ABSTRACT
While the model bacteria Escherichia coli and Bacillus subtilis harbor single chromosomes, which is known as monoploidy, some freshwater cyanobacteria contain multiple chromosome copies per cell throughout their cell cycle, which is known as polyploidy. In the model cyanobacteria Synechococcus elongatus PCC 7942 and Synechocystis sp. PCC 6803, chromosome copy number (ploidy) is regulated in response to growth phase and environmental factors. In S. elongatus 7942, chromosome replication is asynchronous both among cells and chromosomes. Comparative analysis of S. elongatus 7942 and S. sp. 6803 revealed a variety of DNA replication mechanisms. In this review, the current knowledge of ploidy and DNA replication mechanisms in cyanobacteria is summarized together with information on the features common with plant chloroplasts. It is worth noting that the occurrence of polyploidy and its regulation are correlated with certain cyanobacterial lifestyles and are shared between some cyanobacteria and chloroplasts.
Abbreviations
NGS: next-generation sequencing; Repli-seq: replication sequencing; BrdU: 5-bromo-2′-deoxyuridine; TK: thymidine kinase; GCSI: GC skew index; PET: photosynthetic electron transport; RET: respiration electron transport; Cyt b6f complex: cytochrome b6f complex; PQ: plastoquinone; PC: plastocyanin.
GRAPHICAL ABSTRACT
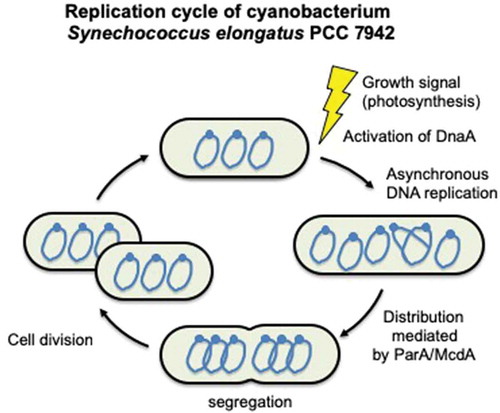
Replication cycle of the cyanobacterium Synechococcus elongatus PCC 7942.
Cyanobacteria are the predominant phototrophs in ocean and freshwater ecosystems and are one of the most widespread phylogenetic clades. Cyanobacteria have oxygen-producing photosynthetic capabilities, meaning that they can produce biomass by using solar energy, and they have recently gained attention for their potential as green cell factories for CO2-neutral biosynthesis of various products. In particular, there is increasing interest in the production of biofuels and valuable chemicals using cyanobacteria [Citation1–Citation3]. Numerous aspects of cyanobacterial genetics and physiology have been intensively studied in recent years.
Some marine picocyanobacteria, such as the clade that includes Synechococcus sp. and Prochlorococcus sp., are monoploid [Citation4,Citation5] like Escherichia coli and Bacillus subtilis, whereas several freshwater cyanobacteria are polyploid, meaning that they harbor multiple chromosome copies per cell [Citation4,Citation6,Citation7]. Similar polyploidy has been observed in some bacterial species, such as Deinococcus radiodurans [Citation8], Thermus thermophilus [Citation9], and in other symbiotic and parasitic bacteria [Citation10–Citation12], as well as in plant chloroplasts, which are evolutionarily derived from cyanobacteria [Citation13,Citation14]. Polyploidy has also been reported in several archaeal lineages [Citation15–Citation18].
The freshwater cyanobacteria Synechococcus elongatus PCC 7942 and Synechocystis sp. PCC 6803 (hereafter referred to as S. elongatus 7942 and S. sp. 6803, respectively) are frequently used as model phototrophs because their transformation efficiencies and growth rates are superior to those of other cyanobacteria. It has been reported that S. elongatus 7942 carries 3–4 chromosome copies () [Citation19–Citation21], whereas S. sp. 6803 contains more than 10 chromosome copies per cell during exponential growth phase [Citation22–Citation25]. Recently, Trichodesmium sp. was reported to be highly polyploid, containing as many as 100 chromosome copies per cell in the field, and 600 chromosome copies under laboratory conditions [Citation26]. The chromosome copy numbers of other cyanobacteria were reported by Griese et al. [Citation21]. Although an unusual chromosome copy number was reported for S. sp. 6803 in this report, a correction was made in a subsequent article by the same group [Citation23].
A plausible evolutionary advantage of polyploidy is that the replicated chromosomes can compensate for damage to the other chromosomes. In cyanobacteria and chloroplasts, this chromosomal damage is highly likely because their DNA is always exposed to the oxidative stress that results from photosynthesis [Citation27]. Another explanation for polyploidy is its correlation with cell volume, as chromosome copy number is proportional to cell size. Trichodesmium sp., which harbors 100–600 chromosome copies per cell, is >3 orders of magnitude larger by volume than monoploid picocyanobacteria, such as Prochlorococcus sp. and Synechococcus sp [Citation26,Citation28]. In addition, recent studies of S. elongatus 7942 revealed that the number of replicating chromosomes is restricted to maintain a stable gene copy number/cell volume ratio during growth [Citation29,Citation30].
In this review, the available knowledge of ploidy and the mechanisms of DNA replication in cyanobacteria, specifically S. elongatus 7942 and S. sp. 6803, is summarized together with information about their common features with chloroplasts. Notably, the occurrence of polyploidy and its regulation are correlated with certain cyanobacterial lifestyles and are shared between some cyanobacteria and plant chloroplasts.
Polyploidy changes
The degree of polyploidy in cyanobacteria is very flexible, and it depends on the growth conditions. In batch culture, cyanobacterial growth progresses from lag phase to exponential phase. This is typically followed by a period of linear growth that continues until the culture reaches a non-growing stationary phase. The degree of ploidy is significantly higher during lag phase than during the exponential and linear phases (). Intensive DNA replication and increased ploidy have been observed during lag phase in both S. elongatus 7942 and S. sp. 6803. During lag phase, the chromosome copy number increases to 4–10 in S. elongatus 7942 [Citation19,Citation20] and to 10–20 in S. sp. 6803 [Citation23,Citation24,Citation25]. This increase is necessary for the subsequent cell division and elongation steps that occur during the exponential and linear growth phases [Citation19].
In contrast, polyploidy is minimized during linear growth phase, which is associated with nutrient limitation in the medium as well as light limitation caused by cell self-shading [Citation31–Citation33]. In batch cultures of S. elongatus 7942 in BG11 medium, the phosphate is completely depleted at the start of linear growth phase (). During linear growth phase, low-level growth was observed, even in the absence of an extracellular phosphate source along with a concomitant reduction in chromosome copy number to 2–3 copies per cell, suggesting that DNA can be used as a source of organic phosphate to support further growth () [Citation19]. A similar phenomenon has been reported in S. sp. 6803. The chromosome number gradually decreased over time, and after 16 d of cultivation, the cells became monoploid [Citation23]. This reduction in chromosome copy number during linear growth phase was suppressed by surplus phosphate [Citation23]. Such ploidy changes are related to maintenance of the balance between the frequency of DNA replication and cell division, which is influenced by growth phase and environmental conditions and seems to be a common mechanism among polyploid organisms. In fact, such polyploidy changes have also been observed in haloarchaea [Citation34,Citation35].
