Abstract
A modified particle-into-liquid sampler (PILS), based on the original design of CitationWeber et al. (2001), is presented. The principal modification in this design is that collected liquid sample is delivered to vials held on a rotating carousel as opposed to an on-line analytical detector. A model is developed to predict aerosol mass concentrations measured by a PILS based on operating parameters and characteristics of the sampled aerosol. A backward model predicts the concentrations of the sampled aerosol based on operating parameters and concentrations measured by the PILS. Both models, which consider plumbing transmission efficiencies, droplet growth, mixing effects, and volatilization losses, predict mass concentrations that are consistent with laboratory tests for step changes in concentration. The average collection efficiency for species (Na + , K + , SO 4 2− , Cl − , NO 3 − ) from a variety of aerosols compared to simultaneous measurements with a differential mobility analyzer (DMA) exceeded 96% except for NH 4 + (88%); NH4 + is theoretically shown to be the most vulnerable to volatilization, followed by Cl − and then NO 3 − , with greater losses caused by increasing droplet pH and temperature. The characterization tests highlight the importance of reducing NH 4 + volatilization by keeping a stable tip temperature of 100°C at the point where steam and ambient air mix in the condensation chamber. Maintaining a stable tip temperature also avoids fluctuations in supersaturations that lead to increased deposition losses of larger droplets. Sample data from the 2004 International Consortium for Atmospheric Research on Transport and Transformation (ICARTT) field campaign are presented.
INTRODUCTION
Instrumental methods for quantifying atmospheric aerosol chemical composition have evolved from filter-based sampling to devices that provide real-time measurements (CitationBuhr et al. 1995; CitationSimon and Dasgupta 1995; CitationZellweger et al. 1999; CitationStolzenburg and Hering 2000; CitationSlanina et al. 2001; CitationWeber et al. 2001; CitationOrsini et al. 2003). Real-time measurements avoid both the potential sampling artifacts and long turn-around time for processed results associated with filter sampling. CitationWeber et al. (2001) developed the original particle-into-liquid sampler (PILS), which grows particles in supersaturated water vapor, thereby creating droplets sufficiently large to be collected by inertial impaction and then chemically analyzed in real-time. The liquid sample can be partitioned into fractions and analyzed by different analytical techniques, such as ion chromatography (IC) and total organic carbon (TOC) analysis.
We report here on a PILS, developed by Brechtel Manufacturing Inc. (www.brechtel.com), which is based on a modification of the original design of CitationWeber et al. (2001). The principal modification in this design is that two syringe pumps drive the liquid sample from the impaction plate at the end of the condensation chamber to vials held on a rotating carousel; in the original PILS design, liquid sample is driven directly from the impaction plate to an analytical detector with either a peristaltic pump or two syringe pumps downstream of the analytical device. Benefits of syringe pumps over peristaltic pumps include steadier flow rates and less degradation in pump tubing over time. The sampling rate of this modified PILS is not limited by the duty cycle time of any on-line analytical detector, which is advantageous for airborne sampling.
A model is needed to predict sample behavior in both the original and modified PILS in order to quantify effects such as volatilization losses and mixing. We present here a mathematical model to simulate the major processes affecting the aerosol sample within the PILS. A model of the instrument is developed that, given the input aerosol concentration, predicts the mass concentration in successive vials collected; the model incorporates plumbing efficiencies, droplet growth, evaporation of semi-volatile species, and the residence time distribution in the debubbler and two syringes that drive the liquid sample to vials. These same processes are considered in a backward model, intended to predict the aerosol mass concentration entering the inlet based on observed concentrations within vials. The two models are used to simulate operation of both the modified and the original PILS, where the step involving the syringes is eliminated when considering the original PILS. Model predictions are compared to data obtained from characterization tests with the original and modified PILS, which include evaluation of the PILS collection efficiency using a differential mobility analyzer (DMA) while sampling a variety of different laboratory aerosols. Sample data from the 2004 International Consortium for Atmospheric Research on Transport and Transformation (ICARTT) field campaign are presented to show that the modified PILS can successfully quantify ambient concentrations of inorganic and organic acid ions with time resolution appropriate for aircraft sampling.
MODIFIED PILS
The basic principle of the PILS instrument is to grow aerosol particles into droplets sufficiently large to be collected by inertial impaction for subsequent chemical analysis (CitationWeber et al. 2001). Many of the instrumental features of the modified PILS design presented here are the same as those described by CitationOrsini et al. (2003). As shown in , the modified PILS samples ambient air at approximately 12.5 L min−1, with a critical orifice maintaining a constant volumetric flow rate. A single-stage pre-impactor (D 50 = 1 μm) removes super-micrometer sized particles. An automated three-way valve (Swagelok) either directs flow through (filter mode) or bypasses (sample mode) a high efficiency particulate air (HEPA) filter to allow for background level testing during experiments. A series of three denuders remove gases that can bias aerosol measurements; inorganic vapors are removed by two denuders with annular glass surfaces (URG-2000-30 × 242-3CSS), and a third denuder (Sunset Laboratory Inc.), composed of 15 thin carbon filter paper sheets (3.15 cm × 20.32 cm × 0.04 cm thick) with 0.2 cm gaps between them, removes organic gases. The glass denuders are placed upstream of the carbon denuder so that detailed extraction tests can be carried out following operation.
The ambient air mixes with steam that is introduced through a tube (0.04 cm ID), the tip of which has a thermocouple attached to provide accurate steam injection tip temperature readings. An algorithm is used to account for pressure fluctuations (specifically for airborne operation) and air flow over the tip, to provide real-time control of the steam injection tip temperature at 100 ± 2°C. Rapid adiabatic mixing of the steam and the cooler ambient air produces a high supersaturation of water vapor that allows droplets to grow large enough (D p > 1 μ m) to be collected by inertial impaction onto a wettable quartz or polycarbonate impactor plate. The condensation chamber is kept at a slight angle (15ˆ) to allow wall condensate to be removed by a drain line at the end of the chamber's main body. Those particles too small (< 1 μ m) to be collected by inertial impaction leave the system with the exhausted air flow.
