ABSTRACT
Lycopene is a highly valued carotenoid with wide applications in various industries. The market demand for lycopene promotes research in metabolic engineering of heterologous hosts for lycopene. In this study, Pichia pastoris strain GS115 was genetically engineered to produce lycopene by integrating the heterologous lycopene biosynthesis genes from Corynebacterium glutamicum ATCC13032. The resulting strain, L1, produced 0.115 mg/g cell dry weight (DCW) lycopene. Through optimization by promoter selection, improving the precursor supply and expanding the Geranylgeranyl diphosphate (GGPP) pool, ultimately, the lycopene yield of the final optimal strain was 6.146 mg/g DCW with shake flask fermentation and 9.319 mg/g DCW (0.714 g/L) in a 3 L fermenter. The lycopene yield in this study is the highest yield of lycopene in P. pastoris reported to date, which demonstrated the potential of P. pastoris in lycopene synthesis and as a candidate host organism for the synthesis of other high value-added terpenoids.
Graphical abstract
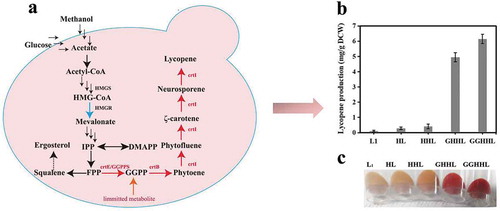
P. pastoris strain GS115 was genetically engineered to produce lycopene and the lycopene yield was improved step by step.
Lycopene (C40H56) is a carotenoid with antioxidative and anticancer activities [Citation1], that is used widely in various markets, such as the nutraceutical and cosmetic industries [Citation2,Citation3]. Currently, lycopene is mainly produced by direct extraction from tomatoes, chemical synthesis, and microbial fermentation [Citation4]. However, the extraction method requires a very long growth cycle. Chemical synthesis involves the use of harmful materials [Citation5]. Therefore, the production of lycopene in microorganisms such as Escherichia coli, Saccharomyces cerevisiae and Pichia pastoris (Komagataella phaffii) [Citation6–Citation8] is considered as a potential alternative.
P. pastoris is a methylotrophic yeast which has the strong respiratory metabolism ability and thus grows to very high cell densities in simple media and can also be easily scaled-up from shake-flasks to large-scale bioreactors [Citation9,Citation10]. Therefore, the combined characteristics of P. pastoris give this strain great potential in producing compounds, such as carotenoids. Recent studies have already proven that P. pastoris is suitable for the production of natural products such as hyaluronic acid, carotenoids, and the sesquiterpenoid (+)-nootkatone [Citation6,Citation11,Citation12]. When the carotenogenic genes derived from Erwinia uredovora were overexpressed with the PGAP promoter, 73.9 mg/L of lycopene was obtained in P. pastoris [Citation13]. However, reports focusing on the production of carotenoids are still deficient. Therefore, heterogeneous carotenoids production in P. pastoris needs to be further studied.
In the present study, a novel source of carotenoid synthetase from Corynebacterium glutamicum ATCC13032 were introduced into the P. pastoris strain GS115 to construct a lycopene producing strain (). The effect of different promoters, PAOX1 and PGAP,on lycopene synthesis was explored and compared (). The results indicated that the inducible promoter PAOX1 may be stronger than PGAP and more suitable for lycopene biosynthesis in P. pastoris. Furthermore, to improve the lycopene production, the endogenous 3-hydroxy-3-methylglutaryl-coenzyme A (HMG-CoA) reductase gene (HMGR) and 3-hydroxy-3-methylglutaryl-CoA synthase gene (HMGS) were overexpressed to modulate the mevalonate pathway. Finally, increasing the number of copies of the endogenous geranylgeranyl diphosphate synthase gene (GGPPS) was used to expand the GGPP pool in a further effort to improve lycopene production.
Figure 1. Overview of the lycopene biosynthesis pathway in P. pastoris.
