://www.maff.go.jp/j/press/syouan/nouan/pdf/140221-01.pdf (in Japanese, available at October, 2015)
- Makino T, Sugahara K, Sakurai Y, Takano H, Kamiya T, Sasaki K, Itou T, Sekiya N 2006: Remediation of cadmium contamination in paddy soils by washing with chemicals: Selection of washing chemicals. Environ. Pollut., 144, 2–10. doi:10.1016/j.envpol.2006.01.017
- Meharg AA, Norton G, Deacon Cet al. 2013: Variation in rice cadmium related to human exposure. Environ. Sci. Technol., 47, 5613–5618. doi:10.1021/es400521h
- Minamikawa K, Fumoto T, Itoh M, Hayano M, Sudo S, Yagi K 2014: Potential of prolonged midseason drainage for reducing methane emission from rice paddies in Japan: a long-term simulation using the DNDC-rice model. Biol Fert Soils, 50, 879–889. doi:10.1007/s00374-014-0909-8
- Moreno-Jiménez E, Meharg AA, Smolders E, Manzano R, Becerra D, Sanchez-Llerena J, Albarran A, Lopez-Pinero A 2014: Sprinkler irrigation of rice fields reduces grain arsenic but enhances cadmium. Sci. Total Environ., 485, 468–473. doi:10.1016/j.scitotenv.2014.03.106
- Nonaka K, Takahashi K 1988: A method of measuring available silicates in paddy soils. Jpn. Agric. Res. Q., 22, 91–95.
- Norton GJ, Duan GL, Dasgupta Tet al. 2009: Environmental and genetic control of arsenic accumulation and speciation in rice grain: comparing a range of common cultivars grown in contaminated sites across Bangladesh, China, and India. Environ. Sci. Technol., 43, 8381–8386. doi:10.1021/es901844q
- Sahoo PK, Kim K 2013: A review of the arsenic concentration in paddy rice from the perspective of geoscience. Geosci. J., 17, 107–122. doi:10.1007/s12303-013-0004-4
- Seyfferth AL, Webb SM, Andrews JC, Fendorf S 2011: Defining the distribution of arsenic species and plant nutrients in rice (Oryza sativa L.) from the root to the grain. Geochim. Cosmochim. Ac., 75, 6655–6671. doi:10.1016/j.gca.2011.06.029
- Spanu A, Daga L, Orlandoni AM, Sanna G 2012: The role of irrigation techniques in arsenic bioaccumulation in rice (Oryza sativa L.). Environ. Sci. Technol., 46, 8333–8340. doi:10.1021/es300636d
- Sumner ME, Miller WP 1996: Cation exchange capacity and exchange coefficients. In Methods of Soil Analysis Part3, Chemical Methods, Eds. Sparks, DL et al., pp. 1201–1229. Soil Science Society of America, Inc., Madison.
- Takahashi Y, Minamikawa R, Hattori KH, Kurishima K, Kihou N, Yuita K 2004: Arsenic behavior in paddy fields during the cycle of flooded and non-flooded periods. Environ. Sci. Technol., 38, 1038–1044. doi:10.1021/es034383n
- Takata Y, Ito T, Ohkura T, Obara H, Kohyama K, Shirato Y 2011: Phosphate adsorption coefficient can improve the validity of RothC model for Andosols. Soil Sci. Plant Nutr., 57, 421–428. doi:10.1080/00380768.2011.584510
- Takeuchi Y, Hori K, Suzuki K et al. 2008, Major QTLs for eating quality of an elite Japanese rice cultivar, Koshihikari, on the short arm of chromosome 3. Breed. Sci, 58, 437–445. doi:10.1270/jsbbs.58.437
- Williams PN, Villada A, Deacon C, Raab A, Figuerola J, Green AJ, Feldmann J, Meharg AA 2007: Greatly enhanced arsenic shoot assimilation in rice leads to elevated grain levels compared to wheat and barley. Environ. Sci. Technol., 41, 6854–6859. doi:10.1021/es070627i
- Yamaguchi N, Ohkura T, Takahashi Y, Maejima Y, Arao T 2014: Arsenic distribution and speciation near rice roots influenced by iron plaques and redox conditions of the soil matrix. Environ. Sci. Technol., 48, 1549–1556. doi:10.1021/es402739a
- Yamaji N, Sasaki A, Xia JX, Yokosho K, Ma JF 2013: A node-based switch for preferential distribution of manganese in rice. Nat. Commun, 4, 2442. doi:10.1038/ncomms3442