Impacted droplets are transported down the impactor by a wash-flow (0.15 to 0.20 mL min−1) supplied by two syringe pumps with 250 μ L syringes (Kloehn Ltd. Model V3), which work together using a “handshaking” technique. Using this technique, one syringe aspirates sample from a wash-flow stock bottle in a downward stroke, while the other is dispensing already-collected wash-flow to the top of the impactor plate in an upward stroke. A stainless steel mesh wick on the perimeter aids in drawing the liquid down the impactor plate. Two syringes use the “handshaking” technique to extract the liquid sample from the bottom of the impactor plate (0.13 mL min−1) and deliver it to a debubbler; volumes of 250 and 50 μ L were used for these syringes (these syringes, regardless of volume, are operated at the same speed, 2.17 μ L s−1), with the lower volume reducing the mean liquid residence time in the PILS. The liquid flow rates are controlled by the programmed syringe pump speeds. After air bubbles are removed by the debubbler, the liquid sample is drawn into one of two syringes (at any one time, one syringe aspirates liquid from the debubbler and the other dispenses liquid to the vials); the debubbler and each syringe behave like two continuously stirred tank reactors (CSTRs) in series. These types of vessels are characterized by perfect mixing, such that the concentration is uniform throughout the vessel. The sample then travels to a computer-controlled injection needle that is inserted into individual vials, the caps of which have septa to minimize contamination. Seventy-two vials are held on a rotating carousel; typically 0.65 mL of sample was collected over a 5-min period in each vial for results presented here. The sample volume and filling time for each vial can be adjusted to optimize the level of dilution and time resolution needed. The addition of syringe pumps and the vial collection technique are beneficial for aircraft sampling since the liquid sample in each vial can be partitioned into fractions for different analytical tests after each flight. Furthermore, the use of a vial collection system avoids the space, power, weight, and down-time of an analytical device while promoting autonomous operation.
Dilution occurs when the impacted droplets are washed from the impactor plate. In addition to the wash-flow, dilution takes place as a result of water vapor condensation on the impactor wall. The dilution factor is determined by spiking the wash-flow of Milli-Q water with non-interfering ions (LiF and LiBr were used for this study); this factor, which typically ranges from 1.1 to 1.4 during airborne flights, is calculated by taking the ratio of the Li+ concentration in the wash-flow supply bottle to the Li+ concentration in each vial. The pressure changes that occur during aircraft flights have been shown to have no influence on the dilution factor since the syringe pumps allow for precise liquid sample flow control.
To make meaningful intercomparisons with other instruments sampling the same air stream simultaneously, it is necessary to know the residence time of an air parcel and liquid sample inside the PILS system (). The debubbler and the syringes complicate this measurement since they act as CSTRs and mix each incoming liquid parcel with their entire liquid volume. An estimate of the residence time in the mixing vessels is made by observing the time needed to empty them when they are completely filled with liquid. All other plumbing residence times are calculated using measured tube volumes and flow rates. The time elapsed from the moment a parcel of air enters the inlet until it impacts onto the droplet impactor plate is estimated to be 3.6 s; this time will vary between different designs based on the plumbing length between the inlet and the droplet impactor (combined 3 m in this design with tube IDs varying between 0.35 and 1.65 cm). The time for each syringe to complete a full round-trip (downward stroke followed by an upward stroke) is measured to be 46 and 230 s using 50 and 250 μ L syringes, respectively. The last liquid parcel to enter a syringe at the end of a downward stroke exits the syringe at the beginning of the next upward stroke, which results in very little time spent in the syringe. As will be shown later, model predictions show that there is a minimum time delay of 301 s and 304–313 s to overcome a step change in concentration for the original and modified PILS (assuming a 66 s debubbler liquid residence time), respectively; the time delays for the modified PILS are used here as a time-offset to intercompare with DMA measurements. The time-offset can be reduced by lowering the debubbler residence time; this involves optimizing the ratio between the flow rate of the wash-flow at the top of the droplet impactor and the speed of the syringes aspirating liquid from the debubbler.
FIG. 2 Modeled stages of the modified PILS. The “P” and “D” markers indicate which equations from are applied in the designated stages of the PILS. At any one time, only one of the syringes is dispensing sample to a vial in an upward stroke (the right syringe just finished an upward stroke, and will now begin a downward stroke) while the other aspirates sample from the debubbler in a downward stroke. The value of τ reported for the debubbler represents the time required to empty it when it is completely filled with liquid, while τ for the syringes represents the cumulative time for a downward and upward stroke. The original PILS sends liquid sample from the debubbler immediately to an analytical detector, such as an IC system, with the liquid driven by a pump downstream of the analytical device.
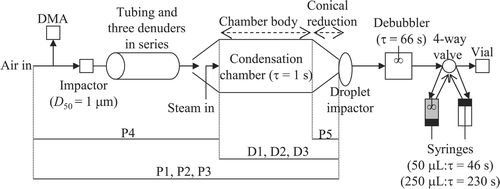
PILS MODEL
A model is developed that predicts the mass of solutes in the PILS vials. The model accounts for plumbing transmission efficiencies, droplet growth, loss processes in the condensation chamber including volatilization and droplet deposition, mixing behavior in the debubbler and the syringes, and the “handshaking” behavior of the syringes. This model also predicts the transition time resulting from a step change in concentration for different operating parameters, such as vial-filling time, mixing vessel volumes, and the round-trip time for the syringes. The inverse model starts from measurements of concentration in vials to back-calculate the actual mass concentrations in the sampled aerosol and to properly adjust the PILS measurements for instrument time response. Both models are also used to simulate sample behavior in the original PILS, with the only difference being that the syringes were not considered.
The important flow elements of the modified PILS are the impactors, the plumbing leading to the condensation chamber, the condensation chamber itself, and the plumbing leading from the droplet impactor to the dispensing needle, which includes the debubbler and two syringes (). During sample transport within the instrument, plumbing-related particle losses can occur by gravitational settling, diffusional deposition, turbulent inertial deposition, and inertial deposition at bends and flow constrictions. The model considers these processes as a function of particle size for all stages up to the droplet impactor, at which point the masses in each particle size bin are added into a cumulative liquid parcel. Predictions can be used to assess the sensitivity of the droplet size distribution to different initial conditions for the air and water vapor streams.
Model Equations
The basic equations of the PILS model are given in ( indicates which equations are applied when modeling specific stages of the instrument). Semi-volatile species in the sampled particles can evaporate as a result of the release of latent heat of condensation and convective heating of the air sample. CitationMa (2004) reports a 13 to 16% loss (by mass) of NH4 + based on PILS (original design) intercomparisons with filter samples obtained during four selected ground-based and airborne studies. For the range of particle surface temperatures examined (25–130°C), NH4 + is the most volatile, followed by Cl−, and then NO3 − (). To estimate the volatilization of these three species during PILS operation, we calculate the quantity of particle-phase NH4 +, Cl−, and NO3 − that will be converted to the gas phase in the condensation chamber. It is assumed that before the sampled aerosol reaches the condensation chamber, the denuders remove all of the gas-phase species (NH3, HCl, and HNO3). The general assumption is made for the equilibria represented in that ambient volatile species are in equilibrium between the gas and particle phase, which has been argued to be especially valid for sub-micrometer particles (CitationPilinis and Seinfeld 1987; CitationZhang et al. 2002); however, this assumption has been shown not to hold under all ambient conditions (CitationHildemann et al. 1984; CitationQuinn et al. 1992; CitationParmar et al. 2001; CitationTrebs et al. 2005).