HMGR: HMG-CoA reductase; HMGS: HMG-CoA synthase; GGPPS (crtE): Geranylgeranyl diphosphate synthase; crtB: Phytoene synthase; crtI: Phytoene desaturase. The heterologous genes crtE, crtI, and crtB are from C. glutamicum ATCC13032; multiple reaction steps were indicated by dashed arrows. The heterogeneous biosynthetic pathways were highlighted with red ellipses.
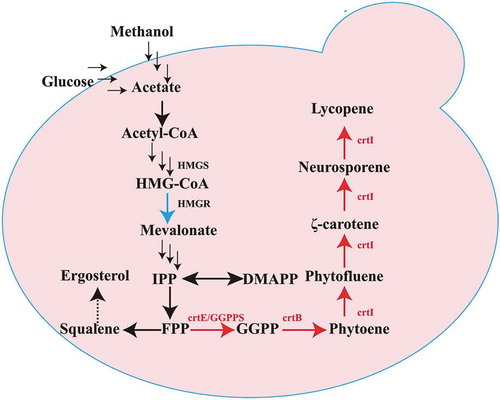
Materials and methods
Strains, vectors, reagents, and culture media
Escherichia coli TOP10F’ (Invitrogen, Carlsbad, CA, USA) was used as the host for DNA manipulations, and the cells were cultivated in 5 mL LB medium or low-salt LB (LBL) medium at 37°C. Zeocin (25 mg/L) or kanamycin (100 mg/L) was used for bacterial plasmid maintenance and selection. The P. pastoris strain GS115 (Invitrogen) was used as the host cell, and was cultivated in YPD medium (yeast extract 1%, peptone 2 %, and glucose 2 %) at 30°C. Transformants of P. pastoris were selected on MD plates (yeast nitrogen base (w/o amino acids) (YNB) 1.34%, biotin 4 × 10−5%, glucose 2%, and agar 2%) or YPDSZ plates (yeast extract 1%, peptone 2%, glucose 2%, sorbitol 18.2%, agar 2%, and 100 mg/L zeocin). Gel-purified linearized plasmids or DNA fragments were transformed into P. pastoris using the electroporation method according to the detailed protocol described by Cregg [Citation14]. The transformed cells were selected on MD plates or YPDSZ plates and incubated at 30°C for 2–3 days. Engineered yeast strains were cultivated in BMGY (yeast extract 1%, peptone 2%, YNB 1.34%, biotin 0.00004%, potassium phosphate (pH 6.0) 100 mM, and glycerol 1%) and BMMY medium (yeast extract 1%, peptone 2%, YNB 1.34%, biotin 0.00004%, potassium phosphate (pH 6.0) 100 mM, and methanol 1%).
Basal salt medium (BSM) (glycerol 4%, K2SO4 1.82%, CaSO4 0.093%, KOH 0.413%, H3PO4 2.67%, MgSO4·7H2O 1.49%, and PTM1 0.435%) and the trace metal solution PTM1 (CoCl2 0.05%, CuSO4·5H2O 0.6%, FeSO4·H2O 6.5 %, H3BO3 0.002%, KI 0.009%, H2SO4 0.5 %, MoNa2O4·2H2O 0.024%, ZnCl2 2%, MnSO4·H2O 0.3%, and biotin 0.02%), were used in fed-batch cultivation (3 L). A glycerol solution 50% (w/v) containing PTM1 1.2% (v/v) and a methanol solution containing PTM1 1.2% (v/v) were used as feed strategies according to the reference protocol in “Pichia Fermentation Process Guidelines” (Invitrogen).
All restriction enzymes, T4 DNA ligase and primers were purchased from Sangon Biotech (Shanghai, China). DNA polymerase which was used for DNA fragments amplified was purchased from Takara Bio (Shiga, Japan). The lycopene standard was purchased from Sigma-Aldrich (St. Louis, MO, USA).
