Abstract
Although much progress has been made on single particle analysis by inductively-coupled plasma-mass spectrometer (ICP-MS), many problems remain for online analysis of environmental aerosol by ICP-MS, such as aerosol direct introduction and response time. In this article, an aerosol sample introduction interface coupled with ICP-MS for direct introduction and quantitative online monitoring of environmental aerosol has been developed and characterized. Through the interface, sampling of environmental aerosol, matching of the sampling aerosol flow and the finally introduced flow, conversion of carrier gas from air-to-air–argon mixture, removal of water vapor and matrix gas, could all be implemented. Moreover, it supports the standard solution nebulization and addition method by which the transport efficiency and the instrumental sensitivity are calibrated totally in real time. Online analytical performance of ICP-MS coupled with the interface was evaluated. Results show that the response time for online monitoring of environmental aerosol improves dramatically as the aerosol sampling flow increases from 100 mL/min to 1 L/min. The sensitivity is improved through condensation growth and concentration enrichment process. The background equivalent concentration of 238U in aerosol is 1.1 × 10−9 g/m3. As a preliminary comparison study, uranium concentration in environmental aerosol in a clean room was monitored by online and offline methods.
Copyright 2014 American Association for Aerosol Research
1. INTRODUCTION
As a powerful analytical tool, inductively-coupled plasma-mass spectrometer (ICP-MS) has been widely used for offline and online monitoring of environmental aerosol in many fields such as environmental protection, climate change, and national security. In offline monitoring technique, long sampling time and large sampling flow are essential to accumulate enough aerosol samples for chemical processing and subsequent analysis by ICP-MS or radioactivity spectrometry. Hence, good detection ability for elemental concentration in aerosols is achievable. For instance, the detection limit for plutonium concentration in environmental aerosol is on the order of 10−17 g/m3 for isotope-dilution-ICP-MS method with sampling time of 12 h, sampling flow of 80 m3/h (Zhou et al. Citation2002). Compared with online monitoring technique, the offline monitoring technique is laborious and time consuming. Moreover, the obtained concentration represents only the average level but not the temporal trend during the sampling period. Therefore, offline monitoring technique could not fulfill the needs such as emergence response and source identification.
Recently, much progress has been made on single particle analysis by ICP-MS and a technique named single particle mode (SPM) has been established. Micron-sized monodisperse particles were usually produced by vibrating-orifice-aerosol-generator (Nomizu et al. Citation1993, Citation2002; Su et al. Citation2009). Nanosized monodisperse particles were produced by electrical classifier commercial instruments, for instance, DMA (Myojo et al. Citation2002), DMA-APM (Suzuki et al. Citation2010) and ion mobility spectrometry (Kapellois and Pergantis Citation2012). These particles carried by argon as the working gas of ICP were finally introduced into the torch under appropriate conditions. The particle number concentration should be low enough that one ion pulse corresponding to one particle is easy to be distinguished from each other. From the frequency and intensity of the ion pulses, the introduced particle number concentration and the element amount contained in single particle could be calculated, respectively. It is reported that 10−16 g level of lead (Nomizu et al. Citation1993), zinc (Nomizu et al. Citation2002), and terbium (Su et al. Citation2009) in individual particle are detectable by using SPM-ICP-MS.
The analytical performance of single particle analysis by ICP-MS mainly depends on the performance of the mass spectrometer. In the technique, the introduction conditions are well controlled and the working gas of ICP (argon) is chosen as the carrier gas of particles. The process of online analysis of environmental aerosol by ICP-MS is much different and many problems remain. First, the aerosol flow introduced directly is restricted because the direct introduction of the carrier gas consisting of air and trace gases (carbon dioxide and water vapor) would cause serious matrix effect for mass spectrometer analysis. Second, sampling pump (Seltzer and Green Citation1994) or self-adsorption accessory (Nishiguchi et al. Citation2008) is necessary to drive the aerosol into the ICP torch because the ICP ion source and environmental aerosol are both under atmospheric pressure. However, the fluctuation of the aerosol sampling flow and its pressure would influence the sensitivity of the instrument. Third, the detected ion signals are often continuous and vary temporally as the particle number concentration is high but unstable. The purpose of online monitoring is to obtain the accurate mass–volume concentration of the element contained in the aerosol and its changing trend. To achieve online quantitative analysis of environmental aerosol by using ICP-MS, two key problems must be overcome: (1) the matching of the large sampling aerosol flow (∼1 L/min) and relative small introduced sample flow (∼100 mL/min); (2) the repression of the matrix effect and real-time calibration of the instrumental sensitivity.