S. elongatus 7942 has been widely used as a model for studying prokaryotic circadian rhythms [Citation36]. Although cell division is controlled by the circadian rhythm [Citation37–Citation39], ploidy is stable even when the circadian rhythm of S. elongatus 7942 cells is synchronized [Citation6], indicating that chromosome copy number regulation is independent of the circadian rhythm in this species. Under circadian rhythm-synchronized culture conditions, it is thought that only one or two chromosomes in the cell replicates in a light-dependent manner. Therefore, ploidy does not significantly change.
Cyanobacteria share certain characteristics related to ploidy changes with chloroplasts, which evolved from a freshwater lineage of cyanobacteria [Citation40]. It has been reported that DNA replication in chloroplasts is not coupled to nuclear DNA replication or chloroplast division [Citation41–Citation43]. In green plants, such as wheat, barley, and pea, the chromosome number in chloroplasts varies according to the developmental stage [Citation41,Citation42,Citation44–Citation46]. Proplastids, which are undifferentiated chloroplasts, undergo intensive DNA amplification before cell division, which is a fundamental step toward subsequent proplastid division. In contrast, during leaf development and plant growth, the DNA content in chloroplasts is reduced to very low levels [Citation47]. Mutants of chloroplast-specific DNA polymerase and RecA exhibited delays in plant growth and development and a reduction in chloroplast DNA content [Citation48,Citation49], suggesting that these proteins are important for chloroplast DNA replication. However, the mechanisms that control the chloroplast DNA copy number are still unclear.
Intracellular distribution of the multiple chromosome copies and their regulation
The distribution of the multi-copy chromosomes in S. elongatus 7942 cells has been investigated by microscopy. The replication origin (oriC) and a site opposite of oriC (the putative terC) in the chromosome were labeled, and their intracellular distributions were assayed. The results of these assays indicated that these regions align along the major axis of the cell and that chromosome number is correlated with cell length () [Citation50–Citation51]. Polyphosphate granules, which are a high-energy phosphate source, and carboxysomes, which are metabolic protein microcompartments where carbon fixation occurs, were observed to behave in a similar manner [Citation51,Citation52].
The distribution of the multi-copy chromosomes is regulated by ParA family proteins, which are chromosome-encoded and participate in chromosomal segregation in several bacteria, including B. subtilis [Citation53,Citation54], Pseudomonas aeruginosa [Citation55], and Vibrio cholerae [Citation56,Citation57]. In S. elongatus 7942, ParA (recently named McdA) was shown to regulate the subcellular position of both carboxysomes and multi-copy chromosomes. ParA exhibits DNA binding activity and repeatedly moves from one end of the cell to the other under the control of McdB protein binding [Citation58–Citation60]. Deletion of ParA specifically disrupts the order of both carboxysomes and chromosomes [Citation50,Citation58]. Since disruption of ParA affects both carboxysomes and multi-copy chromosomes in S. elongatus 7942, these findings indicate that ParA is involved in cellular compartmentalization (). In addition, a parA deletion mutant was shown to be more sensitive to UV-C than the wild-type strain. It has also been shown that ParA interacts with SMC_N family proteins, which are highly conserved among cyanobacteria [Citation50], and a SMC_N homologue is involved in chromosome compaction and segregation [Citation61]. Therefore, like ParA, SMC_N family proteins may be involved in chromosome distribution and the UV stress response in S. elongatus 7942.
Genome structure
The genome sequences of several cyanobacteria are unique in terms of their GC skew, as indicated by plots of the normalized guanine (G) over cytosine (C) content in a subgenomic region with sliding windows along the entire genome [Citation62]. In many monoploid organisms, including E. coli and B. subtilis, the GC skew plot divides the genome into a region two regions, one with an excess of G over C and one with an excess of C over G (the leading and lagging strands, respectively). In genomes with this symmetrical GC skew, the shift points of the GC skew plot are correlated with the replication loci oriC and terC () [Citation63]. Thus, such plots have enabled the prediction of the locations of oriC and terC in the chromosomes of monoploid bacteria, including the picocyanobacteria Synechococcus sp. WH 8102 and Prochlorococcus marinus str. CCMP1375. In these monoploid bacteria, the location of oriC has been predicted with high precision using both GC skew and the binding sequence of DnaA, the initiator of DNA replication in bacteria [Citation64].
The GC skews of polyploid organisms, such as S. elongatus 7942, S. sp. 6803, Gloeobacter violaceus PCC 7421, and D. radiodurans, are distinct from those of monoploid bacteria (). The GC skew in polyploid organisms is asymmetrical and contains many high-G/high-C shift points. A similar pattern was also observed in the chloroplasts of Arabidopsis thaliana (). The strength of the GC skew in a given bacterial chromosome can be calculated mathematically as the GC skew index (GCSI), which is useful for estimating the confidence levels for the prediction of oriC and terC based on GC skew [Citation65–Citation67] and are easily obtained from G-language genome analysis [Citation68]. The GCSI scores of the genomes in monoploid organisms, including picocyanobacteria, are high, suggesting clear GC skews, while the GCSI scores of polyploid organisms are generally lower (). A high GCSI score suggests a strong mutation or selection pressure induced by bi-directional replication machinery starting from a single origin [Citation65–Citation67]. Picocyanobacteria are likely to be susceptible to mutation or selection pressure due to their low chromosome copy number, whereas polyploid cyanobacteria are likely to be comparatively less susceptible. It has been suggested that a low GCSI score can also result from long doubling times [Citation66]. However, in cyanobacteria, a low GCSI score seems to be related to ploidy rather than doubling time, because an inverse correlation between GCSI score and ploidy was observed in cyanobacteria (). However, further study is needed to determine the relationship between genome structure and ploidy.
Replication origin (oriC)
In S. elongatus 7942, oriC and the mechanism of replication have been experimentally identified by replication-sequencing (Repli-seq) using next-generation sequencing (NGS) technology [Citation20]. Since S. elongatus 7942 lacks the thymidine kinase (TK) gene, which is necessary for the incorporation of 5-bromo-2′-deoxyuridine (BrdU, a thymidine analog) in DNA, a TK gene was introduced into S. elongatus 7942. The resulting TK-introduced strain was used for Repli-seq analysis and evaluation of DNA replication activity. BrdU-labeled S. elongatus 7942 DNA was purified and quantitatively analyzed by NGS. A clear peak, indicating BrdU-labeled DNA, was observed upstream of the dnaN gene, which contains the dnaA-box cluster (the binding site of the replication initiator DnaA) [Citation69], on the border between the high- and low-GC regions in the GC skew analysis (). The peak broadened as the BrdU labeling time increased, indicating bi-directional DNA replication in S. elongatus 7942, similar to that in monoploid organisms such as E. coli and B. subtilis.
The oriC-like region in the filamentous cyanobacterium Anabaena sp. PCC 7120 has also been shown to be located in between the dnaA and dnaN genes [Citation70]. The oriC-like region of A. sp. 7120 supported autonomous plasmid replication in S. sp. 6803, indicating that S. sp. 6803 has the ability to utilize the identified oriC-like sequence. Notably, the integration of additional oriC-like regions into the chromosome of A. sp. 7120 led to the appearance of multiple, contiguous proheterocysts in the absence of nitrogen, suggesting the involvement of DNA replication in heterocyst development in A. sp. 7120 [Citation70].