Gene amplification and construction of vectors
To construct the lycopene pathway, the crtE, crtI and crtB genes were amplified from genomic DNA of C. glutamicum ATCC13032. The genes HMGR, HMGS, and GGPPS were amplified from the GS115 genome. For pathway assembly, the constructed plasmid with crtE, crtI and crtB was subsequently inserted into the yeast genome to build a lycopene synthesis pathway. Details of the recombinant plasmids are shown in . The yeast cells carrying plasmids with HIS4 or zeocin selectable markers were selected on MD plates, or YPDSZ plates, respectively.
Table 1. Plasmids used in this study.
Construction of lycopene producing yeast strains
P. pastoris GS115 was used as the host for plasmids integration and lycopene biosynthetic pathway introduction. First, the carotenoid genes (crtE, crtB and crtI) with different promoters were integrated into the yeast chromosomes. Subsequently, HMGR, HMGS, and GGPPS were further integrated into the genome of recombinant yeast L1. The Cre/loxP ZeoR marker recycling vector [Citation15] was used to generate the recombinant strain. The strain construction process is summarized in . All the strains used in this study are summarized in .
Table 2. Yeast strains used in this study.
Shaker flask cultivations
The engineered yeast cells were first inoculated into 10 mL of BMGY medium in a 50 mL Erlenmeyer flask. The cells were grown overnight at 30°C with shaking at 250 rpm. Then, the main cultures were inoculated into a 250 mL Erlenmeyer flask, containing 25 mL of BMMY medium (yeast extract 1%, peptone 2%, YNB 1.34%, biotin 0.00004%, potassium phosphate (pH 6.0) 100 mM, and methanol 1%) with an initial OD600 of 1 and cultured in a shaking incubator under the same conditions for 120 h. 1% (v/v) fresh methanol was added each every 24 h.
Extraction and analysis of carotenoids
To extract carotenoids, 700 mg of 0.5 mm silica beads (BioSpec Products (Bartlesville, OK, USA)) and 1 ml of cold (4°C) organic solvent mixture containing hexane and acetone (3:2 v/v) were added into individual 2 mL screw cap tube containing each lyophilized biomass. The suspensions were subjected to breaking for 30 sec using the cell breakage apparatus sample preparation system. Broken suspensions were centrifuged at 10,000 rpm for 10 min, at 4°C, and supernatants were transferred to 2 mL Eppendorf tubes. The supernatants were evaporated to dryness at 40°C by applying a constant air flow under the fume hood. The extraction cycle described above was performed three to four times until all color was removed from the cracked cells. Carotenoid residues were redissolved in a cold (4°C) organic solvent mixture containing hexane and acetone (3:2 v/v) by vortexing. A volume of 200 μL of the supernatants was transferred to brown 2 mL screw cap glass vials (Agilent Technologies, Palo Alto, CA, USA) and was either stored in the dark at −20°C or no longer than two days or directly subjected to HPLC analysis.
The quantification of lycopene was performed on a Waters 2695 series HPLC system (Waters Technologies, Milford, MA, USA) equipped with Waters C18 column (4.6 mm × 250 mm) and UV/VIS detector detected signals at 450 nm. For separation, samples were eluted with an isocratic elution program acetonitrile-isopropanol-methanol (50:20:30 v/v) at a flow rate of 1 mL/min at 40°C. The injection volume was set to 10 μL.
Fed-batch fermentation of the yeast strains
To create the seed culture, several single colonies were firstly inoculated into 250 mL Erlenmeyer flasks containing 50 mL YPD medium shaking flask at 30°C (250 rpm) for about 20 hours, after which 4% of the seed culture was transferred to a 500 mL Erlenmeyer flask containing 100 mL YPD medium and cultured under the same conditions for about 20 h. 8% of the seed culture was then inoculated into BSM medium in a 3 L fermenter for fed-batch fermentation.