In order to solve problem (1), several calibration methods have been proposed including standard particle method, metal gas addition method, and standard solution nebulization/addition method. The principle of the standard particle method is the same with single particle mode. However, its application is restricted by the poor availability of the standard particle with similar properties to environmental aerosol. The combined use of monodisperse aerosol generator and mass spectrometer is complex and the particles’ monodispersity needs to be monitored. In metal gas addition method, metal carbonyl gas is generated and mixed with the sampled aerosol for online analysis (Nishiguchi et al. Citation2008). The generation rate of the metal gas is determined prior to the real-time calibration of the mass spectrometer. However, only several kinds of metal carbonyl gases for Cr, W, and Mo are practicable up to now. Quantitative analysis of heavy metals aerosol (Pb, Zn, U, etc.) by this method is questionable because the analytical performances of elements between metal aerosol and metal gas are much different. In standard solution nebulization/addition method, continuous, submicron-sized particles carried by argon gas are produced by nebulization and desolvation. Its generation efficiency is determined by the filtration method developed previously (Su et al. Citation2011). This method is flexible and works well with ICP-MS. It has been verified and applied for quantitative analysis of uranium-containing individual particles (Su et al. Citation2010) and ultra-trace plutonium-containing aerosol (Li et al. Citation2011). Recently, the metal gas addition method and standard solution nebulization were used in combination for quantitative real-time monitoring of multi-elements in airborne particulates (Suzuki et al. Citation2012).
Several aerosol introduction interfaces coupled with ICP-AES/ICP-MS have been developed to match the sampling aerosol flow and the finally introduced flow. Virtual impactor was once utilized as the interface of ICP-AES (Meyer and Lee Citation1994). Part of the air–argon mixed gas carrying fly ash was extracted, and meanwhile particles of several microns were concentrated after the virtual impactor. Result shows that the detection limit (10−6 g/m3 for Cr concentration) is mainly restricted by the detection ability of ICP-AES. DMA commercial instrument was also used as the sample introduction interface for ICP-MS (Myojo et al. Citation2002). Through gas diffusion and exchange process in the interface, the carrier gas was converted from air to air–argon gas according to the flow ratio of intake aerosol (0.6 L/min) and clean argon (10 L/min). Meanwhile, the particles’ trajectories under electrical field still maintained the same and then followed the 0.6 L/min air–argon flow. However, restricted by the geometric design of DMA and diffusion coefficient of carrier gas, the exchange performance of CO2 and cyclohexane with argon in DMA is poor. The classified particle is smaller than 160 nm or else the carrier gas would breakdown under high electric voltage.
In 2008, a novel aerosol introduction interface which includes a gas converter as the key apparatus was presented (Nishiguchi et al. Citation2008). The gas converter consists of two concentric tubes: a porous silica inner tube and a borosilicate glass outer tube. The aerosol sample flows in the inner tube and the clean argon gas flows in the outer tube. Because there is partial pressure difference on both sides of the inner tube, the carrier gas and the argon could penetrate the porous membrane and exchange. The particles stay in the inner tube because of its small diffusion coefficient. Gas molecules (N2, CO2, and O2) in aerosol sample could be replaced by argon under appropriate conditions. Therefore, the matrix effect is suppressed which is beneficial for direct introduction of aerosol flow into ICP-MS. Because aerosol flow in one gas converter is recommended to be less than 250 mL/min, four gas converters operating in parallel are needed to achieve the aerosol sampling flow of 1 L/min. Although the nebulized/desolved particles were added online at the outlet of the gas converter (Suzuki et al. Citation2012), it is unable to correct the transport loss in the converter. This issue might be one reason that monitoring results of ICP-MS-based online monitoring technique for some elements were lower than those of the filter-based offline monitoring technique. In the previous work (Su et al. Citation2011), the remarkable influence of sweep gas on aerosol transport efficiency in membrane dryer has been observed. Thus, it is essential to correct the transport efficiency for aerosol flowing through the porous tube, such as the gas converter.
In this article, an aerosol sample introduction interface coupled with ICP-MS for direct introduction and quantitative online monitoring of environmental aerosol has been developed and characterized. Sampling of environmental aerosol, matching of the sampling flow and introducing flow, conversion of carrier gas from air-to-air–argon mixture, removal of water vapor and matrix gas could all be implemented through the interface. Moreover, the standard solution nebulization and addition method by which the transport efficiency and the instrumental sensitivity are calibrated totally in real-time is supported. As a preliminary comparison study, uranium concentration in environmental aerosol in the clean room, where ICP-MS was mounted, was monitored online by ICP-MS coupled with the interface and offline monitoring method based on liquid impinger.
2. EXPERIMENTAL
2.1. Design and Development of the Interface
The principle of the interface and its coupling with ICP-MS is shown in . The interface mainly consists of five modules: (I) environmental aerosol sampling module, (II) standard solution nebulization and desolvation module, (III) aerosol condensation growth module, (IV) aerosol concentration enrichment module, and (V) matrix gas removal module. It works as follows.