Unlike in S. elongatus 7942, which has a genome with a relatively clear GC skew compared to other cyanobacteria, in S. sp. 6803, the replication origin could not be identified [Citation71]. No clear phenotypes, including growth, morphology, and DNA replication activity, were observed in S. sp. 6803 strains lacking the predicted oriC region or dnaA gene. In a Repli-seq analysis of S. sp. 6803, there were no peaks observed in either the predicted oriC region or any other genomic regions, suggesting that there are multiple replication origins that fire asynchronously in S. sp. 6803. This species possesses a genome with strand asymmetry; thus, the replication origin cannot be predicted from GC skew alone, as there are many shift points (). This genomic composition suggests the existence of multiple replication origins in some prokaryotes, similar to that in eukaryotic nuclear chromosomes [Citation72,Citation73]. Therefore, further studies are needed to identify the replication origins in S. sp. 6803.
The mechanism by which chloroplast DNA is replicated is also still a mystery. It appears that chloroplast DNA can be replicated by more than one mechanism [Citation74], including recombination-dependent replication [Citation47,Citation48,Citation75], a double D-loop mechanism [Citation76,Citation77], and rolling circle replication [Citation78]. Studying DNA replication in polyploid cyanobacteria will provide useful information for understanding the mechanism of chloroplast DNA replication.
Asynchronous DNA replication
Analyses based on NGS and microscopy have revealed asynchronous DNA replication in S. elongatus 7942. In rapidly growing bacterial cultures, e.g., E. coli and B. subtilis, DNA replication occurs in almost all cells, and consequently, these bacteria often harbor two or more copies of oriC and one copy of terC [Citation79,Citation80]. Whole-genome NGS showed that the copy number of oriC is significantly greater than that of terC in bacterial cells with multiple replication forks [Citation81,Citation82]. However, in S. elongatus 7942, there is little difference in copy numbers of oriC and terC, even at the peak of DNA replication activity, indicating that the multi-copy chromosomes in this species do not replicate at the same time [Citation20]. Consistent with this observation, immunofluorescence microscopy using BrdU revealed that S. elongatus 7942 cells harbored one or two BrdU foci, indicating a replicated chromosome, even though its multi-copy chromosomes are widely distributed in the cell () [Citation20]. A similar observation was made in S. sp. 6803 [Citation29], suggesting that asynchronous DNA replication is a common feature of polyploid cyanobacteria.
Live-cell imaging has made it possible to visualize the movement of multi-copy chromosomes in S. elongatus 7942 [Citation83,Citation84]. Studies have shown that the distribution of multi-copy chromosomes is correlated with cell division, and multi-copy chromosomes replicate asynchronously in a linear-like fashion according to cell length. There is no specific spatial location where multi-copy chromosomes are replicated in the cell [Citation20,Citation83,Citation84], whereas in monoploid organisms such as E. coli, chromosome replication is preferentially localized either at the center or the quartile points of the major axis [Citation85,Citation86]. After DNA replication, chromosome segregation into daughter cells in S. elongatus 7942 occurs in a seemingly nonrandom fashion, which may be the result of a cellular process that transiently organizes the chromosomes just before the completion of cell division.
Similar observations of DNA replication and chromosomal segregation have been reported in S. sp. 6803 [Citation29,Citation87], which lends further support to the notion that asynchronous DNA replication is a common feature of polyploid cyanobacteria.
The replication initiator protein DnaA
DNA replication is the most fundamental process in the cell cycle of all organisms. The main factors involved in DNA replication are highly conserved among cyanobacteria, and most of them are essential in S. elongatus 7942 () [Citation88]. In model bacteria, such as E. coli, DNA replication is initiated by the binding of DnaA to oriC, which is followed by the recruitment of the components of the replisome [Citation89,Citation90]. The formation of an appropriate DnaA–oriC complex is a critical step in replication control that occurs only once per cell division cycle. Dysfunction of DnaA regulation in E. coli leads to asynchronous DNA replication initiation and polyploidy [Citation91–Citation94], suggesting that regulation of the initiation of DNA replication is important for determining chromosome copy number. Like in most bacteria, DnaA is essential in S. elongatus 7942 [Citation88], and it regulates DNA replication initiation by binding to oriC () [Citation29,Citation71]. The binding of DnaA to oriC in S. elongatus 7942 depends on photosynthesis (see the following section). Notably, the lethal phenotype of a dnaA disruption mutant was suppressed by the integration of an episomal plasmid (pANL) into the chromosome, and in this strain, chromosomal replication was initiated via the plasmid replication initiation system [Citation71].
Table 1. DNA replication factors in cyanobacteria.
Dependence on DnaA varies among cyanobacterial species. In S. sp. 6803 and A. sp. 7120, it has been shown that dnaA is not essential for DNA replication [Citation71,Citation95]. In the symbiotic cyanobacterium Nostoc azolla, the dnaA gene is inactivated by a transposon insertion [Citation96]. dnaA is also not conserved in a diatom endosymbiont of cyanobacterial origin (termed a spheroid body) with a 2.8 Mb genome [Citation97]. Most of the DNA replication enzymes in chloroplasts originated from cyanobacteria and other organisms [Citation98] and are observable vestiges of their symbiotic evolution. However, the only dnaA ortholog is not conserved in the chloroplasts of any plant or alga with sequenced genomes [Citation71]. Genomic analyses suggest that dependence on DnaA varies among cyanobacteria and was lost before the symbiotic relationship that led to the evolution of chloroplast was established.
DNA methylation
DNA methylation is a conserved epigenetic modification that is important for gene regulation and genome stability. Methylation by deoxyadenosine methylase (Dam) plays an important role in DNA replication, DNA repair, and the regulation of gene expression [Citation99–Citation102]. In E. coli, Dam functions as a maintenance methylase during a time window when the chromosome is hemi-methylated following the passage of a replication fork and the parental and daughter strands can be distinguished by their GATC methylation status. The GATC sequences that are hemi-methylated by Dam are involved in multiple aspects of DNA replication, including sequestration of oriC, transcriptional repression of DnaA, and cohesion of sister chromatids in the wake of the progressing replication fork [Citation99,Citation100,Citation102]. In E. coli, although Dam is not essential, a genetic relationship between dam and genes involved in DNA repair has been reported [Citation103–Citation106]. Recently, a novel function for Dam has been reported, the control of aberrant DnaA–oriC-independent replication [Citation107]. Dam-like methylase plays an essential role in V. cholerae, which contains a 1 Mbp secondary chromosome (chromosome II) [Citation108]. Although replication of chromosome II is DnaA-independent, it strictly depends on methylation by Dam [Citation108–Citation111].
In S. sp. 6803, six DNA methylases (M.Ssp6803I–VI) have been identified [Citation112,Citation113]. Among them, M.Ssp6803III and M.Ssp6803IV are Dam-like methyltransferases, encoded by slr1803 and slr6050, respectively, that play crucial roles in S. sp., 6803, as these genes are essential under laboratory conditions [Citation113]. Similar observations have been reported in S. elongatus 7942 and A. sp. 7120 [Citation88,Citation114], and the homologue of M.Ssp6803III in S. elongatus 7942 (Synpcc7942_1790) is also essential. The cytosine methyltransferase M.Ssp6803II, encoded by sll0729, in S. sp. 6803 is also important because it modifies at least 90% of the GGm4CC recognition sequences, and a disruption mutant of sll0729 exhibited a severe growth defect, with a reduction in chlorophyll a content [Citation113]. A suppressor of the sll0729 disruptant decreased the chromosome copy number and increased the levels of DNA topoisomerase 4 subunit A [Citation25], implicating GGm4CC methylation and DNA replication in DNA repair in S. sp. 6803. However, further experiments are needed to clarify the physiological role of methylation in cyanobacteria.