FIG. 3 Temperature dependence of the equilibrium constants for these three reactions: NH3 (g) + H2O (aq)


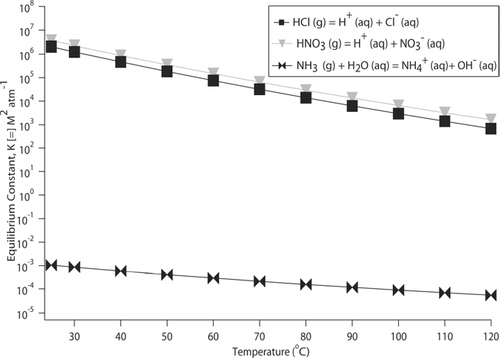
TABLE 1 Equations for droplet growth and transmission efficiency in different stages of the PILSFootnote a
After the condensation chamber and the droplet impactor, the liquid sample travels through the debubbler, syringes, and 0.5 mm ID PEEK tubing prior to reaching the dispensing needle. Although no aerosol mass is expected to be lost in these final flow components, mixing occurs in the debubbler and syringes. It is assumed that each liquid parcel drawn into a mixing vessel immediately mixes with the bulk liquid volume and that the mass concentration of the effluent is equal to the uniform concentration inside the vessel.
Model Predictions
Because the PILS operates with an upstream impactor to remove super-micrometer particles, we examine theoretical losses for sub-micrometer particles in the plumbing up to the condensation chamber (). Losses are predicted to decrease from 2.21 to 0.42% for particles with diameters ranging from 0.01 to 0.08 μ m, respectively. Losses subsequently increase from 0.42 to 8.58% for diameters increasing from 0.08 to 1.0 μ m, respectively. Diffusional losses dominate the total losses for the smaller particle range, ranging from 95 to 57% of the total losses, whereas inertial deposition dominates for the larger particle range, ranging from 43 to 98% of the total losses. Inertial deposition at bends, of which 12 are present in this modified PILS design, dominate the inertial losses.
FIG. 4 Transmission efficiency in the PILS as a function of particle diameter for all plumbing prior to droplet growth in the condensation chamber, and the relative contribution of diffusion and inertial deposition to the total losses.
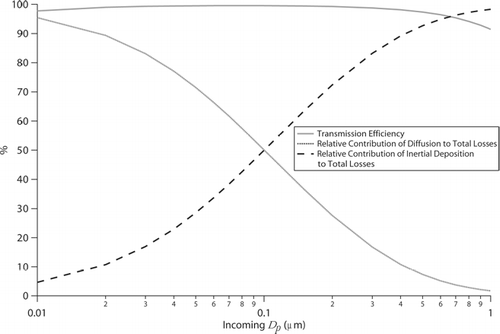
Losses in the condensation chamber are predicted as a function of particle size entering the chamber (). As the steam temperature increases, the supersaturation increases, resulting in larger final droplet diameters in the condensation chamber. The final droplet size is more sensitive to the supersaturation ratio achieved by having higher tip temperatures than to the initial particle size entering the condensation chamber. The value of the water condensation mass accommodation coefficient (α) is shown to have a significant impact on the predicted final size of the droplets; as this value increases, water vapor is more effectively scavenged during condensational growth. There is considerable scatter in experimental measurements for this parameter; values ranging from 0.04 to 1 are applied in current cloud model studies (CitationKreidenweis et al. 2003). CitationWeber et al. (2001) report that particles in the condenser reach final diameters of nominally 2 to 3 μ m, while CitationOrsini et al. (2003) measured a typical droplet size distribution from sampling room air and observed the range of droplet diameters to be between 1 and 5 μ m (with the majority of the droplets between 2 and 3 μ m). CitationSullivan et al. (2004) report that droplet diameters within the PILS can reach up to between 3 and 5 μ m, which is consistent with previous studies. The droplet diameters reported in the three aforementioned studies, the two most recent of which used the same steam condensation chamber as our PILS, are consistent with model predictions assuming an accommodation coefficient between 0.01 and 0.05 and a tip temperature of 100°C ().
FIG. 5 Final droplet diameters after growth in the condensation chamber (τ = 1 s) as a function of the initial particle diameters for different (a) accommodation coefficients (b) and tip temperatures. The (a) total transmission efficiency in the chamber is shown in addition to the (b) relative contribution of different parts of the chamber to the total losses. The final droplet size is more sensitive to both the tip temperature and accommodation coefficient compared to the initial particle size entering the condensation chamber.
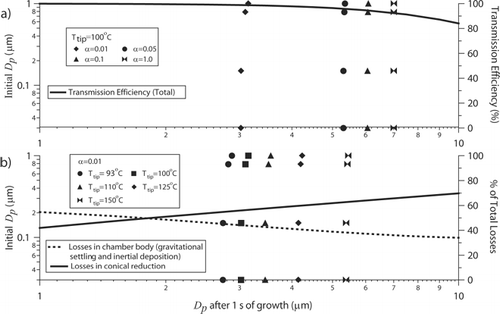
Losses during the droplet growth stage are dominated by gravitational settling and inertial deposition in the main body of the condensation chamber and in the conical section at the end of the chamber (these regions are defined in ). The losses exceed 1 and 10% when droplets reach sizes of 3 and 8 μm, respectively (). shows that losses in the conical section exceed those in the main body when droplets grow larger than 2 μ m. These results emphasize the importance of keeping the PILS tip temperature close to 100°C to minimize the growth of larger droplets that are more effectively lost by deposition prior to reaching the droplet impactor.
Calculations indicate that volatilization effects are most sensitive to temperature and the acidity of the sampled particles. shows the amount of NH4 + expected to remain in the particle phase at the end of the condensation chamber as a function of temperature and acidity. Ammonium is the sole ion vulnerable to volatilization over the span of temperatures, acidity, and dilution factors shown, whereas Cl− and NO3 − losses are predicted to be less than 1%. Ammonium losses are higher as the temperature increases and as the droplet acidity decreases.
FIG. 6 Percentage of NH4 + remaining in the particle phase after the 1 s residence time in the condensation chamber as a function of particle surface temperature, acidity, and the amount of water (which is related to the dilution factor). Each marker reflects a dilution factor of 1.25 where the upper and lower bounds reflect dilution factors of 1.4 and 1.1, respectively. Nitrate and chloride results are not shown since they are predicted to stay completely (100%) in the particle phase for all temperatures, acidities, and dilution factors considered here.
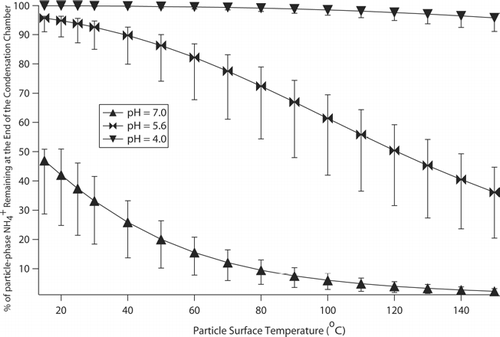
PILS-IC DETECTION LEVELS
Ion Chromatography (IC) System
The technique of choice for analyzing the liquid sample is IC. Two Dionex ICS-2000 systems (25 μ L sample loops) are used for performing off-line quantitative speciated inorganic and organic analysis. A Dionex AS-40 Autosampler feeds samples, which are in 0.5 mL vials with filter-caps, to the anion system (AS-11 column 2 × 250 mm, ASRS Ultra II 2-mm suppressor, potassium hydroxide eluent) first, which is equipped with a de-gas unit to rid the injected sample of any air bubbles that can amplify baseline noise. The anion unit has a 6-port injection valve that splits off part of the sample to the cation system (CS12A column 2 × 250 mm, CSRS Ultra II 2-mm suppressor, methanesulfonic acid eluent).