1. | About 1 L/min environmental aerosol sampled by a diaphragm pump is introduced into the saturator in the interface. | ||||
2. | Standard solution is nebulized and then desolvated to produce continuous droplets carried by argon gas (∼10 L/min). The calibration aerosol mixes with aerosol sample in the saturator and then they undergo the same processes. Hence, the transport efficiency and the instrumental sensitivity of the environmental aerosol could be represented by that of the calibration aerosol. | ||||
3. | For small particles that could not be enriched directly by the Round Virtual Impactor (RVI), saturator and condenser apparatuses for particle condensation growth are manufactured. Through the condensation of working fluid vapor onto the surface of particles, they could finally grow up to several microns suitable to be enriched in the RVI. | ||||
4. | RVI is developed to match the sampling flow and the introduction flow. About 90% of the input flow (major flow) is exhausted stably by a vacuum pump through a critical orifice. The remaining flow (minor flow) with an air–argon volume ratio of 1:9 is extracted for subsequent detection. Meanwhile, particles larger than the cutoff size of RVI (D 50) move forward and follow with minor flow because of their great inertia. Therefore, their number concentration is enriched and the detection ability is improved. | ||||
5. | In order to reduce transport loss of large particles and repress matrix effect, water and n-butanol (working fluid for condensation growth) contained in the particles are evaporated through tube heating and removed through membrane dryer. |
2.1.1. The Removal Performance of Membrane Dryer to Matrix Gas
In order to determine the flow ratio of environmental aerosol and calibration aerosol, the removal performance of membrane dryer to matrix gas was evaluated first. Indium nitrate standard solution (purchased from National Institute of Metrology of Beijing, China) with concentration of about 10−8 g/g was prepared and nebulized by micro-concentric nebulizer (Glass Expansion Inc., Melbourne, Australia). The droplets carried by 1 L/min argon was mixed with nitrogen, desolvated by the membrane dryer, and finally introduced to the torch of ICP-MS (Element, Finnigan MAT Inc., now part of Thermo Scientific Inc., FL, USA). The ion intensities at m/z = 115 under different nitrogen flows (0–100 ml/min) were monitored. The influence of nitrogen flow on the 115In intensity obtained by ICP-MS was investigated.
2.1.2. The Condensation Growth Performance in Saturator and Condenser
The principle of the condensation growth process in saturator and condenser is the same with that in n-butanol-based condensation particle counter (Ahn and Liu Citation1990). N-butanol was chosen as working fluid because it is more effective for hydrophobic particles than water. Besides, this type of condenser is compact in size.
The saturator is a column-type, stainless steel container with geometric size of Φ146 mm × H190 mm. About one-third of the container is filled with n-butanol liquid. The saturator is immersed in a water bath for uniform heating of n-butanol liquid with temperature of T s. The mixed aerosol flowing above the n-butanol liquid surface carries heated vapor and moves toward the condenser.
The condenser consists of two concentric stainless steel tubes. The wall of the inner tube (ID14 mm × L300 mm) is cooled by the coolant with temperature of T c flowing through the outer tube. The n-butanol vapor reaches oversaturation and condenses onto the surface of the particle for growth. The outer wall of the outer tube is covered by heat-resistant material. A membrane dryer (Aridus CETAC Inc., NE, USA) is installed downstream of the condenser. The tube between the dryer and the condenser is heated by a heating jacket at temperature of 90°C. Furthermore, the wall of the dryer's inlet is heated at 160°C to evaporate effectively water vapor and organic solvents (including n-butanol) with low boiling point. Finally, these vapors exchange with argon in sweep gas and are removed partly through porous membrane.
Indoor airborne aerosol sampled by a diaphragm pump (Model 5D1060, Gast Inc., MI, USA) was introduced to evaluate the condensation growth performance in saturator and condenser. The final size distribution of particles at the outlet of the condenser was monitored by laser particle sizer (Model 8810, AIFOM, Chinese Academy of Sciences), the principle of which is the same with aerodynamic particle sizer (Model 3321, TSI Inc., MN, USA). The influence of different conditions, including working fluid temperature, coolant temperature, and carrier gas flow rate (nebulization argon flow Q n plus dilution argon flow Q d), were investigated to find out a group of optimal conditions.
Subsequently, a suspension containing 560 nm polystyrene latex (PSL) standard particle (Polysciences Inc., PA, USA) was nebulized and carried by argon. The PSL particle concentration could be modulated by the regulation of nebulization argon flow as the suspension uptake rate varied accordingly. 0.8 L/min filtered air was pumped and introduced to simulate the effect of carrier gas of environmental aerosol. The final size distribution of particles was monitored by laser particle sizer. The flexibility of the module under the proposed conditions was verified by using PSL particles under different concentration loads.
2.1.3. Development of Round Virtual Impactor and its Enrichment Performance
RVI was designed following the principles proposed previously (Baron and Willeke 2002). Main characteristic parameters are listed in . The distance between the jet nozzle and the receiving nozzle could be adjusted by installation of O-ring of different thickness.