The occurrence of cytosine methylation in plastid DNA has been reported in several plants, including sycamore [Citation115], tomato [Citation116], and maize [Citation117]. It has been suggested that cytosine methylation is involved in the transcriptional regulation and differentiation of plastids, although some observations have cast doubt on its physiological significance [Citation118–Citation121]. In Chlamydomonas reinhardtii, there are two mating types (mt+ and mt-), and the chloroplast DNA in mt+ gametes is methylated by a cytosine DNA methyltransferase [Citation122,Citation123]. Only chloroplast DNA from the mt+ parent remained in the mating cells, thus resulting in uniparental inheritance of the mt+ chloroplast DNA [Citation124]. It has been suggested that the difference in DNA methylation could be responsible for the selection of chloroplast DNA by a specific endonuclease [Citation125] and/or the frequency control of plastid DNA replication in mt- and mt+ cells [Citation126].
Regulation of DNA replication
DNA replication activity, as evaluated by the uptake of BrdU, in three cyanobacterial species, S. elongatus 7942, S. sp. 6803, and A. sp. 7120, increased under light conditions and was inactivated by inhibitors of photosynthetic electron transport (PET) [Citation20,Citation127,Citation128], indicating that activation of DNA replication through PET is conserved among cyanobacteria. In S. elongatus 7942, the binding of DnaA to oriC was promoted under light conditions and was inhibited in the dark or in the presence of PET inhibitors, even though DnaA levels remained constant [Citation29,Citation71]. These findings suggest that in S. elongatus 7942, DNA replication is regulated by the oriC binding activity of DnaA, and not the DnaA protein level, which is associated with the integrity of PET. In contrast, in S. sp. 6803 and A. sp. 7120, DNA replication is regulated by a DnaA-independent mechanism [Citation71]. Therefore, although DNA replication is regulated by the electron transport system, the molecular mechanisms that regulate its initiation probably differ among cyanobacteria.
It has been shown that there are differences in DNA replication under dark conditions among these three aforementioned cyanobacteria [Citation127]. In S. sp. 6803 and A. sp. 7120, DNA replication continued after the bacteria were transferred to the dark, whereas in S. elongatus 7942, DNA replication drastically decreased after transfer to the dark. In cyanobacteria, a portion of the electron transport chain, between plastoquinone (PQ) and plastocyanin (PC), is shared by photosynthesis and respiration. Under dark conditions, the respiratory electron transport (RET) system is activated and PET is inhibited. The respiration activity and ATP content in the dark were higher in S. sp. 6803 and A. sp. 7120 than in S. elongatus 7942, suggesting that the observed differences in metabolic activity are correlated with the distinct DNA replication activities that occur in the dark in cyanobacteria [Citation127].
In the unicellular alga C. reinhardtii, which contains one chloroplast per cell, chloroplast DNA is replicated during the light phase but not during the dark phase, independent of the cell cycle and the timing of chloroplast division in photoautotrophic culture [Citation43]. Inhibition of PET blocked chloroplast DNA replication. However, chloroplast DNA was replicated when the cells were grown heterotrophically in the dark, raising the possibility that chloroplast DNA replication is coupled with the reducing power of photosynthesis or respiration as was observed in cyanobacteria [Citation127].
There are several observations suggesting a relationship between central carbon metabolism and DNA replication in E. coli and B. subtilis [Citation129,Citation130], although this has not yet been confirmed. In these bacteria, central metabolic activities directly affect RET activity. The observation of a relationship between PET or RET and DNA replication in cyanobacteria contributes to our understanding of the fundamental mechanisms of proliferation common among bacteria.
Splicing of DNA replication proteins
Protein splicing is a post-translational process involving a large family of proteins called inteins, which have been identified in all three domains of life [Citation131,Citation132]. Inteins are autocatalytic protein domains that excise protein precursors and ligate their flanking regions to a peptide bond. Most inteins are expressed within a single polypeptide chain (cis-splicing inteins), but some are split into two polypeptides, each containing one extein and one intein fragment (trans-splicing inteins). In archaea and mycobacteria, inteins have been found to be responsive to a range of stressors and environmental conditions, including temperature [Citation133], DNA damage [Citation134], salt [Citation135], redox [Citation135], and reactive oxygen species (ROS) [Citation135]. These conditions are often either highly relevant to the environmental niche of the organism or related to the function of the intein-containing protein.
In cyanobacteria, both cis- and trans-splicing inteins have been identified in DNA replication proteins, except for in marine picocyanobacteria, including P. marinus 1375 () [Citation132]. In S. sp. 6803, three cis-splicing inteins have been identified in DNA helicase (DnaB) [Citation136,Citation137], the τ subunit of DNA polymerase III (DnaX) [Citation138], and the DNA gyrase B subunit (GyrB) () [Citation139]. There are no inteins in DnaX and GyrB in Synechocystis sp. PCC 6714, which is a close relative of S. sp. 6803 [Citation140], although S. sp. 6714 possesses two cis-splicing inteins in DnaB. Another cis-splicing intein has been identified in class II-type ribonucleotide reductase in S. elongatus 7942, A. sp. 7120, and G. violaceus 7421 () [Citation141]. These observations suggest that the acquisition of cis-splicing inteins occurred frequently during cyanobacterial evolution and might be mediated by horizontal gene transfer [Citation132].
It is known that DnaE proteins that are split due to the presence of trans-splicing inteins and are conserved among most freshwater cyanobacteria, including S. elongatus 7942, S. sp. 6803, S. sp. 6714, and A. sp. 7120 () [Citation142,Citation143]. Since a split DnaE is not present in picocyanobacteria or G. violaceus 7421, which are classified in different clades than freshwater cyanobacteria [Citation141,Citation143,Citation144], the process of splitting DnaE with a trans-splicing intein likely occurred in a common ancestor of the freshwater cyanobacteria after the branching of picocyanobacteria and other cyanobacteria. The splicing point of DnaE is identical among cyanobacteria, indicating that the separation event of DnaE protein occurred once in the evolutional history of cyanobacteria. Measurement of in vivo splicing efficiencies and in vitro kinetics revealed that, in cyanobacteria, split DnaE inteins can catalyze protein trans-splicing in tens of seconds [Citation145–Citation147]. Because they splice efficiently and produce fully functional host proteins, inteins are used as biological tools in the fields of protein biology and synthetic biology [Citation145,Citation148–Citation150].
Although there is no direct evidence answering the question of why inteins are present in DNA replication factors in cyanobacteria, it was recently proposed that the DnaB helicase intein functions as an oxidative stress sensor in Mycobacterium smegmatis [Citation151]. There are two cis-splicing inteins within M. smegmatis DnaB helicase, DnaBi1, and DnaBi2 [Citation151]. DnaBi1 splicing is reversibly inhibited by oxidative and nitrosative insults through the formation of an intramolecular disulfide bond in the catalytic cysteine of DnaBi1. Inhibition of cis-splicing in DnaB directly pauses replication and contributes to the maintenance of genome integrity. It is expected that inteins in cyanobacteria may have a similar function because the intracellular conditions in cyanobacteria induce the production of ROS [Citation27]. Therefore, intein regulation might function as a safeguard against replication stress in the presence of excess ROS in cyanobacterial cells. Further studies are needed to characterize the inteins in cyanobacteria.
