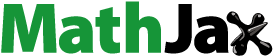
ABSTRACT
The presence of heavy metals and/or harmful bacteria in drinking water represents significant risks to human health. This study aimed to develop a low-cost water treatment technology using synthesized nanocomposites with metal nanoparticles supported on activated carbon (AC) for bacteria and heavy metal removal. In addition, the performance of the developed nanomaterials was compared with that of commercial materials – carbon fibers of three different typologies. The chemical and textural properties of all tested materials were characterized. To simulate a technology to be applied in a water outlet point, removal tests were carried out in a continuous system using suspensions of Escherichia coli and/or Staphylococcus aureus, wherein the contact time with the two phases was minimal (1 min). The obtained results revealed that iron and copper oxides supported on AC with a calcination treatment (CuFeO/AC-C) was the nanocomposite with the best performance, achieving a 6 log reduction for both bacteria in the same suspension up to 9 h operation. A mix of bacteria and heavy metals, simulating a real water, was treated with CuFeO/AC-C obtaining a 6 log reduction of bacteria, a Pb2+ removal >99.9% and Cd2+ removal between 97 and 98% over 180 passage times.
1. Introduction
Available water for consumption has been scarcer over time due to the uncontrollable use of natural resources, pollution, climate changes and the increasing world population density [Citation1–3]. Infectious diseases caused by pathogenic bacteria are the most common and widespread health risk associated with drinking-water [Citation4]. Due to their non-degradability and persistency, heavy metals are pollutants of major concern because they are reported to cause carcinogenic diseases and damage renal nervous and reproductive systems [Citation5,Citation6]. These facts raise some concern, specifically in developing countries, whose low economic power makes it difficult to establish water treatment processes, such as water treatment plants and desalination plants to provide drinking water for their population [Citation7,Citation8]. Physical adsorption of contaminants onto nanomaterials is one of the mechanisms for water treatment, where Van der Waals and electrostatic forces between the adsorbate and adsorbent are established [Citation1]. Adsorption processes are flexible, easy to operate and provide low energy consumption and low operating cost [Citation9]; therefore, they are a good alternative to expensive water treatment technologies.
The use of nanomaterials for water treatment has been studied, showing high removal of contaminants, including bacteria and heavy metals. Carbon nanotubes (CNTs), carbon fibers (CFs), graphene, activated carbon (AC) and metal nanoparticles (MNPs) are some of the nanoadsorbents most studied for water treatment due to their properties, such as high specific surface area and porosity, that provide good adsorption capacity for different types of contaminants [Citation1,Citation10–13].
Few studies report the use of MNPs for bacteria removal from water, and the mechanism behind the bacterial removal using nanomaterials is not well-defined. Various mechanisms have been proposed:
(ii) Adsorption of bacteria due to the electrostatic forces established between the positive charge of the MNPs cations and the negative charge of the cellular membrane of bacteria provided by the organic anions that constitute it (carboxylic acid groups in the proteins) [Citation14].
(iii) MNPs are attracted to the bacteria surface and wrap them causing an accumulation of MNPs on the surface that originates hypertrophy and membrane disruption, with the eventual entrance of toxic compounds, leading to inhibition of cellular growth or even death [Citation12]. Indeed, Liu et al. [Citation15] demonstrated by Raman spectroscopy an increase of lipids and proteins with the exposure of ZnO nanoparticles, as well as C-H2 and C = C deformation in the lipids of cell membranes, causing leakage of intercellular contents and the permeability for ZnO nanoparticles entrance. Released metal ions from MNPs in cells are reduced to metal atoms by thiol groups of proteins, thus inactivating the essential enzymes, blocking respiration, and leading to cell wall destruction and cell lysis. Zarchi et al. [Citation16] reported that MNPs (TiO2 and CdO) presented a strong binding to the outer membrane of Escherichia coli, which caused an inhibition of the active transport and the activities of dehydrogenase and periplasmatic enzymes.
(iiii) Production of secondary toxic products, such as reactive oxygen species (ROS) – superoxide anion, hydroxyl radicals, hydrogen peroxide and organic hydroperoxide – which cause damage to almost all organic biomolecules (carbohydrates, lipids, nucleic acids and proteins) [Citation12]. The physicochemical properties of MNPs, such as surface area, diffusibility and electrophilic nature, determine the amount of ROS produced in bacteria [Citation17], and each species of ROS causes cellular damage by different ways [Citation18–20];
(ivi) MNPs ions get inside of cellular medium causing different ways of damage on bacteria (protein inactivation and disruption of DNA synthesis process) [Citation10,Citation12,Citation21–23]. The metal ions catalyse the oxidation of amino acid side chains resulting in protein-bound carbonyls, and this carbonylation phenomena will lead to a loss of catalytic activity in the case of enzymes, triggering protein degradation. For example, Ag particles which act as a weak acid, tend to react with soft bases (sulphur and phosphorus), which are components of proteins and DNA [Citation24], damaging DNA and thus leading to cell death. The effect of MNPs in DNA replication is reported as a dephosphorylation reaction of the phosphotyrosine residues (essential components of the signal transduction mechanism in bacteria), thus inhibiting signal transduction and blocking the growth of bacteria [Citation25,Citation26].