PILS-IC Technique Background Levels
The additional steps required in the off-line analysis of samples in this design require background level testing to check for contamination; sources of contamination include the Milli-Q water used in the wash-flow, plumbing in the PILS, the PILS and IC vials, including sample transfer between them, and the IC system itself. The background levels of individual species concentrations for analyzed filter samples (n > 300), presented as the average concentration plus three times the standard deviation (σ), are between 0.02 and 0.28 μ g m−3 (). It should be noted that the contribution from the IC alone to these background levels (calculated as the average concentration plus three times σ of the smallest detectable peak for each ion in the IC baseline noise and then converted to air-equivalent units) is less than 0.05 μ g m−3 for all major inorganic ions (NH4 +, K+, Mg2+, SO4 2−, Cl−, NO2 −, and NO3 −) except Na+ and Ca2+, which have IC background levels of 0.10 μ g m−3. To minimize background levels, the droplet impactor plate must be continuously cleaned (between each use of the system), the denuders should be frequently recoated, and the plumbing between the droplet impactor and dispensing needle should be periodically replaced.
TABLE 2 Filter mode background levels (n > 300)
Tests were performed to examine if any sinks or sources exist for different species (NH4 +, SO4 2−, or NO3 −) in the plumbing between the impactor plate and the dispensing needle. A wash-flow solution with known concentrations of either (NH4)2SO4 or NH4NO3 in water was used in place of the standard Milli-Q water solution spiked with LiBr or LiF. For each solution, several vials were collected while no steam was being generated (no droplets made) followed by steam generation. The tip temperature was controlled between 100 ± 2°C during steam production. This test would also reveal if heating of the impactor plate due to deposition of warm droplets and convective heating leads to volatility losses for any of the species in the wash-flow solutions. The collection efficiency in these tests is defined as the ratio of ion concentrations in liquid collected into vials to those in the wash-flow stock solution. The results in show that neither NH4 +, SO4 2−, or NO3 − is preferentially lost on the impactor plate, within the debubbler and syringes, or in the liquid lines before steam production, as the collection efficiency of each ion is approximately unity. During steam generation, volatility losses from the heated impactor plate are negligible since the molar ratios are within the measurement uncertainty (one σ) of the expected values of two and one for (NH4)2SO4 and NH4NO3, respectively. However, the one hour duration of these tests leaves open the possibility that higher tip temperatures and longer periods of heating may lead to increased volatilization losses due to heating of the impactor plate.
TABLE 3 Effect of using (NH4)2SO4 and NH4NO3 independently as wash-flow solutions (NH4 + collection efficiency is the total average of both wash solution tests)
EVALUATION OF MODEL WITH LABORATORY DATA
compares observed and model-predicted normalized mass concentrations from tests in which the PILS was cycled between sample and filter mode, thereby inducing a step change in mass concentration in the sampled air. The sampled particles were composed of Na2SO4 with a unimodal size distribution (number concentration mode at 70 nm with a geometric σ of 1.6). Losses due to volatility were not expected since SO4 2− and Na+ represent relatively non-volatile species. The forward model simulated the operation of the modified PILS for 50 and 250 μ L syringes, in addition to the original PILS, which does not employ syringes. For a specific debubbler residence time, the predicted time delay between when a step change is made and when the modified PILS reaches stable concentrations (defined as less than a 1% change by mass between two successive vials) varies according to the syringe round-trip time; for a debubbler residence time of 66 s, the minimum time delays for 50 and 250 μ L syringes are predicted to be 304 and 313 s, respectively (). Variations in the minimum time delays predicted are seen for single simulations considering successive step changes due to the position of the two syringes when one of them draws in the first liquid parcel following the step change in concentration; variations ranged between 10 and 60 s for simulations with the 50 and 250 μ L syringes, respectively. Data and predictions indicate that it usually takes two “transition vials” (at 5 min each with a debubbler residence time of 66 s) using 50 and 250 μ L syringes, during which the concentration gradually increases or decreases depending on the direction of the step change, before stable mass concentrations are reached (); however, predictions and observations occasionally show that only one transition vial is needed for 50 μ L syringes. The minimum time delay for the original PILS, assuming the same characteristic residence time in the debubbler, is predicted to be 301 s (). The minimum time delays predicted for the original and modified PILS designs are within 12 s of each other, indicating that mixing in the debubbler has a dominant effect on the time response of each design to a step change in concentration. Thus, the addition of syringes in the liquid flow path of the modified design does not significantly increase the minimum time response to concentration changes; however, the syringes have a broadening effect on the range of the time delay during each successive step change in concentration. To decrease the time response, the residence time needs to be minimized in the mixing vessels. shows that as the debubbler mixing volume is reduced, resulting in lower residence times, the minimum time response also drops substantially. also shows modified PILS observations and predictions from a step change test where 1-min vials were collected and the debubbler residence time was reduced to 15 s (using 50 μ L syringes with a 15 s round-trip time); one or two 1-min vials are needed prior to stabilization with good agreement between observations and predictions. Predictions for the original PILS show that for an assumed 1-min duty cycle time for an online analytical detector and a debubbler residence time of 15 s, one transition vial is needed prior to stabilization during step changes.
FIG. 7 Comparison of experimental and theoretical mass concentrations in the modified PILS. Model predictions are also shown for the original PILS, which does not include syringes in the liquid sample's path from the debubbler to the analytical detector. All concentrations have been normalized to the maximum concentration applied during the step changes. The time response is shown to be dramatically improved with shorter debubbler residence times (top right), where the residence time is taken to be the time required to empty the vessel when it is completely filled with liquid during normal operation; the 50 μ L syringes were set to operate at three times their usual speed (15 s round-trip) and the flow rate ratio between the wash-flow at the top of the droplet impactor and the liquid being aspirated into the syringes from the debubbler was reduced to 1.01, resulting in less liquid volume being held in the debubbler.