TABLE 1 Main characteristic parameters of round virtual impactor
The enrichment performance of RVI was measured as shown in . Monodisperse, fluorescent-labeled PSL suspension (Duke Inc., now part of Thermo Fisher Inc., CA, USA) was nebulized and carried by argon gas to produce test aerosol. It was then introduced into a chamber for uniform mixing and representative sampling. The upstream concentration and downstream concentration of RVI were determined at the same time to calculate the enrichment factor of RVI. As there is only one laser particle sizer in our lab, alternative measurement of upstream concentration and downstream concentration is troublesome in experiment. Instead, two BioSampler (BKC Inc., PA, USA) with about 20 mL collection liquids were utilized for simultaneous sampling, and so the parallel measurement of the aerosol concentration was converted to the relative measurement of fluorescent intensity in collection liquids after sampling. The test aerosol was collected by BioSampler 1# downstream of the sampling port “1” to obtain the upstream concentration. Meanwhile, RVI downstream of the sampling port “2” operated under nominal conditions. The enriched particles in its minor flow were collected by BioSampler 2# to obtain the downstream concentration. Clean argon gas was supplemented through a Y-type connector to fulfill the sampling flow of BioSampler 2# (∼10 L/min).
After sampling of about 20 min, the collection liquids in both BioSamplers were dispersed by ultrasonic cleaner for 20 min. The fluorescent intensities at wavelength of 433 nm were measured by fluorometer (960MC model, Shanghai Jingke Inc.). Then, the enrichment factor of RVI for particle of D p is
In which C 1 and C 2 are the aerosol concentrations at sampling port 1# and 2#, respectively. m 1, F 1, Q 1, and η 1 are the mass of the collection liquid, the fluorescent intensity, the sampling flow rate, and collection efficiency of BioSampler upstream of RVI, respectively. The parameters with subscript 2# correspond to those of BioSampler downstream of RVI. Q sp is the supplemented argon flow.
In order to determine the ratio of η 2/η 1, RVI and Y-type connector were both removed in , and then two BioSamplers were connected directly to the sampling ports for sampling. The ratio of aerosol concentration at sampling port 1# to that at sampling port 2# is determined by:
In which Q BS,1 and Q BS,2 are the sampling flow rates of BioSampler 1# and 2#, respectively. Exchange the sample positions of two BioSampler, while maintain other conditions the same. Then, the aerosol concentration ratio is
Divide Eq. (3) by Eq. (2) using C′2/C′1=C″2/C″1
2.2. Performance Evaluation of the Combined use of ICP-MS with the Interface
The generation efficiency of calibration aerosol was determined offline by the filter-based method (Su et al. Citation2011). Φ47 mm, 0.45 μm PTFE filter (Sartorius Inc., Goettingen, Germany) was placed in a lab-made filter holder and connected downstream to the diffusion dryer as shown in . The sampling flow was drawn by a diaphragm pump. Standard solution containing ytterbium and lutetium was nebulized and collected quantitatively (>99%) by the filter. The sample on the filter was washed off and transferred into a 2% (v/v) HNO3 solution for analysis. External standard method with ICP-MS was utilized to determine the concentration in the solution sample.
The aerosol sample introduction interface was constructed and utilized with ICP-MS as shown in . 233U standard solution was prepared and nebulized to produce the 233U-containing calibration aerosol. It was carried by 10 L/min argon and mixed with about 1 L/min filtered air. When the saturator and the condenser operated under different temperatures, the dependence of instrument response on the dilution argon flow was obtained. Hence, the influence of the introduction conditions on the performance of ICP-MS plus the interface was evaluated.
2.3. Preliminary Monitoring of Environmental Aerosol in the Clean Room
The ICP-MS instrument is installed in a 10,000-class clean room in which the concentration of particles larger than 0.3 μm is less than 1020/m3 [ISO 14644–1]. As a preliminary comparison study, the uranium concentration in environmental aerosol in this clean room was monitored by both offline and online methods.
First, two BioSamplers were used in parallel for offline sampling. The collection efficiencies for particles larger than 0.3 μm are higher than 75% (Willeke et al. Citation1998). Nitric acid (Xi’an Chemical Reagent Plant, China) was purified by sub-boiling distillation and then diluted to 2% HNO3 by 18.2 MΩ cm deionized water (Milli-Q system, Millipore Inc., MA, USA) prior to their use as collection liquid in the BioSampler. Therefore, the uranium background in the reagent was depressed by 1 order of magnitude. After aerosol sampling, the liquid was analyzed by ICP-MS without complex chemical processing. Hence, the contamination possibly introduced in the chemical process was eliminated. These steps are crucial for monitoring of ultra-trace uranium in environmental aerosol. In this work, about 20 mL purified 2% HNO3 (v/v) was used as collection liquid. After sampling, the liquid was dispersed by ultrasonic cleaner for 20 min. It was then nebulized and quantitatively analyzed by using external calibration method with ICP-MS. The 238U mass concentration in the offline sampled aerosol (C a,BS) was determined as
In which C 8 is the measured 238U concentration in the collection liquid, m BS is the liquid mass after aerosol sampling, η c is the collection efficiency, and t is the sampling time.