It is possible to create a nanocomposite by combining MNPs with carbon materials. The resultant nanocomposite has improved properties when compared to those of each material individually [Citation10,Citation27]. In addition, the use of nanocomposites instead of MNPs or carbon materials separately is advantageous because there is a dispersion of MNPs on the surface of the carbon materials, with a better control of the particle size while avoiding the aggregation of MNPs [Citation10,Citation27,Citation28]. AC has the advantages of having higher surface area and well-defined porosity [Citation29,Citation30] which permits to act as a good filter for removal water contaminants. Due to the textural properties of this material, it is possible to obtain an efficient dispersion of MNPs onto the AC surface, obtaining a composite. Also, it is a nontoxic carbon allotrope, in terms of ecotoxicity, when compared with CNTs [Citation31] and less expensive, when compared with CNTs and graphene The heavy metal removal using nanocomposites with MNPs in oxide form is also carried out with electrostatic forces, but in this case, the oxygen groups of metal oxides provide a negative charge that attracts heavy metal cations present in the water [Citation32]. Nanocomposites can be synthesized by different techniques, including physical methods such as dispersion in an aqueous medium with colloidal routes, and chemical methods like co-precipitation [Citation33]. In terms of the separation step of nanocomposite particles and treated water, a magnetic field can be applied when the used nanocomposite is composed of magnetic metals [Citation34].
In this study, different nanocomposites were prepared using AC as physical support for MNPs of iron, copper, and silver oxides, due to the reported bactericide properties of these metals [Citation13,Citation35], and tested for their efficacy on the removal of bacteria and/or heavy metals. Moreover, nanocomposites with N-doped AC were tested since nitrogen-containing groups have shown enhancements in bacteria and heavy metal removal [Citation36,Citation37]. The mechanism behind the bacteria and heavy metal removal with functionalized materials with amine groups involves electrostatic forces between the positive charge of MNPs and the negative charge of contaminants [Citation38]. Additionally, it is reported that the amine groups ensure a high binding affinity to at least eight different species of Gram-positive and Gram-negative bacteria [Citation39].
Some CFs are used in water treatment systems working like filters [Citation40]. There are different types of CFs that depend on their composition. The raw materials of CF are polyacrylonitrile (PAN) fibres, cellulose fibres, phenolic resin fibres and, depending on the composition of each type of fibre, cloth or felt CFs are obtained [Citation41,Citation42]. To compare the performance of nanocomposites with AC and CFs, three different commercial CFs-activated carbon fiber cloth (CF-ACF); felt activated carbon fiber (CF-SOFT) and a felt carbon fibre impregnated with casein (milk protein) (CF-CAS) – were tested to remove bacteria from water. CF-CAS was selected for bacteria removal tests due to the reported studies of antibacterial properties of casein [Citation43–45]. The antibacterial mechanism of this protein is explained by strong electrostatic interactions between positive charged casein (NH3+) chains and the negatively charged bacterial cell membranes due to the presence of phospholipids which are equilibrated by divalent cations such as Ca2+ and Mg2+. When casein interacts with bacteria, electrostatic interactions between phospholipids and casein cations are stronger than the divalent cations of the bacteria. Therefore, these counter-ions are released and the cell membrane loses its integrity, causing cell death [Citation46].
The bacteria removal tests were carried out in a continuous system using suspensions of E. coli and/or Staphylococcus aureus. Heavy metal removal tests were performed, firstly, in a batch system to select the material with the best properties for cadmium (Cd2+) and lead (Pb2+) removal, followed by Cd2+ and Pb2+ removal tests in a continuous system.
2. Experimental
2.1. Materials
The reagents used to synthesize nanocomposites: NaOH, HCl, and ethanol 96 vol. % were purchased from VWR Chemicals®, FeCl3·6H2O from Alfa Aesar company, FeCl2·4H2O from Sigma-Aldrich® company, Cu(NO3)2·3H2O from Merck KGaA company, AgNO3 from PanReac AppliChem company, Melamine from Honeywell FlukaTM and granular activated carbon from CABOT. Pb(NO3)2 purchased from Honeywell FlukaTM and CdCl2 from Sigma-Aldrich® company were used in aqueous solutions for heavy metal removal assays.
The tested commercial materials CF-ACF and CF-SOFT were purchased from KurarayTM company and CF-CAS was supplied by Bosch Termotecnologia (Aveiro, Portugal).