The high-density fermentation of the recombinant P. pastoris strain was performed in a 3 L fed-batch fermentation (FUS10-A, Shanghai Guoqiang Bioengineering Equipment, Shanghai, China) containing 1.2 L of BSM. The parameters of the culture fermenter were as follows: growth at 30°C, air flow rate of 10 L/min, growth at pH 5.5 (the pH was adjusted to 5.5 by adding ammonia solution (25%, v/v)) and stirring speed of 800 rpm. The dissolved oxygen (DO) was maintained at approximately 20–30%. Typical recombinant P. pastoris batch fermentation was composed three phases. The batch phase (phase I) was in BSM for initial cell growth; this phase lasted for approximately 18–24 h at pH 5.5 (the pH was adjusted to 5.5 by adding ammonia solution (25%, v/v)) and 30°C. After the glycerol was exhausted, phase II (fed-batch phase) was started to allow further cell growth by feeding limited glycerol. When the cell OD600 reached approximately 230, there was a carbon source starvation period of 30 min, and glycerol feeding was stopped to allow complete consumption of the glycerol. At the same time, the temperature was 25°C, and the pH was adjusted to 6.0 by adding ammonia solution (25%, v/v). The induction phase (phase III) was initiated by the addition of methanol as the carbon source (10–15 g/h). The dissolved oxygen (DO) was maintained at approximately 20–30% constantly, growth at 30°C, air flow rate of 10 L/min, stirring speed of 800 rpm and the pH was adjusted to 6.0 by adding ammonia solution (25%, v/v). OD600 and lycopene productivity were monitored throughout a 6-day induction.
Results and discussion
Construction of the lycopene synthetic pathway in P. pastoris
P. pastoris does not naturally produce lycopene. The yeast strain GS115 was engineered to confer a novel heterogeneous biosynthetic pathway for the production of lycopene. To construct the lycopene synthetic pathway in P. pastoris, we attempted to integrate the crt genes from a new source of C. glutamicum ATCC13032 into the yeast chromosome using the linearized PHKA-crtE-crtB-crtI and PGAP-crtE-crtB-crtI vectors with the promoters of PAOX1 and PGAP, respectively. The resulting strains were PAOX1/L (L1) and PGAP/L (). Promoter AOX1 (PAOX1) is a strong and tightly regulated promoter, while promoter GAP (PGAP) is constitutive promoter. According to “Pichia pastoris expression Guidelines”, methanol as a carbon source (BMMY medium) was usually used to cultivate P. pastoris transformants with the expression vector whose promoter is AOX1 (PAOX1) and P. pastoris transformants with the expression vector whose promoter is GAP (PGAP) were usually cultivated in medium with glucose as a carbon source. For shake flask fermentation, PAOX1/L (L1) and PGAP/L were cultivated in BMGY medium with an initial OD600 of 1; fresh methanol (1% v/v) and glucose as carbon sources were added every 24 h, respectively. The results of the HPLC analysis showed that 0.115 mg/g DCW of lycopene was accumulated when the crtE, crtI and crtB genes from C. glutamicum ATCC13032 were expressed in GS115 using the promoter PAOX1, which was superior to PGAP (0.045 mg/g DCW) ()), while the cell growth of the strain PGAP/L is better ()). The results indicated that heterologous biosynthesis of lycopene as a metabolic product could be achieved in engineered P. pastoris using the lycopene synthesis genes from C. glutamicum ATCC13032 and that the inducible promoter PAOX1 may be more suitable for lycopene biosynthesis in P. pastoris. In previous studies, when the E. uredovora crt genes were used to construct lycopene biosynthesis pathways in P. pastoris with the PGAP promoter, only 73.9 mg/L of lycopene in minimal medium and 4.6 mg/g DCW of lycopene in batch fermentation were obtained [Citation13]. The yield of lycopene was so low, and there are few researches for the optimization of lycopene synthesis in P. pastoris. Thus, optimization of lycopene synthesis in P. pastoris needs to be further studied.
Figure 3. Cell growth and lycopene contents of engineered P. pastoris strains.
(a) The cell dry weight of the PAOX1/L and PGAP/L strains cultured in BMMY medium, measured at 0, 24, 48, 72, 96 and 120 h. (b) The lycopene contents of the PAOX1/L and PGAP/L strains cultured in BMMY for 5 days with 1% methanol addition and 1% glucose addition every 24 h, respectively.