Then, the uranium concentration in environmental aerosol in the clean room was monitored online by ICP-MS coupled with the developed interface. Environmental aerosol of 0.8 L/min (Q a) was sampled and mixed with the calibration aerosol containing 233U. Under optimal introduction conditions, ion intensity at m/z = 233 (I 3) and 238 (I 8) were measured alternatively. The relationship is
So the 238U mass concentration in the sampled aerosol (C a,ICP) is
In which k is the mass discrimination factor of m/z = 238 to m/z = 233. C
st is the prepared concentration of the standard solution and Q
up is the liquid uptake rate of the nebulizer. and
are mass introduction rates of 238U in the environmental aerosol sample and 233U in calibration aerosol, respectively. η
t is the aerosol transport efficiency. Because the size distributions between the sampled aerosol and the calibration aerosol are similar, their transport efficiencies are approximately the same.
3. RESULTS AND DISCUSSION
3.1. The Optimal Flow Ratio of Environmental Aerosol and Calibration Aerosol
The mass introduction rate in the calibration aerosol is constant under the same generation condition. It is convenient to investigate the influence of introduced nitrogen flow on the instrumental sensitivity. As shown in , the signal intensity increases sharply as the nitrogen flow (Q N2) increases from 0 to 18 mL/min when the sweep gas flow (Q sweep) is 2.7 L/min. After the peak, the signal intensity decreases exponentially until Q N2 reaches about 100 mL/min. If the nitrogen flow is even larger, the plasma would be unstable and even extinguished which is unacceptable for analysis. The trends of the instrumental response at Q sweep = 2.5 L/min and 2.3 L/min are the same, except that the maximum value of the intensity decreases and the corresponding nitrogen flow increases.
FIG. 3 The influence of nitrogen flow rate on the ICP-MS response to the calibration aerosol carried by 1 L/min argon flow.
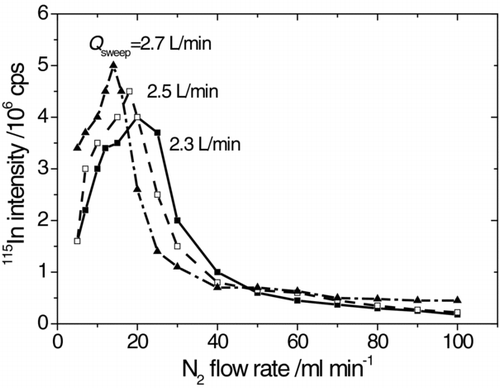
The instrumental response at Q N2 = 100 mL/min drops by 1 order of magnitude comparing with the peak value. Fortunately, it decreases slowly with the nitrogen flow around 100 mL/min. The flow ratio of the nitrogen flow (simulating the environmental aerosol) and the calibration aerosol is 0.1 L/min:1 L/min. Thus, the flow rates of the environmental aerosol and the calibration aerosol in the interface are designed to be 1 L/min and 10 L/min to make the dilution ratio the same. The finally introduced sample flow (1 L/min) is extracted as minor flow by RVI. In the gas converter developed by Suzuki, the recommended value of the environmental aerosol was 0.25 L/min, which was diluted by 0.8–1 L/min argon flow carrying the nebulized aerosol.
3.2. The Condensation Growth Performance for Indoor Airborne Particles
Indoor aerosol, with flow rate (Q a) of 0.8 L/min and temperature (T a) of 14.5°C, was sampled and introduced into the saturator. The mode diameter of the original distribution aerosol is 0.7 μm. The final size distributions of aerosol after growth under different temperature differences between the saturator and the condenser (dT = T s − T c) are shown in . The size distributions are all normalized for direct comparison. The particle growth begins to activate at dT = 15.1°C. As dT increases from 16.1°C to 17.9°C, the size distribution shifts to greater size and becomes narrow. The reason is that the super-saturation field of n-butanol in the condenser is enhanced to make the particles grow larger. It is also observed that the final size of particles decrease as the carrier gas flow increases. Because the super-saturation field of n-butanol in the condenser is weakened and the growth process is shortened.
FIG. 4 The final size distributions of indoor aerosol after growth under different temperature differences.