2.2. Synthesis of nanocomposites
Iron oxide supported on AC (FeO/AC) and iron and copper oxide supported on AC (CuFeO/AC) were synthesized using a method of co-precipitation previously reported [Citation47,Citation48], wherein 40 mmol of FeCl3·6H2O and 20 mmol of FeCl2·4H2O were dissolved in a solution of 2 M HCl, adding 1 g of AC. Then, the iron precursor mixture was dropped in a solution of 1.5 M NaOH with vigorous mechanical stirring. The precipitation reaction formed FeO/AC and stirring was maintained for 30 min. CuFeO/AC synthesis was carried out by dissolving 40 mmol of FeCl3·6H2O in deionized water (solution A) and 20 mmol of Cu(NO3)2·3H2O was dissolved in a solution of 0.5 M HCl (solution B). Solutions A and B were heated to 50°C and a solution of 3 M NaOH was heated to 100°C. Before precipitation reaction, the solutions A and B were mixed and 1 g of AC was added; then the suspension was dropped into 3 M NaOH solution with vigorous mechanical stirring, which was maintained for 2 h. Some of FeO/AC and CuFeO/AC were submitted to a heat treatment at 200°C for 2 h with N2 gas flow, resulting in FeO/AC-C and CuFeO-C, respectively. The performance and metal(s) leaching of the heat-treated and non-treated nanocomposites was further compared.
To assess the bactericide properties of silver, a nanocomposite of AC impregnated with 5% of silver was prepared by an incipient wetness impregnation [Citation27] using Ag(NO3)2 as a metal precursor. After drying, a thermal treatment at 200°C for 2 h with N2 gas flow was carried out, which resulted in 5% AgO/AC. Oxide state of silver was chosen to get the presence of silver particles due to the reported information about the importance of the oxidation states of silver that can provide toxic Ag+ ions for bacteria, wherein is more available with silver oxide than zero valency Ag [Citation13]. 5% AgO/FeO/AC and 5% CuO/AC nanocomposites were prepared using the same method, substituting Ag(NO3)2 by Cu(NO3)2·3H2O for 5% CuO/AC, and impregnating Ag (Ag(NO3)2) in the previously prepared FeO/AC for 5% AgO/FeO/AC. The N-doped AC nanocomposite was prepared by three steps: (1) solvothermal N-doping AC [Citation49] by dispersing 0.39 g melamine and 1 g AC in 100 mL of ethanol 96 vol.% followed by mixing for 24 h. After that, the particles were filtered and dried at 100°C. Afterwards, a heat treatment was carried out at 600°C for 1 h with N2 gas, obtaining AC doped with nitrogen groups (NQ – quaternary, N5 – pyrrolic, N6 – pyridinic [Citation50]) named as AC@N; (2) co-precipitation of CuFeO following the same procedure of CuFeO/AC synthesis and (3) calcination at 200°C for 2 h with N2 gas flow, obtaining CuFeO/AC@N–C. The particle’s granulometry was controlled and established to be between 0.1 and 0.3 mm because small nanoparticles have been reported with high antimicrobial activity than larger ones [Citation51–53]. CFs tests were carried out by cutting circular samples of each CF and stack them in the reactor to obtain the desired size of fixed bed. Considering previous studies reporting the use of nanocomposites prepared with similar methods (differing the carbon support), it was admitted that all the synthesized nanocomposites contain nanoscale particles, since transmission electron microscopy (TEM) analysis suggested that all the metallic particles presented in the carbon support are at the nanoscale (less than 100 nm) [Citation47, Citation54].
2.3. Characterization methods
The studied materials were characterized by thermogravimetric analysis (TGA, Netzsch STA 409 PC Luxx®) to determine the amount of carbon and metal present, and their thermostability in a temperature range of 50–900°C in N2 and air atmosphere. The textural properties were determined by N2 physisorption at −196°C (Quantachrome NOVA 4200e) and the resulting isotherms allowed to determinate specific surface areas using the Brunaer, Emmett and Teller (BET) method (SBET) [Citation55]. Mesopore area (SMESO) and micropore volume (VMICRO) were determined using the t-method and the total volume of pores was measured at a relative pressure of 0.95 (VP, P/P0 = 0.95) [Citation56]. Temperature programmed reduction (TPR, AMI-200 Altamira Instruments) using a thermal conductivity detector (TCD) was carried out to determine reduction states of the metals present in nanocomposites and to quantify H2 consumption in the reduction reactions. Elemental analysis (vario Micro Analysis CHNS and OXY cube analyser) was performed to determine the amount of carbon, hydrogen, nitrogen, sulphur, and oxygen present in commercial and N-doped materials. Temperature programmed desorption coupled to a mass spectrometry detector (TPD-MS, AMI-300, Altamira Instruments connected to Dycor Dymaxion Mass Spectrometer) was performed to study the oxygen-containing surface groups on these materials. Scanning Electron Microscopy (SEM) was performed to analyse bacteria morphology when in contact with silver particles to verify the reported bactericide properties of silver nanoparticles. In the assay wherein 5% AgO/AC and 5% AgO/FeO/AC were tested, each treated suspension was collected and filtered through a cellulose nitrate membrane (0.22 µm porosity, Whatman). Bacterial cells were then fixed by immersing with 10 mL of glutaraldehyde (4 vol. %) for 24 h. After that, the reminiscent glutaraldehyde was filtered, and the dehydration step was performed with ethanol washes at increasing concentrations (25, 50, 70, 80, 90 and 100 vol. %) for 10 min, each. As a control, a bacterial suspension in the absence of particles was submitted at the same procedure. For SEM analysis, the membranes were coated with Au/Pd thin films by sputtering using SPI Module Sputter Coater equipment, and images were obtained with a high-resolution environmental scanning electron microscope (Schottky) with X-ray microanalysis and diffused retro electron diffraction pattern analysis: Quanta 400FEG ESEM/EDAX Genesis X4M. The crystalline structure of the materials was obtained by powder XRD analysis with a PANalytical X’Pert PRO diffractometer with Cu Kα radiation source (λ = 1.54 nm).