Acknowledgments
I sincerely thank Dr. Ryudo Ohbayashi and Dr. Kazuharu Arakawa for critical reading of the manuscript and constructive suggestions.
Disclosure statement
No potential conflict of interest was reported by the author.
Additional information
Funding
References
- Oliver NJ, Rabinovitch-Deere CA, Carroll AL, et al. Cyanobacterial metabolic engineering for biofuel and chemical production. Curr Opin Chem Biol. 2016;35:43–50.
- Nozzi NE, Oliver JW, Atsumi S. Cyanobacteria as a platform for biofuel production. Front Bioeng Biotechnol. 2013;1:7.
- Watanabe S, Ozawa H, Kato H, et al. Carbon-free production of 2-deoxy-scyllo-inosose (DOI) in cyanobacterium Synechococcus elongatus PCC 7942. Biosci Biotechnol Biochem. 2018;82(1):161–165.
- Binder BJ, Chisholm SW. Cell cycle regulation in marine Synechococcus sp. strains. Appl Environ Microbiol. 1995;61(2):708–717.
- Burbage CD, Binder BJ. Relationship between cell cycle and light-limited growth rate in oceanic Prochlorococcus (MIT9312) and Synechococcus (WH8103) (Cyanobacteria). J Phycol. 2007;43:2.
- Mori T, Binder B, Johnson CH. Circadian gating of cell division in cyanobacteria growing with average doubling times of less than 24 hours. Proc Natl Acad Sci U S A. 1996;93(19):10183–10188.
- Mann N, Carr NG. Control of macromolecular composition and cell division in the blue-green algae Anacystis nidulans. J Gen Microbiol. 1974;83(2):399–405.
- Minton KW. DNA repair in the extremely radioresistant bacterium Deinococcus radiodurans. Mol Microbiol. 1994;13(1):9–15.
- Ohtani N, Tomita M, Itaya M. An extreme thermophile, Thermus thermophilus, is a polyploid bacterium. J Bacteriol. 2010;192(20):5499–5505.
- Komaki K, Ishikawa H. Intracellular bacterial symbionts of aphids possess many genomic copies per bacterium. J Mol Evol. 1999;48(6):717–722.
- Tobiason DM, Seifert HS. The obligate human pathogen, Neisseria gonorrhoeae, is polyploid. PLoS Biol. 2006;4(6):e185.
- Bresler V, Montgomery WL, Fishelson L, et al. Gigantism in a bacterium, Epulopiscium fishelsoni, correlates with complex patterns in arrangement, quantity, and segregation of DNA. J Bacteriol. 1998;180(21):5601–5611.
- Bendich AJ. Why do chloroplasts and mitochondria contain so many copies of their genome? Bioessays. 1987;6(6):279–282.
- Kuroiwa T. The replication, the replication, differentiation, and inheritance of plastids with emphasis on the concept of organelle nuclei. Int Rev Cytol. 1991;128:1–62.
- Hildenbrand C, Stock T, Lange C, et al. Genome copy numbers and gene conversion in methanogenic archaea. J Bacteriol. 2011;193(3):734–743.
- Breuert S, Allers T, Spohn G, et al. Regulated polyploidy in halophilic archaea. PLoS One. 2006;1:e92.
- Spaans SK, van der Oost J, Kengen SW. The chromosome copy number of the hyperthermophilic archaeon Thermococcus kodakarensis KOD1. Extremophiles. 2015;19(4):741–750.
- Liu X, Miao D, Zhang F, et al. Characterization of the minimal replicon of pHM300 and independent copy number control of major and minor chromosomes of Haloferax mediterranei. FEMS Microbiol Lett. 2013;339(1):66–74.
- Watanabe S, Ohbayashi R, Kanesaki Y, et al. Intensive DNA replication and metabolism during the lag phase in cyanobacteria. PLoS One. 2015;10(9):e0136800.
- Watanabe S, Ohbayashi R, Shiwa Y, et al. Light-dependent and asynchronous replication of cyanobacterial multi-copy chromosomes. Mol Microbiol. 2012;83(4):856–865.
- Griese M, Lange C, Soppa J. Ploidy in cyanobacteria. FEMS Microbiol Lett. 2011;323(2):124–131.
- Labarre J, Chauvat F, Thuriaux P. Insertional mutagenesis by random cloning of antibiotic resistance genes into the genome of the cyanobacterium Synechocystis strain PCC 6803. J Bacteriol. 1989;171(6):3449–3457.
- Zerulla K, Ludt K, Soppa J. The ploidy level of Synechocystis sp. PCC 6803 is highly variable and is influenced by growth phase and by chemical and physical external parameters. Microbiology. 2016;162(5):730–739.
- Klotz A, Georg J, Bučinská L, et al. Awakening of a dormant cyanobacterium from nitrogen chlorosis reveals a genetically determined program. Curr Biol. 2016;26(21):2862–2872.
- Gärtner K, Klähn S, Watanabe S, et al. Cytosine N4-methylation via M.Ssp6803II is involved in the regulation of transcription, fine-tuning of DNA replication and DNA repair in the cyanobacterium. Front Microbiol. 2019;10:1233.
- Sargent EC, Hitchcock A, Johansson SA, et al. Evidence for polyploidy in the globally important diazotroph Trichodesmium. FEMS Microbiol Lett. 2016;363:21.
- Latifi A, Ruiz M, Zhang CC. Oxidative stress in cyanobacteria. FEMS Microbiol Rev. 2009;33(2):258–278.
- Carpenter E, Subramaniam A, Capone D. Biomass and primary productivity of the cyanobacterium Trichodesmium spp. in the tropical N Atlantic ocean. Deep-Sea Res Pt I. 2004;52(9):1787–1788.
- Ohbayashi R, Nakamachi A, Hatakeyama TS, et al. Coordination of polyploid chromosome replication with cell size and growth in a cyanobacterium. MBio. 2019;10:2.
- Zheng XY, O’Shea EK. Cyanobacteria maintain constant protein concentration despite genome copy-number variation. Cell Rep. 2017;19(3):497–504.
- Foster JS, Singh AK, Rothschild LJ, et al. Growth-phase dependent differential gene expression in Synechocystis sp. strain PCC 6803 and regulation by a group 2 sigma factor. Arch Microbiol. 2007;187(4):265–279.
- Broedel SE, Wolf RE. Growth-phase-dependent induction of 6-phosphogluconate dehydrogenase and glucose 6-phosphate dehydrogenase in the cyanobacterium Synechococcus sp. PCC7942. Gene. 1991;109(1):71–79.
- Tandeau de Marsac N, Houmard J. Adaptation of cyanobacteria to environmental stimuli: new steps towards molecular mechanisms. FEMS Microbiol Rev. 1993;104(1):119–189.
- Zerulla K, Soppa J. Polyploidy in haloarchaea: advantages for growth and survival. Front Microbiol. 2014;5:274.
- Ludt K, Soppa J. Polyploidy in halophilic archaea: regulation, evolutionary advantages, and gene conversion. Biochem Soc Trans. 2019;47(3):933–944.
- Golden SS, Canales SR. Cyanobacterial circadian clocks–timing is everything. Nat Rev Microbiol. 2003;1(3):191–199.