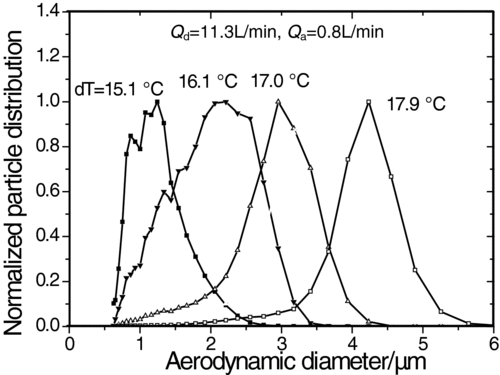
The total concentration of nebulized droplets containing 560 nm PSL particle increases with the square of the nebulization argon flow, as shown in . The droplet concentration increases from 300 cm−3 to 3400 cm−3 as Q n increases from 0.16 L/min to 0.23 L/min. Under conditions of Q d = 12.7 L/min and dT = 17.1°C, the final size reaches the range of 3–5 μm and shifts to the left as Q n increases. It is demonstrated that the particles could grow to the range of 3–4 μm which are larger than the cutoff size of RVI (about 2.5 μm) under the proposed conditions.
3.3. The Concentration Enrichment Performance of RVI
To evaluate the concentration enrichment performance of RVI to the grown particles in the range of 3–4 μm, fluorescent-labeled PSL particles with nominal diameter of 3.1 μm was nebulized. As the fluorescent-labeled PSL particles are not standard particles, product certificate is not provided by Duke. Hence, the accurate size and its true deviation are not known. Prior to their use after long-time storage, they were observed offline by scanning electron microscope. Statistical result from 34 particles shows that the relative standard deviation (RSD) of the diameters is 1.2%. The average value of the measured diameter agrees well with the nominal diameter and the relative error is less than 5%. The result demonstrates that these fluorescent-labeled PSL particles are monodisperse.
The measured sampling flow rates of the two BioSampler are Q BS,1 = 10.1 L/min and Q BS,2 = 10.4 L/min, respectively. Experimental results show that the relative collection efficiency of BioSampler 2# to BioSampler 1# for 3.1 μm particle is 90.2% (RSD = 5.1%). Because the ICP ion source is very sensitive to the sample introduction conditions, for instance, the directly introduced aerosol flow rate, the enrichment performance of RVI under different flow conditions was investigated experimentally. Result shows that the measured enrichment factor of RVI for 3.1 μm particle agrees with the theoretical value under different conditions. The average value of the ratio is 91% (RSD = 13.3%), which fulfils the design requirement.
Through particle growth in saturator/condenser and subsequent dynamic enrichment in RVI, inertial separation and concentration enrichment for particles from several nanometers to several micrometers could be achieved. Therefore, the detection ability and response time could be improved obviously. The transport loss due to gravitational settlement and impaction loss was not characterized specifically as the transport efficiency and the instrumental sensitivity are calibrated totally through online addition of reference aerosol by nebulization.
3.4. Online Analytical Performance Evaluation of ICP-MS Coupled with the Interface
The liquid uptake rate of the nebulizer (Q up) is 0.145 g/min (RSD = 6.4%). The generation efficiency of the calibration aerosol (η a) is 33.5% (RSD = 7.6%). The prepared concentration of 233U standard solution (C st) is 8.0 × 10−10 g/g. Thus, the mass introduction rate of 233U is 3.89 × 10−11 g/min according to EquationEquation (6).
In a base case, saturator and condenser both operated under room temperature thus no temperature difference existed. The dependence of ICP-MS response on dilution argon flow in this case is shown in . The signal intensity increases first with the increase of dilution argon flow, because more samples are introduced into the torch through the interface. But the response decreases when the dilution argon flow is larger than about 12.8 L/min, because the ICP plasma is cooled as too many argon flows are introduced.
When the temperature difference between the saturator and the condenser increases to 21.8°C and 25.0°C, the peak of the response curve shifts left and rises up. Comparing the signal intensities at Q d = 12.3 L/min under dT = 0°C and 25°C, the response is improved by a factor of 2.3 through concentration enrichment and matrix gas removal processes. Moreover, the sampling flow of environmental aerosol increases by 1 order of magnitude through the interface comparing with that without the interface. Thus, the response time for online monitoring of environmental aerosol improves dramatically.
3.5. Monitoring of Uranium Concentration in Environmental Aerosol in the Clean Room
The detectable 238U concentration in environmental aerosol through online monitoring by ICP-MS coupled with the interface was evaluated. The background ion intensity at m/z = 238 (I b) is 2000 cps, while the ion intensity at m/z = 233 (I st) is 8.31 × 104 cps (Q d = 12.3 L/min, dT = 25°C). The measured mass discrimination factor (k) is 1.05. By using EquationEquations (6) and (7), the background equivalent concentration of 238U in the interface plus ICP-MS instrument is calculated to be 1.1 × 10−9 g/m3. The detection ability for uranium concentration in environmental aerosol is several orders of magnitude better than that based on MIP-AES (Duan et al. Citation2000).