2.4. Heavy metals removal experiments
The batch experiments were performed to evaluate adsorption efficiency at room temperature, wherein 20 mg of material were used for treating 20 mL of a 25 mg L−1 Cd2+ solution (CdCl2 precursor) at pH 6, and a 25 mg L−1 Pb2+ solution at pH 5 (Pb(NO3)2 precursor), separately. Considering reported studies about the effect of pH on the adsorption of Cd2+ and Pb2+, solutions at pH 6 and 5, respectively, allow better adsorption capacities [Citation57]. These solutions were prepared with deionized water and the selected concentration of 25 mg L−1 was chosen to access a well-defined performance of each nanocomposite. Samples were taken at different contact times with adsorbents (2, 5, 10, 20, 30, 60 and 120 min) to determine the kinetics. To ensure an efficient suspension of the nanocomposites in the heavy metals’ solutions, the samples were kept shaking in a bath-shaker for each contact time. The Inductively Coupled Plasma (ICP-OES ICAP 7400 THERMO) technique was applied to determine the concentrations of Cd2+ and Pd2+.
The heavy metal removal tests in a continuous system were performed with the material which exhibited the best results in the batch assays and for that, a solution with 25 mg L−1 of Cd2+ and 25 mg L−1 Pb2+ were prepared, followed by measurements of adsorbed heavy metals by ICP technique. However, it should be highlighted that this cadmium and lead initial concentration is far above that found in real water samples [Citation58, Citation59]. Therefore, to assess the performance at a real concentration of these metals in water, the material with the best performance was tested in another batch and continuous assays carried out with 0.10 mg L−1 of each metal. The chosen concentration of 0.10 mg L−1 was detected in some freshwater samples (see Online Resource, Figure S1) and is above the legal limits for each heavy metal: 0.003 and 0.010 mg L−1 of Cd2+ and Pb2+, respectively (set by World Health Organization, [Citation4]).
2.5. Continuous system assays
A Omnifit® column (Merck) with a radius of 0.5 cm was used in continuous assays. The nanocomposites and CFs were sterilized at 160°C for 2 h, except for CF-CAS because this material could lose its active phase (casein) by protein denaturation (78°C for casein, [Citation60]) in these sterilization conditions. The established conditions for continuous system assays were a fixed bed (LFB) of 1 cm (700 mg of nanocomposites or 300 mg of CFs) and a contact time with the material of 1 min (tC). To achieve these conditions, firstly the fixed bed volume (VFB) and the flow rate () were determined by equations (Equation1
(1)
(1) ) and (Equation2
(2)
(2) ), corresponding to 0.8 mL min−1. The volume of suspension to treat (VS) was determined by Equationequation (3
(3)
(3) ), wherein tOP is the total time of operation (180 min), and the obtained value was 144 mL. The continuous system () was operated inside a laminar flow cabinet (Class II Microbiological Safety Cabinet Bio II Advance Plus, Telstar®). Briefly, the feed suspension was maintained in a Schott flask and pumped (ISMATEC®) to the Omnifit® column reactor through PTFE tubes. The treated suspension was collected in another Schott flask.