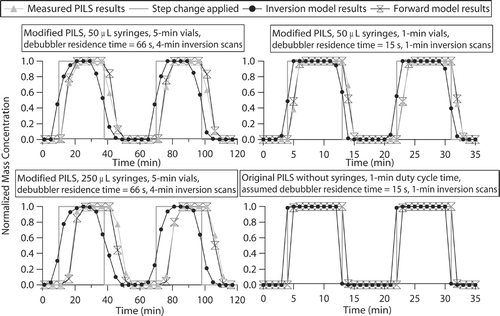
TABLE 4 Minimum time response (defined as the minimum time before successive mass concentrations vary by less than 1%) to step changes in concentration for the original and modified PILS designs as a function of the debubbler residence time
After a step increase in concentration, the normalized PILS levels observed indicate that mass losses are less than 1%. This is consistent with mass losses predicted by the forward model for droplet diameters up to 3 μ m. Mass losses of less than 3.5% are predicted for droplets with diameters between 3 and 5 μ m. CitationOrsini et al. (2003) report that the overall collection efficiency for operation at 15 L min−1 is better than 95% for particles with diameters between 0.03 and 6.0 μ m, which is consistent with our model predictions.
The experimental PILS mass measurements from the step change tests are now used as inputs to the inversion model to back-calculate the time-adjusted mass concentrations of the sampled aerosol (predicted mass measurements from the forward model are used as the input for the original PILS). The goal of the inverse model is to accurately predict the maximum and minimum concentrations attained during the step changes and to minimize the transition time during step changes by effectively deconvoluting mixing effects. The results of the inverse model are reported either as 1 or 4-min scans, where these times represent a broad range of representative DMA scanning times. This allows model predictions to be compared to DMA mass concentrations for experiments where the aerosol density is known. For 50 and 250 μ L syringes (assuming a debubbler residence time of 66 s), between two and four 4-min transition scans are needed before the PILS-measured concentrations stabilize and match the step function concentration change. Fewer scans are usually needed prior to stabilization for the 50 μ L syringes compared to 250 μ L syringes because fewer experimental transition vials are considered, and the mixing time is significantly shorter in the 50 μ L syringes (46 s round-trip versus 230 s for 50 and 250 μ L syringes, respectively). For the modified PILS test with 1-min vials and the shorter debubbler residence time (15 s), predictions showed that two or three 1-min scans are needed prior to stabilization. It is predicted that only one 1-min scan is needed for the original PILS to reach stable concentrations with a 15 s debubbler residence time and a 1-min duty cycle time. After the period of transition, the model accurately predicts the minimum and maximum concentrations associated with the step changes to within 1% for both the modified and original PILS.
PILS Collection Efficiency for Different Aerosol Seeds
To determine the PILS collection efficiency for aerosols of different composition and to compare the model with experimental results, the modified PILS sampled a variety of laboratory aerosols in parallel with a DMA. The aerosols studied include (NH4)2SO4, NH4NO3, NaCl, Na2SO4, K2SO4, and KNO3. A constant rate atomizer generated particles that were dried before reaching two 210Po neutralizers and then injected into a 28 m3 teflon chamber. A source of constant concentration aerosol allowed for direct comparison between the PILS, DMA, and model predictions. The relative humidity (RH) inside the chamber was maintained at less than 6% and the temperature was kept at 20°C (Vaisala HMP230 series transmitters). The PILS tip temperature was maintained at 100 ± 2°C for these tests. Complete number distributions (10–800 nm) were provided with 4-min frequency by a cylindrical scanning electrical mobility spectrometer, connected to a TSI model 3760 condensation nuclei counter. On average, the size distribution of the atomized seed aerosol particles was unimodal, with a number concentration mode centered between 70 and 100 nm and a geometric σ between 1.5 and 1.7. Using appropriate corrections for particle charging, DMA transfer function, and other scanning voltage correction factors, data from the DMA can be used to calculate a total aerosol volume concentration in the aerosol flow. Because the RH was less than 6% for all tests, the pure seed aerosol densities could be used to convert from volume to mass concentration. The DMA-derived masses were used to determine the relative contributions of cations and anions present based on the measured anion-to-cation ratios in the atomized solutions. The aerosols listed above were used to study the volatilization effects for NH4 +, Cl−, and NO3 −. The collection efficiency of two of the aerosols tested, (NH4)2SO4 and Na2SO4, were also measured by CitationMa (2004) in a laboratory setting using a similar approach with the original PILS.
The collection efficiency of the PILS compared to the DMA and the molar ratios measured in the PILS vials, as total averages, are shown in and , respectively. The total aerosol mass loadings for these tests ranged between 1 and 140 μ g m−3, with no significant variation in the collection efficiency as a function of mass loading for all ions studied. The PILS measurements are within 4% of the DMA-derived mass concentrations for K+, Na+, SO4 2−, Cl−, and NO3 −. The collection efficiency of NH4 +, however, is approximately 88% for the two ammonium-containing aerosols studied. The measured molar ratios are near the expected values for each seed aerosol tested, with the exception of ammonium-containing aerosols, where the ratio is lower than expected, confirming that NH4 + is vulnerable to volatilization loss within the PILS system. Measured collection efficiencies of Cl− and NO3 − were on the order of 96 to 97%, suggesting that they are less susceptible to volatilization losses as compared to NH4 +. These results are consistent with thermodynamic data shown earlier (), indicating that over the temperature span of PILS operation, NH4 + is the ion most susceptible to volatilization.
TABLE 5 PILS collection efficiency for anions and cations compared to the DMA; a shape factor of 1.08 was applied to the DMA data for NaCl (CitationHameri et al. 2001)
TABLE 6 Molar ratios for different aerosols tested by the PILS
CitationMa (2004) reports that when (NH4)2SO4 was generated and sampled by a PILS in laboratory tests, the mean NH4 +:SO4 2− molar ratio showed that NH4 + was undermeasured by approximately 10 ± 10%. When Na2SO4, a relatively non-volatile compound, was tested, the mean Na+:SO4 2− ratio was 2.03 ± 0.06. A comparison of the results from these two tests provides further evidence that NH4 + volatilization occurs in the PILS. Furthermore, the observed molar ratios from the Na2SO4 and (NH4)2SO4 laboratory tests show agreement between this study and that of CitationMa (2004).
Theoretical calculations for NH4 + losses in the PILS in show consistency with the experimental observations. CitationMa (2004) showed that the pH for PILS-collected liquid with dissolved particle sample can be 5.6, which is consistent with measurements obtained with the current PILS. Assuming a pH of 5.6 as an average for droplets composed of ammonium-containing aerosol particles, calculations predict that 5 to 15% and 7 to 20% of the total NH4 + is lost by volatilization for particle surface temperatures between 30 and 40°C, respectively, for a range of dilution factors between 1.1 and 1.4 (this range is representative of field and laboratory test conditions). These two particle surface temperatures represent a range of values predicted by the PILS model for the operating conditions in these tests. Therefore, the bars shown in should be used as a range for the predicted NH4 + loss. The experimentally determined 12% loss of NH4 + is in the range of the predicted values for the expected operating conditions in the condensation chamber.