Then, offline monitoring of uranium concentration in airborne particles larger than 0.3 μm was conducted. The 208Pb, 232Th, and 238U concentrations in the blank collection liquid in BioSampler before sampling are 2.2 × 10−10 g/g, 1.8 × 10−10 g/g, and 6.3 × 10−10 g/g, respectively. The sampling flow rates of BioSampler 1# and 2# are Q BS,1 = 10.1 L/min and Q BS,2 = 10.4 L/min. The sampling time (t) is 33 min. The collection efficiency is set unity approximately. The determined concentration in the collection liquid after sampling is higher than the blank concentration by a factor of only 1.4–3.9. Following EquationEquation (6), the mass concentrations of these three elements in environmental aerosol larger than 0.3 μm obtained by offline monitoring method are listed in . 238U mass concentration in particles larger than 0.3 μm is on the order of 10−10 g/m3.
TABLE 2 The concentrations of 208Pb, 232Th, and 238U in environmental aerosol larger than 0.3 μm obtained by the offline monitoring method
Size distributions of particles in the range of 10–800 nm in the clean room were scanned subsequently by Scanning Mobility Particles Size (Model 3936L, TSI Inc.). No peak is observed and the total number concentration is as low as that in the filtered air. It indicates that element mass concentration in particles smaller than 0.3 μm is less than that in particles larger than 0.3 μm. Therefore, the elemental mass concentrations are mainly contributed by airborne particles larger than 0.3 μm. They are several orders of magnitude lower than the regulatory value for the purpose of radiation protection [GB 8703–88].
The background equivalent concentration of 238U in ICP-MS coupled with the interface is a little high, so the ultra-trace 238U in the airborne particles in the clean room introduced directly is undetectable. The background mainly comes from the memory effect in the instrument and impurity in the reagent. Wash of the sampling cone in ICP-MS instrument and the membrane dryer in the interface and selection of ultra-pure reagent are necessary to reduce the background.
In Suzuki's work (Suzuki et al. Citation2012), 15 metal elements except uranium were monitored in real-time by direct introduction into ICP-MS coupled with a gas converter. It is reported that the limit of detection and limit of quantification for lead concentration in airborne aerosol are 0.9 × 10−9 g/m3 and 3.0 × 10−9 g/m3. Hence, the detection ability of the equipment developed in this article is comparable. It has some advantages:
1. | The aerosol sampling flow with single interface could reach 1 L/min without the need of parallel use of four gas converters, therefore the construction and operation of the interface are simple and easy-to-use. | ||||
2. | Calibration aerosol is introduced at the inlet of the interface to calibrate the transport efficiency and instrumental sensitivity in total for quantitative monitoring of environmental aerosol. | ||||
3. | Isotope contained in the calibration aerosol is preferred to simulate the analytical properties of element to be measured and to reduce the memory effect and possible contamination for ultratrace monitoring with ICP-MS. |
4. CONCLUSION
An aerosol sample introduction interface coupled with ICP-MS for direct introduction and quantitative online monitoring of environmental aerosol has been developed and characterized. The calibration aerosol is nebulized and desolvated to calibrate the transport efficiency in the interface and instrumental sensitivity in total for quantitative analysis of environmental aerosol.
Online analytical performance of ICP-MS coupled with the interface was evaluated. The results show that the response time for online monitoring of environmental aerosol improves dramatically. The sensitivity is improved through concentration enrichment and matrix gas removal processes. The background equivalent concentration of 238U is 1.1 × 10−9 g/m3. As preliminary comparison study, monitoring of uranium concentration in environmental aerosol in the clean room was conducted. The 238U concentration in environmental aerosol larger than 0.3 μm by conventional offline monitoring method is on the order 10−10 g/m3. Restricted by the background, the ultra-trace 238U could not be detected by the online monitoring method.
In future application, online monitoring of environmental aerosol with 238U concentration in the range of 10−9 to 10−6 g/m3 would be feasible. Furthermore, miniaturized mass spectrometer with combination of smart ion source, i.e., microwave-induced plasma (MIP) (Jin et al. Citation1998), and miniature mass analyzer (Badman and Cooks Citation2000), i.e., time-of-flight-MS and ion trap-MS, is now under study for in situ, real-time monitoring of environmental aerosol.
Acknowledgments
This work was financially supported by National Natural Science Foundation of China (NSFC) under Grant No. 11105107. The authors acknowledge Professor Hai-Jun Dang for his helpful comments on the article.
REFERENCES
- Ahn , K. H. and Liu , B. Y. H. 1990 . Particle Activation and Droplet Growth Processes in Condensation Nucleus Counter-I. Theoretical Background . J. Aerosol Sci. , 21 : 249 – 261 .
- Badman , E. R. and Cooks , R. G. 2000 . Miniature Mass Analyzers . J. Mass Spectrom., , 35 : 659 – 671 .
- Baron, P. A, and Willeke, K. 2001 . Aerosol Measurement: Principles, Techniques and Applications, , 2nd Edition , New York : John Wiley & Sons Inc. .
- Duan , Y.-X. , Su , Y.-X. , Jin , Z. and Abeln , S. P. 2000 . A Field-Portable Plasma Source Monitor for Real-Time Air Particulate Monitoring . Anal. Chem. , 72 : 1672 – 1679 .