- Yang Q, Pando BF, Dong G, et al. Circadian gating of the cell cycle revealed in single cyanobacterial cells. Science. 2010;327(5972):1522–1526.
- Dong G, Golden SS. How a cyanobacterium tells time. Curr Opin Microbiol. 2008;11(6):541–546.
- Mori T. Cell division cycles and circadian rhythms. In: Ditty J, Mackey S, Johnson C, editors. Bacterial circadian programs. Berlin:Springer; 2009. p. 183–204.
- Ochoa de Alda JA, Esteban R, Diago ML, et al. The plastid ancestor originated among one of the major cyanobacterial lineages. Nat Commun. 2014;5:4937.
- Powikrowska M, Oetke S, Jensen PE, et al. Dynamic composition, shaping and organization of plastid nucleoids. Front Plant Sci. 2014;5:424.
- Sakai A, Takano H, Kuroiwa T. Organelle nuclei in higher plants: structure, composition, function, and evolution. Int Rev Cytol. 2004;238:59–118.
- Kabeya Y, Miyagishima SY. Chloroplast DNA replication is regulated by the redox state independently of chloroplast division in Chlamydomonas reinhardtii. Plant Physiol. 2013;161(4):2102–2112.
- Baumgartner BJ, Rapp JC, Mullet JE. Plastid transcription activity and DNA copy number increase early in barley chloroplast development. Plant Physiol. 1989;89(3):1011–1018.
- Lamppa GK, Elliot LV, Bendich AJ. Changes in chloroplast number during pea leaf development: an analysis of a protoplast population. Planta. 1980;148(5):437–443.
- Boffey SA, Ellis JR, Selldén G, et al. Chloroplast division and DNA synthesis in light-grown wheat leaves. Plant Physiol. 1979;64(3):502–505.
- Oldenburg DJ, Bendich AJ. Changes in the structure of DNA molecules and the amount of DNA per plastid during chloroplast development in maize. J Mol Biol. 2004;344(5):1311–1330.
- Rowan BA, Oldenburg DJ, Bendich AJ. RecA maintains the integrity of chloroplast DNA molecules in Arabidopsis. J Exp Bot. 2010;61(10):2575–2588.
- Morley SA, Nielsen BL. Chloroplast DNA copy number changes during plant development in Organelle DNA polymerase mutants. Front Plant Sci. 2016;7:57.
- Watanabe S, Noda A, Ohbayashi R, et al. ParA-like protein influences the distribution of multi-copy chromosomes in cyanobacterium Synechococcus elongatus PCC 7942. Microbiology. 2018;164(1):45–56.
- Murata K, Hagiwara S, Kimori Y, et al. Ultrastructure of compacted DNA in cyanobacteria by high-voltage cryo-electron tomography. Sci Rep. 2016;6:34934.
- Long BM, Badger MR, Whitney SM, et al. Analysis of carboxysomes from Synechococcus PCC7942 reveals multiple Rubisco complexes with carboxysomal proteins CcmM and CcaA. J Biol Chem. 2007;282(40):29323–29335.
- Lee PS, Grossman AD. The chromosome partitioning proteins Soj (ParA) and Spo0J (ParB) contribute to accurate chromosome partitioning, separation of replicated sister origins, and regulation of replication initiation in Bacillus subtilis. Mol Microbiol. 2006;60(4):853–869.
- Real G, Autret S, Harry EJ, et al. Cell division protein DivIB influences the Spo0J/Soj system of chromosome segregation in Bacillus subtilis. Mol Microbiol. 2005;55(2):349–367.
- Vallet-Gely I, Boccard F. Chromosomal organization and segregation in Pseudomonas aeruginosa. PLoS Genet. 2013;9(5):e1003492.
- Fogel MA, Waldor MK. A dynamic, mitotic-like mechanism for bacterial chromosome segregation. Genes Dev. 2006;20(23):3269–3282.
- Kadoya R, Baek JH, Sarker A, et al. Participation of chromosome segregation protein ParAI of Vibrio cholerae in chromosome replication. J Bacteriol. 2011;193(7):1504–1514.
- Savage DF, Afonso B, Chen AH, et al. Spatially ordered dynamics of the bacterial carbon fixation machinery. Science. 2010;327(5970):1258–1261.
- MacCready JS, Hakim P, Young EJ, et al. Protein gradients on the nucleoid position the carbon-fixing organelles of cyanobacteria. Elife. 2018;7:e39723.
- Schumacher MA, Henderson M, Zhang H. Structures of maintenance of carboxysome distribution Walker-box McdA and McdB adaptor homologs. Nucleic Acids Res. 2019;47(11):5950–5962.
- Graumann PL. SMC proteins in bacteria: condensation motors for chromosome segregation? Biochimie. 2001;83(1):53–59.
- Lobry JR. Asymmetric substitution patterns in the two DNA strands of bacteria. Mol Biol Evol. 1996;13(5):660–665.
- Frank AC, Lobry JR. Asymmetric substitution patterns: a review of possible underlying mutational or selective mechanisms. Gene. 1999;238(1):65–77.
- Luo H, Gao F. DoriC 10.0: an updated database of replication origins in prokaryotic genomes including chromosomes and plasmids. Nucleic Acids Res. 2019;47(D1):D74–D77.
- Arakawa K, Tomita M. Measures of compositional strand bias related to replication machinery and its applications. Curr Genomics. 2012;13(1):4–15.
- Arakawa K, Suzuki H, Tomita M. Quantitative analysis of replication-related mutation and selection pressures in bacterial chromosomes and plasmids using generalised GC skew index. BMC Genomics. 2009;10:640.
- Arakawa K, Tomita M. The GC skew index: a measure of genomic compositional asymmetry and the degree of replicational selection. Evol Bioinform Online. 2007;3:159–168.
- Arakawa K, Kido N, Oshita K, et al. G-language genome analysis environment with REST and SOAP web service interfaces. Nucleic Acids Res. 2010;38(Web Server issue):W700–5.
- Liu Y, Tsinoremas NF. An unusual gene arrangement for the putative chromosome replication origin and circadian expression of dnaN in Synechococcus sp. strain PCC 7942. Gene. 1996;172(1):105–109.
- Zhou Y, Chen WL, Wang L, et al. Identification of the oriC region and its influence on heterocyst development in the filamentous cyanobacterium Anabaena sp. strain PCC 7120. Microbiology. 2011;157(Pt 7):1910–1919.
- Ohbayashi R, Watanabe S, Ehira S, et al. Diversification of DnaA dependency for DNA replication in cyanobacterial evolution. Isme J. 2016;10(5):1113–1121.
- Gao F. Bacteria may have multiple replication origins. Front Microbiol. 2015;6:324.
- Gao F. Recent advances in the identification of replication origins based on the Z-curve method. Curr Genomics. 2014;15(2):104–112.
- Nielsen BL, Cupp JD, Brammer J. Mechanisms for maintenance, replication, and repair of the chloroplast genome in plants. J Exp Bot. 2010;61(10):2535–2537.
- Maréchal A, Brisson N. Recombination and the maintenance of plant organelle genome stability. New Phytol. 2010;186(2):299–317.
- Chiu WL, Sears BB. Electron microscopic localization of replication origins in oenothera chloroplast DNA. Mol Gen Genet. 1992;232(1):33–39.
- Kunnimalaiyaan M, Nielsen BL. Fine mapping of replication origins (Ori A and ori B) in Nicotiana tabacum chloroplast DNA. Nucleic Acids Res. 1997;25(18):3681–3686.