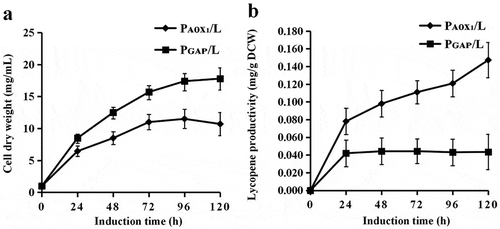
Engineering the MVA pathway for lycopene production in P. pastoris
The mevalonate (MVA) pathway provides an effective supply for the synthesis of terpenoids and can improve the target product yield [Citation16]. For the efficient synthesis of target products, increasing the supply of the MVA pathway in P. pastoris was expected to be an efficient way to enhance the yield of lycopene. The overexpression of endogenous HMG1 or truncated HMG1 (tHMG1),the key enzyme gene in the MVA pathway, could increase the supply of the FPP precursor for terpenoid biosynthesis [Citation17–Citation19] in S. cerevisiae. Therefore, the Pichia pastoris endogenous gene HMGR was overexpressed to enhance metabolic flux from the MVA pathway, resulting in the strain HL. The yield of lycopene in engineering yeast was determined by HPLC after 120 h of shake-flask fermentation. As a result, 0.288 mg/g DCW of lycopene was produced, which was 2.5 times higher than that of strain L1 ()). This result was similar to the synthesis of carotenoids in S. cerevisiae [Citation20].
Figure 4. Production of lycopene by engineered P. pastoris strains.
(a) Analysis of lycopene production of engineered P. pastoris strains, including L1, HL, HHL, GHHL and GGHHL. Strains were cultured in BMMY for 5 days with 1% methanol addition every 24 h . (b) The comparison of the lycopene production of different strains.
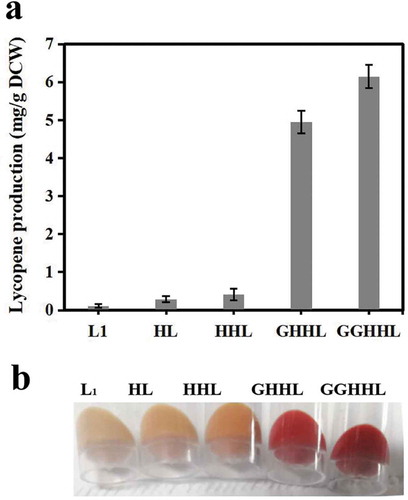
Subsequently, to further enhance the flux of the MVA pathway for enhancement lycopene biosynthesis, we attempted to overexpress endogenous 3-hydroxy-3-methylglutaryl-CoA synthase (HMGS) on the basis of the recombinant strain HL. The resulting strain HHL yielded 0.410 mg/g DCW of lycopene. The lycopene yield increased 3.5-fold after comprehensive modulation of the expression of the endogenous genes HMGR and HMGS ()). These results indicate that the optimization of the MVA pathway increased metabolic flux of precursors effectively and ultimately improved the ability of P. pastoris to synthesize lycopene.
Expanding the GGPP pool for improving lycopene production
GGPP is a key rate-limiting factor for lycopene synthesis in yeast [Citation21]. With a purpose to improve the pool of GGPP, IDI1 from E. coli was overexpressed to increase the flux of FPP in E. coli, which is the immediate precursor of GGPP. And the overexpression of GGPPS could also enhance the GGPP pool in yeast [Citation22]. The endogenous gene GGPPS of GS115 was overexpressed in the stain HHL to enhance the conversion of FPP flux to GGPP, generating the strain GHHL. After shake-flask fermentation for 120 h, the production of lycopene achieved approximately 4.949 mg/g DCW(GHHL), which was improved by 40-fold compared to that of the initial strain L1 ()). By increasing the gene copy numbers of GGPPS (GGHHL), the lycopene yield improved sharply. This result indicated that the GGPPS was a rate-limiting gene for lycopene synthesis. To further improve lycopene production, increasing the gene copy numbers of GGPPS might be helpful for lycopene synthesis. Subsequently, the vector carrying two copies of GGPPS was integrated into the strain HHL ()) to improve the lycopene yield. The lycopene yield of the generating strain GGHHL was 6.146 mg/g DCW, which was 50-fold, 24.2% higher than those of strain L1 and GHHL, respectively. These results showed that the increase of GGPP pool could significantly increase the yield of lycopene.