(1)
(1)
(2)
(2)
(3)
(3)
2.5.1 Bacteria removal tests
The bacteria removal tests were performed with suspensions of 106 CFU mL−1, taking into account the standard limits for sterile pharmaceutical conditions [Citation61] and maintaining the same conditions from previous works [Citation48, Citation54]. The chosen bacteria E. coli and S. aureus are associated with medical-infections [Citation62] and are Gram-negative bacilli and Gram-positive cocci, respectively, which permitted to evaluate the influence of the cell morphology and/or cell wall structure on the performance of the tested materials. Suspensions of the strains E. coli DSM 1103 and S. aureus DSM 1104 were used individually or in mixture as reported by Santos et al. [Citation54]. Briefly, stock cultures of E. coli and S. aureus were grown on Plate Count Agar (PCA) medium (VWR Chemicals®) at 30°C for 20 h and 37°C for 24 h, respectively. Then, suspensions were prepared at a density of 108 CFU mL−1 (obtained by measurements of optical density of, approximately, 0.1 at 610 nm for E. coli and 0.13 at 600 nm for S. aureus) followed by serial dilutions to obtain suspensions of 106 CFU mL−1. Assays were performed using axenic or mixed suspensions of these bacterial strains in sterile saline solution (0.85% NaCl, w v−1) alone or supplemented with 25 mg L−1 Cd2+ and 25 mg L−1 Pb2+, which precursors (CdCl2 and Pb(NO3)2, respectively) were filter sterilized and added after autoclaving the saline solution to avoid possible metals precipitation reactions [Citation63, Citation64].
Each suspension was used to feed the Omnifit® column reactor, as described above. Samples were taken from the feed suspension immediately before starting the flow and from the treated effluent every 30 min up to 180 min. The first sample of the treated effluent (t = 0) was collected 3 min after starting the flow.
To enumerate the cultivable bacteria, samples of the feed suspension and from the treated effluent were serially diluted and 100 µL of the dilutions 10−1, 10−2, 10−3 and 10−4 were spread, in duplicate, on PCA medium. After incubation, the colony-forming units (CFU) were enumerated in plates containing between 30 and 300 CFU. Control samples, collected from a Omnifit® column reactor without fixed bed (tested materials), were processed in parallel using the same methodology. The three materials that exhibited better efficiencies for bacteria removal were tested in triplicate (N = 3).
To assess the performance of the materials over longer operation periods, a second assay was performed for 9 h, maintaining the contact time of 1 min. In this assay, the enumeration of the cultivable bacteria in the treated effluent was performed in samples collected every 3 h. In addition, the residual treated suspension (210 mL in duplicate) was filtered through a cellulose nitrate membrane (0.22 µm porosity, Whatman) for bacteria retention, which was incubated on the surface of a PCA plate (see Online Resource Figure S2).
The performance of each studied material in bacteria removal was evaluated using Equation (4), which permitted to measure the log reduction.
(4)
(4)
2.5.2 Metal leaching
To guarantee the absence of leached metals from nanocomposites, samples at 30, 120 and 180 min of treatment, in continuous system, were collected. ICP technique was used to determine the presence of leached ions (Fe2+, Fe3+, Cu2+ and Ag+) from nanocomposites. The obtained results were discussed taking into account the legal limits of each metal: 2.0 mg L−1 for Cu2+;0.1 mg L−1 for Ag+ and 2.0 mg L−1 for Fe ions [Citation4].
3. Results and discussion
3.1 Materials characterization
The textural properties and carbon and metal amounts measured by TGA are present in . The support AC demonstrated a high specific surface area of 934 m2 g−1, but all its textural parameters decreased with the incorporation of the MNPs, as expected. For example, the nanocomposite FeO/AC presented a specific surface area of 371 m2 g−1 due to the deposition and obstruction of AC pores (a decrease of 0.473 cm3 g−1–0.322 cm3 g−1) by iron oxide, which contributed to a low adsorption of N2 but an increase of SMESO because iron oxide can provide some porosity [Citation65]. Similar results were obtained for the nanocomposites 5% AgO/AC and 5% CuO/AC, but with small decreases of SBET, 901 m2 g−1 and 822, respectively, due to the low amounts of MNPs on these materials when compared with the previous nanocomposite.
Table 1. Textural properties and mass fractions of carbon (C) and metal (M) of the studied materials.
However, a difference between the SBET of 5% AgO/AC and 5% CuO/AC with the same amount of metal was noted and can be attributed to the different nanostructure of each metal [Citation66, Citation67]. Differences in textural properties between calcined and non-calcined nanocomposites were verified: CuFeO/AC-C and FeO/AC-C showed a higher SBET (338 m2 g−1 and 420 m2 g−1, respectively) than the non-calcined samples (309 m2 g−1 and 371 m2 g−1, respectively). The effect of calcination is correlated with heterojunctions phenomena between metals and AC [Citation67, Citation68], and the elimination of precursor molecules that were blocking AC pores. The nanocomposite 5% AgO/FeO/AC demonstrated a slight decrease of SBET (346 m2 g−1) compared with FeO/AC (371 m2 g−1) due to the silver impregnation. The lowest superficial area was achieved by CuFeO/AC@N due to the N doping of AC, which contributed to a decrease of the surface area of AC (AC with 934 m2 g−1 and AC@N with 761 m2 g−1) and the co-precipitation of MNPs in the AC@N support. The commercial materials CF-ACF and CF-SOFT presented the highest superficial areas, while CF-CAS showed 281 m2 g−1 probably, due to the casein impregnation.