- Kolodner RD, Tewari KK. Chloroplast DNA from higher plants replicates by both the cairns and the rolling circle mechanism. Nature. 1975;256(5520):708–711.
- Wang JD, Levin PA. Metabolism, cell growth and the bacterial cell cycle. Nat Rev Microbiol. 2009;7(11):822–827.
- Yoshikawa H, O’Sullivan A, Sueoka N. Sequential replication of the Bacillus subtilis chromosome. 3. Regulation of initiation. Proc Natl Acad Sci U S A. 1964;52:973–980.
- Srivatsan A, Han Y, Peng J, et al. High-precision, whole-genome sequencing of laboratory strains facilitates genetic studies. PLoS Genet. 2008;4(8):e1000139.
- Kono N, Arakawa K, Sato M, et al. Undesigned selection for replication termination of bacterial chromosomes. J Mol Biol. 2014;426(16):2918–2927.
- Chen AH, Afonso B, Silver PA, et al. Spatial and temporal organization of chromosome duplication and segregation in the cyanobacterium Synechococcus elongatus PCC 7942. PLoS One. 2012;7(10):e47837.
- Jain IH, Vijayan V, O’Shea EK. Spatial ordering of chromosomes enhances the fidelity of chromosome partitioning in cyanobacteria. Proc Natl Acad Sci U S A. 2012;109(34):13638–13643.
- Berlatzky IA, Rouvinski A, Ben-Yehuda S. Spatial organization of a replicating bacterial chromosome. Proc Natl Acad Sci U S A. 2008;105(37):14136–14140.
- Niki H, Yamaichi Y, Hiraga S. Dynamic organization of chromosomal DNA in Escherichia coli. Genes Dev. 2000;14(2):212–223.
- Schneider D, Fuhrmann E, Scholz I, et al. Fluorescence staining of live cyanobacterial cells suggest non-stringent chromosome segregation and absence of a connection between cytoplasmic and thylakoid membranes. BMC Cell Biol. 2007;8:39.
- Rubin BE, Wetmore KM, Price MN, et al. The essential gene set of a photosynthetic organism. Proc Natl Acad Sci U S A. 2015;112(48):E6634–6643.
- Katayama T, Ozaki S, Keyamura K, et al. Regulation of the replication cycle: conserved and diverse regulatory systems for DnaA and oriC. Nat Rev Microbiol. 2010;8(3):163–170.
- Skarstad K, Katayama T. Regulating DNA replication in bacteria. Cold Spring Harb Perspect Biol. 2013;5(4):a012922.
- Skarstad K, Boye E, Steen HB. Timing of initiation of chromosome replication in individual Escherichia coli cells. Embo J. 1986;5(7):1711–1717.
- Keyamura K, Abe Y, Higashi M, et al. DiaA dynamics are coupled with changes in initial origin complexes leading to helicase loading. J Biol Chem. 2009;284(37):25038–25050.
- Keyamura K, Fujikawa N, Ishida T, et al. The interaction of DiaA and DnaA regulates the replication cycle in E. coli by directly promoting ATP DnaA-specific initiation complexes. Genes Dev. 2007;21(16):2083–2099.
- Ishida T, Akimitsu N, Kashioka T, et al. DiaA, a novel DnaA-binding protein, ensures the timely initiation of Escherichia coli chromosome replication. J Biol Chem. 2004;279(44):45546–45555.
- Richter S, Hagemann M, Messer W. Transcriptional analysis and mutation of a dnaA-like gene in Synechocystis sp. strain PCC 6803. J Bacteriol. 1998;180(18):4946–4949.
- Ran L, Larsson J, Vigil-Stenman T, et al. Genome erosion in a nitrogen-fixing vertically transmitted endosymbiotic multicellular cyanobacterium. PLoS One. 2010;5(7):e11486.
- Nakayama T, Kamikawa R, Tanifuji G, et al. Complete genome of a nonphotosynthetic cyanobacterium in a diatom reveals recent adaptations to an intracellular lifestyle. Proc Natl Acad Sci U S A. 2014;111(31):11407–11412.
- Moriyama T, Sato N. Enzymes involved in organellar DNA replication in photosynthetic eukaryotes. Front Plant Sci. 2014;5:480.
- Casadesús J. Bacterial DNA methylation and methylomes. Adv Exp Med Biol. 2016;945:35–61.
- Wion D, Casadesús J. N6-methyl-adenine: an epigenetic signal for DNA-protein interactions. Nat Rev Microbiol. 2006;4(3):183–192.
- Erova TE, Kosykh VG, Sha J, et al. DNA adenine methyltransferase (Dam) controls the expression of the cytotoxic enterotoxin (act) gene of Aeromonas hydrophila via tRNA modifying enzyme-glucose-inhibited division protein (GidA). Gene. 2012;498(2):280–287.
- Løbner-Olesen A, Skovgaard O, Marinus MG. Dam methylation: coordinating cellular processes. Curr Opin Microbiol. 2005;8(2):154–160.
- McGraw BR, Marinus MG. Isolation and characterization of Dam+ revertants and suppressor mutations that modify secondary phenotypes of dam-3 strains of Escherichia coli K-12. Mol Gen Genet. 1980;178(2):309–315.
- Marinus MG. Recombination is essential for viability of an Escherichia coli dam (DNA adenine methyltransferase) mutant. J Bacteriol. 2000;182(2):463–468.
- Glickman BW, Radman M. Escherichia coli mutator mutants deficient in methylation-instructed DNA mismatch correction. Proc Natl Acad Sci U S A. 1980;77(2):1063–1067.
- Harinarayanan R, Gowrishankar J. A dnaC mutation in Escherichia coli that affects copy number of ColE1-like plasmids and the PriA-PriB (but not Rep-PriC) pathway of chromosomal replication restart. Genetics. 2004;166(3):1165–1176.
- Raghunathan N, Goswami S, Leela JK, et al. A new role for Escherichia coli Dam DNA methylase in prevention of aberrant chromosomal replication. Nucleic Acids Res. 2019;47(11):5698–5711.
- Demarre G, Chattoraj DK. DNA adenine methylation is required to replicate both Vibrio cholerae chromosomes once per cell cycle. PLoS Genet. 2010;6(5):e1000939.
- Egan ES, Waldor MK. Distinct replication requirements for the two Vibrio cholerae chromosomes. Cell. 2003;114(4):521–530.
- Val ME, Soler-Bistué A, Bland MJ, et al. Management of multipartite genomes: the Vibrio cholerae model. Curr Opin Microbiol. 2014;22:120–126.
- Duigou S, Knudsen KG, Skovgaard O, et al. Independent control of replication initiation of the two Vibrio cholerae chromosomes by DnaA and RctB. J Bacteriol. 2006;188(17):6419–6424.
- Scharnagl M, Richter S, Hagemann M. The cyanobacterium Synechocystis sp. strain PCC 6803 expresses a DNA methyltransferase specific for the recognition sequence of the restriction endonuclease PvuI. J Bacteriol. 1998;180(16):4116–4122.
- Hagemann M, Gärtner K, Scharnagl M, et al. Identification of the DNA methyltransferases establishing the methylome of the cyanobacterium Synechocystis sp. PCC 6803. DNA Res. 2018;25(4):343–352.