Effect of gene copy numbers of the crt gene on the production of lycopene
To further increase lycopene production, optimizing the ratios of the crt genes might be helpful for improving the yield of lycopene. For this purpose, a series of vectors pZACH-crtE, pZACH-crtB, pZACH-crtI carrying crtE, crtB and crtI were constructed. These vectors were introduced into the lycopene producing strain GGHHL, respectively. However, no linear correlation between lycopene productivity and copy number was found after increasing the copy number of crtE, crtB and crtI. The lycopene level in the transformed cells did not significantly increase as expected (Supplementary Figure S3). Thus, it is almost certain that the rate-limiting step in the entire lycopene production pathway may be the supply of GGPP in carotenogenic P. pastoris.
Fed-batch fermentation of the strains L1 and GGHHL for lycopene production
The lycopene-producing capacity of strains L1 and GGHHL was evaluated further by high-density fermentation in a 3-L fermenter at pH 5.5. Two stages of cell growth and product accumulation were used during high-density fed-batch fermentation. The prepared liquid seeds were inoculated into the bioreactor, and the induction phase was started with methanol when the biomass (OD600) of the strain reached 230. During the second induction stage with methanol, although the biomass of the strain increased slightly, the accumulation of lycopene increased significantly. After the induction for approximately 136 h, the strain GGHHL produced a lycopene yield of 9.319 mg/g DCW (0.714 g lycopene/L) until the completion of fermentation ()), while the lycopene yield of the original strain L1 was 0.192 mg/g DCW. Although the lycopene content of the strain GGHHL in this work (0.714 g/L) was still lower than that in the S. cerevisiae production system (2.37 g/L) [Citation20], it was much higher than previous reports on lycopene production from engineered P. pastoris strains [Citation13], or the natural producers such as B. trispora and tomato [Citation23].
Conclusion
A lycopene-producing P. pastoris strain was constructed by applying crt genes from C. glutamicum ATCC13032. Lycopene synthesis in P. pastoris were optimized from promoter choice, precursor supply, expanding the GGPP pool and varying the copy number the crt genes. The lycopene yield was improved step by step and finally was up to 9.319 mg/g DCW (0.714 g lycopene/L) approximately 50-fold improvement compared to that of the initial strain L1 (0.192 mg/g DCW), which is the highest lycopene content in P. pastoris reported to date. This work demonstrates the potential of P. pastoris in lycopene synthesis, providing a good example for microbial overproduction of other natural terpenoids in P. pastoris through adaptive optimization of the heterologous pathway.
Author contribution
Shuli Liang conceived the study and designed the experiments. Xinying Zhang, Denggang Wang and Yehong Duan performed the experiments and analysed the data. Xinying Zhang, Xueyun Zheng, Ying Lin and Shuli Liang discussed the results and contributed to improvement of the manuscript. Xinying Zhang wrote the manuscript.
20191110supplementary_materials.docx
Download MS Word (301 KB)Disclosure statement
No potential conflict of interest was reported by the authors.
Supplementary material
Supplemental data for this article can be accessed here.
Additional information
Funding
References
- Grether-Beck S, Marini A, Jaenicke T, et al. Molecular evidence that oral supplementation with lycopene or lutein protects human skin against ultraviolet radiation: results from a double-blinded, placebo-controlled, crossover study. Br J Dermatol. 2017 May;176(5):1231–1240.
- Lee PC, Momen AZ, Mijts BN, et al. Biosynthesis of structurally novel carotenoids in Escherichia coli. Chem Biol. 2003;10(5):453–462.
- Sies H, Stahl W. Lycopene: antioxidant and biological effects and its bioavailability in the human. Pro Soc Exp Biol Med. 1998;218(2):121–124.