The mass fractions of carbon and metal determined by TGA achieved the expected values for the prepared the elimination of some molecules of the metal precursors because in the TGA profiles (see Online Resource, Figure S3) of FeO/AC-C and CuFeO/AC-C were observed lower mass losses. However, the nanocomposites with iron oxide demonstrated an increase of mass in the burning phase with air, probably due to some incorporation of oxygen in the inorganic fraction.
The commercial CFs showed different thermostabilities, wherein CF-CAS was the most unstable, probably due to the degradation of casein molecular groups, which presents low thermostability in high temperatures [Citation60]. TPR profiles are represented in , where the different reduction states of iron and copper can be observed.
For the nanocomposites FeO/AC, CuFeO/AC, CuFeO/AC@N and 5% AgO/FeO/AC the first reduction state of Fe3O4 occurred between 300 and 400°C. For these nanocomposites, a second peak occurred between 400 and 700°C, corresponding to the sequential reduction of Fe3O4 to FeO followed to Fe0 [Citation69]. The TPR profiles of nanocomposites with copper oxide showed a peak between 200°C and 350°C, coupled to the peak of the first reduction state of Fe3O4, corresponding to the reduction of CuO to Cu0 [Citation70].
Also, a higher H2 consumption was noted in the metal reductions of CuFeO/AC than 5% CuO/AC due to the higher metal amount (confirmed in TGA analysis) and higher than CuFeO/AC@N because AC@N had fewer free oxygen functional groups than AC (see EA analysis, ) that can react with H2. For the nanocomposites with silver, the reduction peak of Ag2O to Ag0 was not observed due to the low H2 chemisorption of this metal [Citation71]. However, in the TPR profile of 5% AgO/FeO/AC, the reduction peaks of iron oxide were identified but at slightly lower temperatures (between 200 and 450°C) due to the presence of silver [Citation72]. Elemental analysis was performed for commercial CFs, original AC, and the N-doped AC. The results, shown in , demonstrated that commercial CFs had high amounts of oxygen. CF-CAS presented a higher amount of nitrogen and hydrogen than the other CFs due to the presence of casein.
Table 2. Elemental analysis of the commercial and activated carbon-based materials.
AC showed a significant amount of oxygen groups in its composition, and the N-doping of AC provided an increase of nitrogen amount of approximately 3 wt.%.
The crystalline phases of the studied nanocomposites identified by XRD are presented in . Different phases were expected to be observed in the three nanocomposites with iron oxide, such as magnetite (Fe3O4) and maghemite (γ-Fe2O3) with cubic crystal structure, which are difficult to distinguish in XRD pattern due to the similar crystal structure, hematite (α-Fe2O3) with rhombohedral crystal structure and goethite (α-FeO(OH)) whose crystal structure consists of double chains of edge-shared octahedra that are joined to other double chains by sharing corners and hydrogen bonds [Citation73, Citation74]. XRD analysis identified the presence of magnetite (∼36°, ∼64°) in FeO/AC, CuFeO/AC and 5% AgO/FeO/AC, maghemite only in FeO/AC and goethite (∼35°) in FeO/AC and CuFeO/AC, which are in accordance with the literature for iron oxides AC composites [Citation9, Citation73]. In the nanocomposites with copper oxide, a tenorite crystal phase was identified at ∼38°, which is also in accordance with literature for composites with AC [Citation75]. The identified silver (Ag) phases were at 38o, 44o, 64o, 77.2° and 81.5° and silver chloride (AgCl) at 46.3°, 55.0° and 57.6° for 5% AgO/AC with diffraction peaks with higher intensity and for 5% AgO/FeO/AC without AgCl detection [Citation76]. This analysis also permitted to verify that silver particles were not in the oxide form, which could influence the heavy metal removal tests due to the absence of the needed negative charge provided by oxygen atoms [Citation32]. The identified diffraction peeks at 26–27° are characteristic of graphite, which was well seen in the nanocomposites with low amount of metals and higher amount of carbon (5% AgO and 5% CuO/AC) [Citation77]. It should be highlighted that graphite’s peaks revealed the presence of an amorphous structure, which is disorderly stacked up by carbon rings and helpful for producing well-defined adsorbent [Citation78].