- Matveyev AV, Young KT, Meng A, et al. DNA methyltransferases of the cyanobacterium Anabaena PCC 7120. Nucleic Acids Res. 2001;29(7):1491–1506.
- Ngernprasirtsiri J, Kobayashi H, Akazawa T. DNA methylation as a mechanism of transcriptional regulation in nonphotosynthetic plastids in plant cells. Proc Natl Acad Sci U S A. 1988;85(13):4750–4754.
- Ngernprasirtsiri J, Kobayashi H, Akazawa T. DNA methylation occurred around lowly expressed genes of plastid DNA during tomato fruit development. Plant Physiol. 1988;88(1):16–20.
- Ngernprasirtsiri J, Chollet R, Kobayashi H, et al. DNA methylation and the differential expression of C4 photosynthesis genes in mesophyll and bundle sheath cells of greening maize leaves. J Biol Chem. 1989;264(14):8241–8248.
- Fojtová M, Kovarík A, Matyásek R. Cytosine methylation of plastid genome in higher plants. Fact or artefact? Plant Sci. 2001;160(4):585–593.
- Ahlert D, Stegemann S, Kahlau S, et al. Insensitivity of chloroplast gene expression to DNA methylation. Mol Genet Genomics. 2009;282(1):17–24.
- Marano MR, Carrillo N. Chromoplast formation during tomato fruit ripening. No evidence for plastid DNA methylation. Plant Mol Biol. 1991;16(1):11–19.
- Tomas R, Vera A, Martin M, et al. Changes in protein synthesis without evidence of DNA methylation in barley chloroplasts during leaf growth and development. Plant Sci. 1992;85(1):71–77.
- Nishiyama R, Wada Y, Mibu M, et al. Role of a nonselective de novo DNA methyltransferase in maternal inheritance of chloroplast genes in the green alga, Chlamydomonas reinhardtii. Genetics. 2004;168(2):809–816.
- Nishiyama R, Ito M, Yamaguchi Y, et al. A chloroplast-resident DNA methyltransferase is responsible for hypermethylation of chloroplast genes in Chlamydomonas maternal gametes. Proc Natl Acad Sci U S A. 2002;99(9):5925–5930.
- Nishimura Y, Misumi O, Matsunaga S, et al. The active digestion of uniparental chloroplast DNA in a single zygote of Chlamydomonas reinhardtii is revealed by using the optical tweezer. Proc Natl Acad Sci U S A. 1999;96(22):12577–12582.
- Nishimura Y, Misumi O, Kato K, et al. An mt(+) gamete-specific nuclease that targets mt(-) chloroplasts during sexual reproduction in C. reinhardtii. Genes Dev. 2002;16(9):1116–1128.
- Umen JG, Goodenough UW. Chloroplast DNA methylation and inheritance in Chlamydomonas. Genes Dev. 2001;15(19):2585–2597.
- Ohbayashi R, Yamamoto JY, Watanabe S, et al. Variety of DNA replication activity among cyanobacteria correlates with distinct respiration activity in the dark. Plant Cell Physiol. 2017;58(2):279–286.
- Ohbayashi R, Watanabe S, Kanesaki Y, et al. DNA replication depends on photosynthetic electron transport in cyanobacteria. FEMS Microbiol Lett. 2013;344(2):138–144.
- Maciąg M, Nowicki D, Janniere L, et al. Genetic response to metabolic fluctuations: correlation between central carbon metabolism and DNA replication in Escherichia coli. Microb Cell Fact. 2011;10:19.
- Jannière L, Canceill D, Suski C, et al. Genetic evidence for a link between glycolysis and DNA replication. PLoS One. 2007;2(5):e447.
- Mills KV, Perler FB. The mechanism of intein-mediated protein splicing: variations on a theme. Protein Pept Lett. 2005;12(8):751–755.
- Novikova O, Topilina N, Belfort M. Enigmatic distribution, evolution, and function of inteins. J Biol Chem. 2014;289(21):14490–14497.
- Topilina NI, Novikova O, Stanger M, et al. Post-translational environmental switch of RadA activity by extein-intein interactions in protein splicing. Nucleic Acids Res. 2015;43(13):6631–6648.
- Lennon CW, Stanger M, Belfort M. Protein splicing of a recombinase intein induced by ssDNA and DNA damage. Genes Dev. 2016;30(24):2663–2668.
- Reitter JN, Cousin CE, Nicastri MC, et al. Salt-dependent conditional protein splicing of an intein from Halobacterium salinarum. Biochemistry. 2016;55(9):1279–1282.
- Pietrokovski S. A new intein in cyanobacteria and its significance for the spread of inteins. Trends Genet. 1996;12(8):287–288.
- Williams NK, Prosselkov P, Liepinsh E, et al. In vivo protein cyclization promoted by a circularly permuted Synechocystis sp. PCC6803 DnaB mini-intein. J Biol Chem. 2002;277(10):7790–7798.
- Liu XQ, Hu Z. Identification and characterization of a cyanobacterial DnaX intein. FEBS Lett. 1997;408(3):311–314.
- Gorbalenya AE. Non-canonical inteins. Nucleic Acids Res. 1998;26(7):1741–1748.
- Kopf M, Klähn S, Pade N, et al. Comparative genome analysis of the closely related Synechocystis strains PCC 6714 and PCC 6803. DNA Res. 2014;21(3):255–266.
- Nakamura Y, Kaneko T, Sato S, et al. Complete genome structure of Gloeobacter violaceus PCC 7421, a cyanobacterium that lacks thylakoids. DNA Res. 2003;10(4):137–145.
- Wu H, Hu Z, Liu XQ. Protein trans-splicing by a split intein encoded in a split DnaE gene of Synechocystis sp. PCC6803. Proc Natl Acad Sci U S A. 1998;95(16):9226–9231.
- Caspi J, Amitai G, Belenkiy O, et al. Distribution of split DnaE inteins in cyanobacteria. Mol Microbiol. 2003;50(5):1569–1577.
- Gupta RS, Mathews DW. Signature proteins for the major clades of cyanobacteria. BMC Evol Biol. 2010;10:24.
- Shah NH, Dann GP, Vila-Perelló M, et al. Ultrafast protein splicing is common among cyanobacterial split inteins: implications for protein engineering. J Am Chem Soc. 2012;134(28):11338–11341.
- Cheriyan M, Pedamallu CS, Tori K, et al. Faster protein splicing with the Nostoc punctiforme DnaE intein using non-native extein residues. J Biol Chem. 2013;288(9):6202–6211.
- Zettler J, Schütz V, Mootz HD. The naturally split Npu DnaE intein exhibits an extraordinarily high rate in the protein trans-splicing reaction. FEBS Lett. 2009;583(5):909–914.
- Kim WJ, Lee SM, Um Y, et al. Development of SyneBrick vectors as a synthetic biology platform for gene expression in Synechococcus elongatus PCC 7942. Front Plant Sci. 2017;8:293.
- Schaerli Y, Gili M, Isalan M. A split intein T7 RNA polymerase for transcriptional AND-logic. Nucleic Acids Res. 2014;42(19):12322–12328.
- Stevens AJ, Sekar G, Shah NH, et al. A promiscuous split intein with expanded protein engineering applications. Proc Natl Acad Sci U S A. 2017;114(32):8538–8543.
- Kelley DS, Lennon CW, Li Z, et al. Mycobacterial DnaB helicase intein as oxidative stress sensor. Nat Commun. 2018;9(1):4363.