- Sun T, Miao L, Li Q, et al. Production of lycopene by metabolically-engineered Escherichia coli. Biotechnol Lett. 2014 Jul;36(7):1515–1522.
- Levesque F, Seeberger PH. Continuous-flow synthesis of the anti-malaria drug artemisinin. Angew Chem. 2012 Feb 13;51(7):1706–1709.
- Araya-Garay JM, Feijoo-Siota L, Rosa-dos-Santos F, et al. Construction of new Pichia pastoris X-33 strains for production of lycopene and beta-carotene. Appl Microbiol Biotechnol. 2012 Mar;93(6):2483–2492.
- Xie W, Lv X, Ye L, et al. Construction of lycopene-overproducing Saccharomyces cerevisiae by combining directed evolution and metabolic engineering. Metab Eng. 2015 July 1;30:69–78.
- Zhu F, Lu L, Fu S, et al. Targeted engineering and scale up of lycopene overproduction in Escherichia coli. Process Biochem. 2015;50(3):341–346.
- Macauley-Patrick S, Fazenda ML, McNeil B, et al. Heterologous protein production using the Pichia pastoris expression system. Yeast. 2005 Mar;22(4):249–270.
- Ramon A, Marin M. Advances in the production of membrane proteins in Pichia pastoris. Biotechnol J. 2011 Jun;6(6):700–706.
- Wriessnegger T, Augustin P, Engleder M, et al. Production of the sesquiterpenoid (+)-nootkatone by metabolic engineering of Pichia pastoris. Metab Eng. 2014 Jul;24:18–29.
- Jeong E, Shim WY, Kim JH. Metabolic engineering of Pichia pastoris for production of hyaluronic acid with high molecular weight. J Biotechnol. 2014 Sep;20(185):28–36.
- Bhataya A, Schmidt-Dannert C, Lee PC. Metabolic engineering of Pichia pastoris X-33 for lycopene production. Process Biochem. 2009;44(10):1095–1102.
- Cregg JM, Tolstorukov I, Kusari A, et al. Expression in the yeast pichia pastoris. Methods Enzymol. 2009;463:169-189.
- Li C, Lin Y, Zheng X, et al. Recycling of a selectable marker with a self-excisable plasmid in Pichia pastoris. Sci Rep. 2017 Sep 11;7(1):11113.
- Li X, Wang Z, Zhang G, et al. Improving lycopene production in Saccharomyces cerevisiae through optimizing pathway and chassis metabolism. Chem Eng Sci. 2019;193:364–369.
- Misawa N. Pathway engineering for functional isoprenoids. Curr Opin Biotechnol. 2011 Oct;22(5):627–633.
- Paradise EM, Kirby J, Chan R, et al. Redirection of flux through the FPP branch-point in Saccharomyces cerevisiae by down-regulating squalene synthase. Biotechnol Bioeng. 2008 Jun 1;100(2):371–378.
- Rico J, Pardo E, Orejas M. Enhanced production of a plant monoterpene by overexpression of the 3-hydroxy-3-methylglutaryl coenzyme A reductase catalytic domain in Saccharomyces cerevisiae. Appl Environ Microbiol. 2010 Oct;76(19):6449–6454.
- Ma T, Shi B, Ye Z, et al. Lipid engineering combined with systematic metabolic engineering of Saccharomyces cerevisiae for high-yield production of lycopene. Metab Eng. 2019 Mar;52:134–142.
- Verwaal R, Jiang Y, Wang J, et al. Heterologous carotenoid production in Saccharomyces cerevisiae induces the pleiotropic drug resistance stress response. Yeast. 2010 Dec;27(12):983–998.
- Song MC, Kim EJ, Kim E, et al. Microbial biosynthesis of medicinally important plant secondary metabolites. Nat Prod Rep. 2014 Nov;31(11):1497–1509.
- Mantzouridou F, Tsimidou MZ. Lycopene formation in Blakeslea trispora chemical aspects of a bioprocess. Trends Food Sci Technol. 2008;19(7):363–371.