3.2 Heavy metals removal in batch experiments – kinetics
The batch experiments permitted to evaluate the performance of the materials on Cd + 2 and Pb2+ adsorption, separately. The obtained results confirmed the promising properties of AC for heavy metals removal [Citation79]. As shown in , AC achieved a Pb2+ removal of approximately 45% after 2 min of contact time. For cadmium removal, AC demonstrated to be inefficient. However, with magnetic nanocomposites, it was possible to achieve better results than with AC and the non-magnetic nanocomposites (5% CuO/AC and 5% AgO/AC). The iron oxide increased the electrostatic and Van der Waal forces between heavy metals and MNPs supported on porous AC [Citation32, Citation80]. The nanocomposites with 5% of silver oxide showed lower adsorption properties than the corresponded ones without silver oxide, demonstrating that silver displayed the worst adsorption properties for lead and cadmium removal. The presence of copper oxide provided an enhancement of adsorption properties when comparing the nanocomposite CuFeO/AC with FeO/AC, in the range of 0–30 min of contact time, and 5% CuO/AC with 5% AgO/AC, wherein was obtained a slight enhancement of cadmium removal and a graduated increase over the contact time in lead removal. Preferred adsorption of lead instead of cadmium was noted, and according to similar studies, the same phenomenon was verified in cadmium and lead removal using granular AC. The reported studies explain that adsorption difference can be related to: (i) the nature of AC, i.e. the type of raw materials used to synthesize the AC provide different physical structure (porosity, surface area, etc.) which originates different capacities for heavy metals adsorption [Citation81, Citation82] and (ii) the pH of the solutions of each metal due to the competition of hydrogen ions with metal ions for sorption sites on the adsorbent [Citation57]. The material with the most promising capacity for heavy metals removal was CuFeO/AC with the highest removal values after 120 min of contact (58.1% for Cd2+ and 92.7% for Pb2+). These results were achieved with a very high concentration of each heavy metal in solution. Comparing these batch experiments with XRD analysis () the presence of magnetite/maghemite and goethite in FeO/AC, CuFeO/AC and 5% AgO/FeO/AC explains the better results on heavy metals removal for these nanocomposites. Moreover, iron oxide phases have a large surfacer area, contributing to their high adsorption capacity [Citation83]. Giraldo et al. [Citation84] synthesized magnetite nanoparticles using a co-precipitation method and the obtained nanoparticles were used to treat Pb2+ and other heavy metals in a batch mode, observing that nanosized magnetite had the best adsorption effect towards Pb2+ (0.180 mmol g−1). In this study, CuFeO/AC obtained the best performance in heavy metals removal due to the presence of the iron oxide crystal phases mentioned before, as well as the presence of tenorite with higher diffractions peaks (larger crystals), which all contributed to a synergetic effect for Pb2+ and Cd2+ adsorption. Also, XRD analysis confirmed the worst performance of 5% AgO/AC due to the absence of silver oxide form, as explained before.
3.3 Continuous system assays
3.3.1 E. coli removal
AC (without MNPs) was able to remove the bacterial cells per se, showing a log reduction close to 5 within the first moments of contact with the suspension (t0, (a)). Indeed, the macroporous structure of AC may have allowed the adsorption of bacteria due to its dimensions (≥100 nm) [Citation85]; however, over time, it contributed to the obstruction of AC’s porous, providing a decrease of the removal performance over time, reaching log reductions of 2.86 after 180 min ((a)).
Figure 5. E. coli removal tests in continuous system using (a) AC and magnetic nanocomposites (N = 1); (b) calcinated magnetic nanocomposites, and silver and copper nanocomposites (N = 1); (c) commercial CFs (N = 1) and (d) the most promising nanocomposites (N = 3). Error bars represent standard deviations. No removal was observed in the controls (data not shown).