Tip Temperature Sensitivity Tests
Several tests were performed to determine the influence of the tip temperature on the sampling efficiency of aerosols composed of (NH4)2SO4, NH4NO3, NaCl, Na2SO4, K2SO4, and KNO3. Vials were collected while the tip temperature was maintained at 100 ± 2°C. The tip temperature was then allowed to increase to between 120 and 150°C for at least 20 min before decreasing and restabilizing at 100 ± 2°C, during which time vials were continuously being collected.
shows results from a representative test performed using (NH4)2SO4 aerosol. Prior to the intense heating, the collection efficiency of NH4 + and SO4 2− are 90 and 100%, respectively. When the tip temperature was increased to 147°C, the collection efficiency for both species dropped to between 30 and 35% before returning to their original values when the tip temperature was reduced to 100°C. During the period after the tip returned to 100 ± 2°C, NH4 + initially exhibited a collection efficiency between 86 and 91% (between 0 and 35 min after the tip returned to 100 ± 2°C), but stabilized at 91% for the last two vials collected 70 and 75 min after the tip returned to 100 ± 2°C. The collection efficiency of SO4 2− was consistently between 98 and 101% after the intense heating. The increased sensitivity of NH4 + to the intense heating immediately after the tip temperature was lowered to 100 ± 2°C is due to the heated condensation chamber and impactor plate, leading to additional volatilization losses before the temperature decreased. The general response of NH4 + to the excessive heating shown in is consistent with that observed for the other five aerosols listed above.
FIG. 8 Tip temperature sensitivity results for a test where the modified PILS sampled (NH4)2SO4 aerosol. During the intense heating (from 10 to 45 min), the tip reaches up to 147°C and the NH4 + and SO4 2−mass collection efficiency levels drop significantly. The molar ratio is slightly less than two whenever the tip temperature is held at 100 ± 2°C, mainly due to NH4 + volatilization.
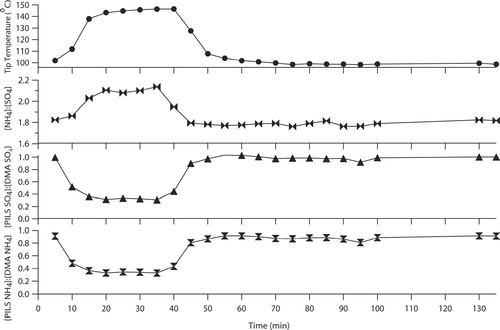
These results show that as the tip temperature is increased, particles grow to a sufficiently large size that deposition results in substantial droplet losses in the condensation chamber, specifically in the conical reduction region leading to the impactor jet. The initial decrease in species concentrations may, to a lesser extent, be partly associated with increased condensation of water vapor onto the droplet impactor and resultant dilution, since the temperature difference between the impactor plate and the mixed flow of steam and air is higher just after the tip temperature increases. During the intense heating, the increased deposition losses explain the observed decreases in collection efficiency of non-volatile species such as Na+, K+, and SO4 2−. Also, intense heating in the condensation chamber was shown to lead to additional volatilization losses for NH4 + (exceeding those shown in ) even when the tip temperature was allowed to return to normal operating values, since the chamber and the impactor plate remained at elevated temperatures over one hour after the intense heating tests were completed. These results emphasize the importance of maintaining the tip temperature at 100°C to minimize volatilization and deposition in the condensation chamber.
The PILS model developed here underestimates losses in the condensation chamber if deposition is primarily responsible for increased losses during the intense heating periods. The model predicts collection efficiencies of 84 and 66% for droplet diameters of 10 and 15 μ m, respectively. The droplet diameters predicted for tip temperatures ranging from 93 to 150°C in b are all less than 6 μ m, assuming an accommodation coefficient of 0.01. Larger droplet sizes would be predicted with larger values of the accommodation coefficient, yielding better agreement between measurements and predictions for particle losses; droplets are predicted to grow to 11 μ m at a tip temperature of 150°C and assuming an accommodation coefficient of 1. If the accommodation coefficient has a temperature dependence, this could explain both the increased droplet growth at higher tip temperatures and the significant droplet losses predicted. The equations used to predict droplet losses in the PILS, particularly the conical reduction section, are likely underpredicting the losses for the larger droplets.
FIELD DATA
A modified PILS has been successfully deployed in two field missions and the reader is referred to CitationSorooshian et al. (2006) for an in-depth analysis of data collected during the 2004 ICARTT field campaign. Flight tracks and data from one flight during ICARTT are shown in where the Center for Interdisciplinary Remotely-Piloted Aircraft Studies (CIRPAS) Twin Otter research aircraft flew transect legs downwind of the Conesville Power Plant (Coshocton County, Ohio) in clouds. There was significant SO4 2− growth with increasing distance downwind of the plant due to conversion of SO2 to SO4 2−. The ammonium-to-sulfate molar ratio was between 1.1 and 1.6 for most of the flight downwind of the plant, indicating that there was insufficient ammonia to neutralize the relatively high level of sulfuric acid. The high level of acidity can also explain why virtually no NO3 − was detected (< 0.3 μ g/m3). Oxalate and SO4 2− are strongly correlated and aqueous-phase precursors to oxalic acid, including glyoxylate, were detected indicating that aqueous-phase processing in cloud led to the elevated levels of oxalate measured during this flight. The results show that the modified PILS design is effective at quantifying ambient mass concentrations of both inorganic and organic acid ions with 5-min time resolution.
FIG. 9 (a) Flight tracks; (b) Time series of the mass concentrations of various inorganic and organic acid ions measured during one ICARTT flight that was marked by the presence of clouds. The shaded areas denote when the Twin Otter aircraft was flying downwind of the Conesville Power Plant. The aircraft flew transect legs perpendicular to the studied plume, with increasing distance downwind of the power plant with time.
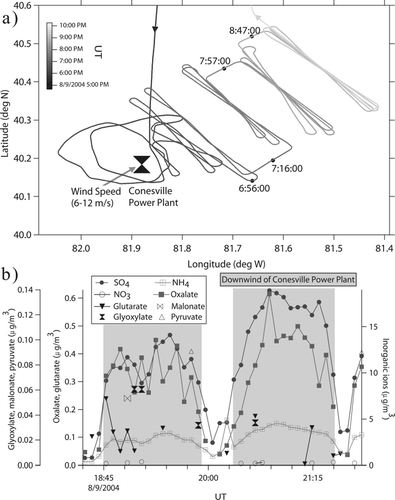
FUTURE WORK
There are several ways to improve the design of the PILS. The time response of the instrument to concentration changes can be improved by minimizing the liquid residence time in mixing vessels, primarily the debubbler and the syringes (only used in the modified design). With the modified PILS, syringe volumes can be reduced and they can be driven at higher speeds to minimize both the unpumped liquid volume in the debubbler and the residence time in the syringes. PILS users can correct for volatilization losses with correction factors (error bars) based on experimental results, such as those presented here for NH4 +. The instrument can also be optimized with respect to sampling organic aerosol both in laboratory tests and ambient field studies. Longer IC programs can be used to improve resolution of organic peaks by storing liquid samples in vials for subsequent off-line chemical analysis versus the limited time for chromatographic separations of the on-line systems (CitationWeber et al. 2001). It should be noted that the original PILS can perform longer IC programs as well, however, this is only practical for ground-based sampling since users must sacrifice time resolution during airborne flights. The PILS can also be coupled to other analytical detectors to quantify organics other than the organic acids that the IC can detect. A new technique described by CitationSullivan et al. (2004) couples the PILS to a TOC analyzer in order to quantify water-soluble organic carbon (WSOC). Techniques such as this increase the fraction of the total particulate organic mass that the PILS can speciate and quantify.