- Jin , Q.-H. , Yang , W.-J. Liang , F. 1998 . Recent Advances in Microwave Plasma Torch Atomic Emission Spectrometry . J. Anal. Atom. Spectrom. , 13 : 377 – 384 .
- Kapellois , E. A. and Pergantis , S. A. 2012 . Size and Elemental Composition of Nanoparticles using Ion Mobility Spectrometry with Inductively Coupled Plasma Mass Spectrometry . J. Anal. Atom. Spectrom. , 27 : 21 – 24 .
- Li , Z.-M. , Su , Y.-Y. Ren , X.-J. 2011 . Direct Determination of Ultra-trace Plutonium Nanoparticles in Downstream of a Six-Stage HEPA Filter by Inductively-Coupled-Plasma Mass Spectrometry: A Field Application . Aerosol Sci. Tech. , 45 : 1199 – 1205 .
- Meyer , G. A. and Lee , K. W. 1994 . Real-Time Determination of Metal Hazardous Air Pollutants in Flue Gas Emission: Laboratory Study . Process Control and Quality , 6 : 187 – 194 .
- Myojo , T. , Takaya , M. and Ono-Ogasawara , M. 2002 . DMA as a Gas Converter from Aerosol to “Argonsol” for Real-Time Chemical Analysis using ICP-MS . Aerosol Sci. Tech. , 36 : 76 – 83 .
- Nishiguchi , K. , Utania , K. and Fujimori , E. 2008 . Real-Time Multi-Element Monitoring of Airborne Particulate Matter using ICP-MS Instrument Equipped with Gas Converter Apparatus . J. Anal. Atom. Spectrom. , 23 : 1125 – 1129 .
- Nomizu , T. , Hayashi , H. Hoshino , N. 2002 . Determination of Zinc in Individual Airborne Particles by Inductively Coupled Plasma Mass Spectrometry with Digital Signal Processing . J. Anal. Atom. Spectrom. , 17 : 592 – 595 .
- Nomizu , T. , Kaneco , S. Tanaka , T. 1993 . Determination of Fetogram Amounts of Zinc and Lead in Individual Airborne Particles by Inductively Coupled Plasma Mass Spectrometry with Direct Air-Sample Introduction . Anal. Sci. , 9 : 843 – 846 .
- Seltzer , M. D. and Green , R. B. 1994 . Instrumentation of Continuous Emissions Monitoring of Airborne Metals . Process Control and Quality , 6 : 37 – 46 .
- Su , Y.-Y. , Li , Z.-M. , Dong , H.-B. , Zhou , G.-Q. , Zhai , L.-H. Xu , J. 2011 . Preliminary Study on Aerosol Particle Addition Calibration Method for On-Line Quantitative Analysis of Airborne Radioactive Particles with ICP-MS . Int. J. Environ. Anal. Chem. , 91 ( 5 ) : 473 – 483 .
- Su , Y.-Y. , Li , Z.-M. , Dong , H.-B. , Zhou , G.-Q. , Zhai , L.-H. Xu , J. 2009 . Study of On-Line Chemical Analysis of Aerosol Individual Particles with ICP-MS . J. Ins. Anal. (Fen Xi Ce Shi Xue Bao) , 28 : 436 – 439 . (in Chinese)
- Su , Y.-Y. , Li , Z.-M. Zhou , G.-Q. 2010 . On-Line Quantitative Analysis of Uranium in Individual Particle with ICP-MS using Isotopic Aerosol Particle Addition Calibration Method . Atom. Energy Sci. Technol. , 44 ( 3 ) : 272 – 277 . (in Chinese)
- Suzuki , Y. , Sato , H. Hikida , S. 2010 . Real-Time Monitoring and Determination of Pb in Single Airborne Nanoparticle . J. Anal. Atom. Spectrom. , 25 : 947 – 949 .
- Suzuki , Y. , Sato , H. Hiyoshi , K. 2012 . Quantitative Real-Time Monitoring of Multi-Elements in Airborne Particulates by Direct Introduction into an Inductively Coupled Plasma Mass Spectrometer . Spectrochim. Acta B , 76 : 133 – 139 .
- Willeke , K. , Lin , X.-J. and Grinshpun , S. A. 1998 . Improved Aerosol Collection by Combined Impaction and Centrifugal Motion . Aerosol Sci. Tech. , 28 ( 5 ) : 439 – 456 .
- Zhou , G.-Q. , Zhu , F.-R. , Zhang , Z.-B. , Bai , J.-F. , Jin , Y.-R. Zhang , J.-M. 2002 . Determination of Ultra-Trace Plutonium in Atmospheric Aerosols by Inductively Coupled Plasma Mass Spectrometry . J. Chinese Mass Spectrom. (Zhi Pu Xue Bao) , 23 : 151 – 155 . (in Chinese)