CONCLUSIONS
This study has introduced a modified PILS, as well as a model of the PILS sampling behavior. The main modification in this PILS is the implementation of syringe pumps to drive the liquid sample flow from the impactor to vials held on a rotating carousel. The instrument's time resolution is not limited by the duty cycle time of any on-line analytical technique, which is advantageous for airborne studies; this allows for a variety of analytical tests to be performed on an individual liquid sample, including longer IC gradient programs to resolve more organic peaks. This design of the PILS is also well-suited for remote ground-based operation as it requires less monitoring compared to a PILS connected to an analytical instrument.
An instrument model is developed that takes into account mixing effects, plumbing efficiencies, droplet growth, evaporation of semi-volatile species, and the “handshaking” technique of the syringes. When considering multiple step changes in the aerosol sample, the model accurately predicts experimentally measured concentrations in PILS vials. It was shown that for the modified PILS configurations used in this study (50 and 250 μ L syringes with a debubbler residence time of 66 s) that one or two 5-min transition vials are needed before stable concentrations (± 1% by mass) are reached in successive vials. However, when the debubbler residence time is reduced to 15 s and 1-min vials are collected using 50 μ L syringes, only one transition vial is needed. The minimum predicted time delay prior to reaching stabilization after a step change is between 301 and 313 s for two modified PILS configurations and the original PILS (assuming a debubbler residence time of 66 s), suggesting that mixing in the debubbler is mainly responsible for the time delay. An inverse model is also developed to back-calculate the actual time-history of aerosol concentrations entering the PILS based on the measured concentrations. The predictions show intermediate agreement with experimental data from the modified PILS, with the main issue being that the model cannot completely deconvolute the mixing effects within the PILS during the transition between step changes. However, after the transition period in the forward and inversion models, predictions and experimental data for the aerosol mass concentrations agree within 1%.
Results from laboratory characterization tests of the sampling efficiency and volatilization losses within the PILS have been compared to model predictions and good agreement has been found. Characterization tests show that the average modified PILS mass collection efficiency, as compared to the DMA-derived mass for a variety of aerosols exceeds 96% with the exception of NH4 +, which exhibits a collection efficiency of 88% (n = 369) for (NH4)2SO4 and NH4NO3. Results from similar tests conducted with an original PILS for (NH4)2SO4 and Na2SO4 aerosols agree with those observed with the modified PILS. When considering the operating conditions in the condensation chamber, it is predicted that between 5 and 20% of the particle-phase NH4 + is converted to gas-phase NH3, which is consistent with experimental results. The temperature at the point where steam and the aerosol sample mix is an important parameter that must be properly controlled to prevent significant particle losses. If the tip temperature is too high, the increase in supersaturation produces larger droplets that are more effectively lost by deposition in the condensation chamber. Volatilization losses of NH4 + are also increased under high tip temperature conditions. Data from 369 samples of laboratory-generated (NH4)2SO4 at different mass loadings suggest that PILS users should apply a correction factor of 1.14 (1/0.88) to their NH4 + results to account for volatility losses (when the tip is in the operating range of 100 ± 2°C). However, this factor should be used with caution since NH4 + volatilization is theoretically shown to be sensitive to droplet pH and ambient samples do not necessarily behave like laboratory-generated (NH4)2SO4 samples. Field data from ICARTT 2004 show that the modified PILS is successful at quantifying ambient concentrations of inorganic and organic acid ions with 5-min time resolution.
This work was supported by the National Science Foundation grant ATM-0340832 and the Office of Naval Research.
Notes
a Laminar and turbulent flow are distinguished by calculating the Reynolds number (Re = ρ g gUd/η), where ρ g , η, U, and d are the density and viscosity of the gas, characteristic gas velocity, and tubing dimension, respectively. The transmission efficiency of the two impactors, which are obtained from theoretical impactor efficiency curves, depend on the jet Reynolds number, the ratio of the jet-to-plate distance and the nozzle diameter (values are given in ), and the Stokes number, Stk = ρ p C c D 2 p U/9 η W, where ρ p and D p are the particle density and diameter, respectively. The slip correction factor, C c , accounts for noncontinuum effects, η is the air viscosity, W is the nozzle diameter, and U is the average air velocity at the nozzle exiting point. It is assumed that the droplets that do impact on the second impactor are collected. CitationHangal and Willeke (1990) argue that there are no inertial transmission losses for sub-isokinetic isoaxial sampling; no losses are assumed to occur in the conical expansion at the beginning of the condensation chamber.
b The labels under this column are shown as markers on to indicate which equations are applied when modeling specific stages of the PILS. “P” denotes a plumbing transmission efficiency equation and “D” denotes a droplet growth equation.
a The debubbler residence time is taken to be the time required to empty it when it is completely filled with liquid during normal operation.
REFERENCES
- Buhr , S. M. , Buhr , M. P. , Fehsenfeld , F. C. , Holloway , J. S. , Karst , U. , Norton , R. B. , Parrish , D. D. and Sievers , R. E. 1995 . Development of a Semicontinuous Method for the Measurement of Nitric-Acid Vapor and Particulate Nitrate and Sulfate . Atmos. Environ , 29 (19) : 2609 – 2624 . [CSA]
- Crane , R. L. and Evans , R. L. 1977 . Inertial Deposition of Particles in a Bent Pipe . J. Aerosol Sci , 8 : 161 – 170 . [CROSSREF] [CSA]
- Fuchs , N. A. 1964 . The Mechanics of Aerosols , Pergamon : Oxford .
- Gormley , P. G. and Kennedy , M. 1949 . Diffusion from a Stream Flowing Through a Cylindrical Tube . Proc. R. Irish Acad , 52A : 163 – 169 . [CSA]
- Hameri , K. , Laaksonen , A. , Vakeva , M. and Suni , T. 2001 . Hygroscopic Growth of Ultrafine Sodium Chloride Particles . J. Geophys. Res , 106 (D18) : 20749 – 20757 . [CROSSREF] [CSA]
- Hangal , S. and Willeke , K. 1990 . Overall Efficiency of Tubular Inlets Sampling at 0-90 Degrees from Horizontal Aerosol Flows . Atmos. Environ , 24A : 2379 – 2386 . [CSA]
- Hildemann , L. M. , Russell , A. G. and Cass , G. R. 1984 . Ammonia and Nitric-Acid Concentrations in Equilibrium with Atmospheric Aerosols—Experiment Vs Theory . Atmos. Environ , 18 (9) : 1737 – 1750 . [CSA]
- Kreidenweis , S. M. , Walcek , C. J. , Feingold , G. , Gong , W. , Jacobson , M. Z. , Kim , C. -H. , Liu , X. , Penner , J. E. , Nenes , A. and Seinfeld , J. H. 2003 . Modification of Aerosol Mass and Size Distribution due to Aqueous-phase SO2 Oxidation in Clouds: Comparison of Several Models . J. Geophys. Res. , 108 (D7) : 4213 doi:10.1029/2002JD002697.[CROSSREF] [CSA]
- Ma , Y. 2004 . Developments and Improvements to the Particle-Into-Liquid Sampler (PILS) and its Application to Asian Outflow Studies, Ph.D. Dissertation , Atlanta, GA : Georgia Institute of Technology .
- Muyshondt , A. , McFarland , A. R. and Anand , N. K. 1996 . Deposition of Aerosol Particles in Contraction Fittings . Aerosol Sci. Technol , 24 : 205 – 216 . [CSA]
- Orsini , D. A. , Ma , Y. , Sullivan , A. , Sierau , B. , Baumann , K. and Weber , R. J. 2003 . Refinements to the Particle-Into-Liquid Sampler (PILS) for Ground and Airborne Measurements of Water Soluble Aerosol Composition . Atmos. Environ , 37 : 1243 – 1259 . [CROSSREF] [CSA]
- Parmar , R. S. , Satsangi , G. S. , Lakhani , A. , Srivastava , S. S. and Prakash , S. 2001 . Simultaneous Measurements of Ammonia and Nitric Acid in Ambient Air at Agra (27 degrees 10′ N and 78 degrees 05′ E) (India) . Atmos. Environ , 35 (34) : 5979 – 5988 . [CROSSREF] [CSA]
- Pilinis , C. and Seinfeld , J. H. 1987 . Continued Development of a General Equilibrium-Model for Inorganic Multicomponent Atmospheric Aerosols . Atmos. Environ , 21 : 2453 – 2466 . [CROSSREF] [CSA]
- Pui , D. Y. H. , Romay-Novas , F. and Liu , B. Y. H. 1987 . Experimental Study of Particle Deposition in Bends of Circular Cross Section . Aerosol Sci. Technol , 7 : 301 – 315 . [CSA]
- Quinn , P. K. , Asher , W. E. and Charlson , R. J. 1992 . Equilibria of the Marine Multiphase Ammonia System . J. Atmos. Chem. , 14 (1–4) : 11 – 30 . [CROSSREF] [CSA]
- Schwendiman , L. C. , Stegen , G. E. and Glissmeyer , J. A. 1975 . Report BNWL-SA-5138 , Richland, Washington : Battelle Pacific Northwest Laboratory .
- Seinfeld , J. H. and Pandis , S. N. 1998 . Atmospheric Chemistry and Physics , New York : Wiley-Interscience .
- Simon , P. K. and Dasgupta , P. K. 1995 . Continuous Automated Measurement of the Soluble Fraction of Atmospheric Particulate Matter . Anal. Chem. , 67 (1) : 71 – 78 . [CROSSREF] [CSA]
- Slanina , J. , ten Brink , H. M. , Otjes , R. P. , Even , A. , Jongejan , P. , Khlystov , A. , Waijers-Ijpelaan , A. and Hu , M. 2001 . The Continuous Analysis of Nitrate and Ammonium in Aerosols by the Steam Jet Aerosol Collector (SJAC): Extension and Validation of the Methodology . Atmos. Environ , 35 (13) : 2319 – 2330 . [CROSSREF] [CSA]
- Sorooshian , A. , Varutbangkul , V. , Brechtel , F. J. , Ervens , B. , Feingold , G. , Bahreini , R. , Murphy , S. M. , Holloway , J. S. , Atlas , E. L. , Buzorius , G. , Jonsson , H. , Flagan , R. C. and Seinfeld , J. H. 2006 . Oxalic Acid in Clear and Cloudy Atmospheres: Analysis of Data from ICARTT 2004 . J. Geophys. Res. , [CSA]
- Stolzenburg , M. R. and Hering , S. V. 2000 . Method for the Automated Measurement of Fine Particle Nitrate in the Atmosphere . Environ. Sci. Technol. , 34 (5) : 907 – 914 . [CROSSREF] [CSA]
- Sullivan , A. P. , Weber , R. J. , Clements , A. L. , Turner , J. R. , Bae , M. S. and Schauer , J. J. 2004 . A Method for On-Line Measurement of Water-Soluble Organic Carbon in Ambient Aerosol Particles: Results from an Urban Site . Geophys. Res. Lett , 31 : L13105 [CROSSREF] [CSA]
- Thomas , J. W. 1958 . J. Air Pollut. Control Assoc , 8 : 32 [CSA]
- Trebs , I. , Metzger , S. , Meixner , F. X. , Helas , G. N. , Hoffer , A. , Rudich , Y. , Falkovich , A. H. , Moura , M. A. L. , da Silva , R. S. , Artaxo , P. , Slanina , J. and Andreae , M. O. 2005 . The NH4+-NO3–Cl–SO42–H2O Aerosol System and its Gas Phase Precursors at a Pasture Site in the Amazon Basin: How relevant are Mineral Cations and Soluble Organic Acids? . J. Geophys. Res. , 110 (D7) doi:10.1029/2004JD005478[CSA]
- Wang , J. , McNeill , V. F. , Collins , D. R. and Flagan , R. C. 2002 . Fast Mixing Condensation Nucleus Counter: Application to Rapid Scanning Differential Mobility Analyzer Measurements . Aerosol Sci. Technol , 36 : 678 – 689 . [CROSSREF] [CSA]
- Weber , R. J. , Orsini , D. , Daun , Y. , Lee , Y. N. , Klotz , P. J. and Brechtel , F. J. 2001 . A Particle-Into-Liquid Collector for Rapid Measurement of Aerosol Bulk Chemical Composition . Aerosol Sci. Technol , 35 : 718 – 727 . [CROSSREF] [CSA]
- Willeke , K. and Baron , P. A. 2001 . Aerosol Measurement: Principles, Techniques, and Applications , New York : Wiley-Interscience .
- Zellweger , C. , Ammann , M. , Hofer , P. and Baltensperger , U. 1999 . NOy Speciation with a Combined Wet Effluent Diffusion Denuder-aerosol Collector Coupled to Ion Chromatography . Atmos. Environ , 33 (7) : 1131 – 1140 . [CROSSREF] [CSA]
- Zhang , J. , Chameides , W. L. , Weber , R. J. , Cass , G. , Orsini , D. , Edgerton , E. , Jongejan , P. and Slanina , J. 2002 . An Evaluation of the Thermodynamic Equilibrium Assumption for Fine Particulate Composition: Nitrate and Ammonium During the 1999 Atlanta Supersite Experiment . J. Geophys. Res , 108 (D7) : 8414 doi:10.1029/2001JD001592[CROSSREF] [CSA]