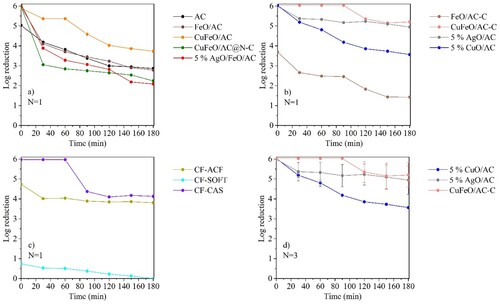
The incorporation of MNPs on AC provided an enhancement of the performance of the materials, which, excepting FeO/AC-C, achieved a 6 log reduction at t0 ((a,b)); however, FeO/AC achieved a similar performance of AC after 30 passage times, whereas CuFeO/AC and CuFeO/AC@N–C showed better and worse performance than AC, respectively. Such results indicate that N-doping of AC did not enhance the nanocomposite properties for this application. b presents the performance of the calcined nanocomposites and the metal-impregnated ones. Impregnation of AC with metals with bactericide properties (silver or copper) allowed to obtain nanocomposites capable of achieving log reductions above 3.5 after 180 min (4.94 ± 0.72 and 3.56 ± 0.03, for 5% AgO/AC and 5% CuO/AC, respectively, (d)). However, the nanocomposite with silver and iron oxide showed an opposite effect in E. coli removal, with lower removal performance than 5% AgO/AC and FeO/AC ((a)). Comparing the XRD analysis of 5% AgO/AC and 5% AgO/FeO/AC, it was noted that the diffraction peaks of silver crystals of 5% AgO/AC were larger than 5% AgO/FeO/AC and AgCl crystal phases in the first nanocomposite were also identified (see ). These differences may contribute to higher bactericide properties of 5% AgO/AC than 5% AgO/FeO/AC. Although 5% AgO/FeO/AC did not achieve promising results, an interesting phenomenon was observed in the E. coli cells that survived in the treatment with this nanocomposite. A distinct morphology of the formed CFU was observed and SEM analysis revealed that the cells surviving the 5% AgO/FeO/AC treatment were agglomerated and deformed, in contrast to those of control (). Hence, despite surviving, the contact with silver seems to have deleterious effects on the cellular structure of this organism. Indeed, Ag+ is described as a cellular membrane disruptor, which leads to cellular death [Citation13, Citation86, Citation87]. FeO/AC-C showed a worse performance on E. coli removal (1.4 log reduction after 180 min) than the FeO/AC (2.9 log reduction after 180 min). In contrast, calcination of CuFeO/AC improved the performance of the nanocomposite, achieving 6.0 ± 0.02 log reductions up to 90 passage times and 5.4 ± 0.6 log reduction after 120 min, which was maintained up to 180 min ((d)). These results demonstrated that the calcination step was crucial for the enhancement of bacteria removal properties because it permitted a better attachment of metals and unblocking some pores of the nanocomposite (see ). Also, the type of metals present in CuFeO/AC-C played an important role. Comparing FeO/AC (69% of inorganic matter, TGA) and 5% CuO/AC (7% of inorganic matter, TGA), copper oxide demonstrated higher bactericide properties than iron oxide, even in low amounts.
Figure 6. SEM images of E. coli cells in the control sample and after contact with 5% AgO/FeO/AC nanocomposite.
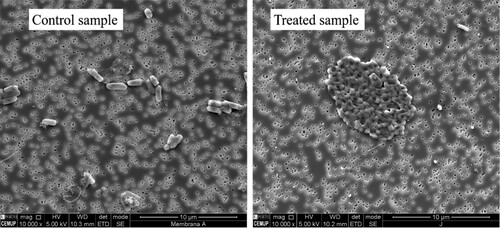
Among the tested CFs ((c)) CF-CAS showed the best performance, reaching a 6 log reduction during 60 passage times. After that period, the performance of CF-CAS decreased with ∼4 log reduction up to 180 min operation, probably due to the saturation of this material that had low surface area and porosity (see ) bactericide activity of casein [Citation43–45]. According to the literature, the antimicrobial property of casein may rely upon the formation of amphipathic structures, which disturb the cell membrane permeability, allowing the leakage of ions and other materials from the cell [Citation28, Citation44]. CF-ACF showed a worse performance than CF-CAS, although with a stable behaviour of removal (∼3.9 log reduction between 30 and 180 min), suggesting an adsorption mechanism for E. coli removal due to its high specific surface area (1905 m2 g−1, see ). CF-SFOT achieved the worst performance in E. coli removal. Despite having a higher specific surface area than AC (see , CF-SOFT), the amount of oxygen groups in CF-SFOT is considerable high (see ), compared with the other materials. Following the reported information, a high amount of oxygen groups on the surface of the material can result in a high negative charge which acts as a repellent to the negative charge of bacteria surface structures [Citation85]; for this reason, the low capacity of CF-SOFT to remove E. coli from water could be attributed to the high amount of oxygen groups. In addition, it may also be influenced by the physical structure of the fibre (felt), which is different from CF-ACF (cloth) and by the absence of a bactericide component as CF-CAS does.
According to the results described above, the three most promising synthesized nanomaterials for water disinfection were 5% AgO/AC, 5% CuO/AC and CuFeO/AC-C, which were selected for further studies.
3.3.2 S. aureus removal
S. aureus removal assays were performed in the presence of the three materials that showed promising results in E. coli removal, i.e. 5% AgO/AC, 5% CuO/AC and CuFeO/AC-C in three independent assays (N = 3). shows the obtained results and permitted to conclude that silver oxide (5% AgO/AC) had a worse performance for S. aureus removal than for E. coli (at 180 min, 4.0 ± 0.09 and 4.9 ± 0.7 log reduction, respectively). The morphologic difference between S. aureus (Gram-positive cocci) and E. coli (Gram-negative bacilli) may have contributed to these results, wherein S. aureus with a thicker peptidoglycan layer and one less outer membrane than E. coli [Citation88], may better tolerate the silver oxide particles. However, in the presence of copper oxide (5% CuO/AC and CuFeO/AC-C) the obtained results demonstrated an opposite effect since a complete removal of S. aureus occurred all over time. Such results indicate that copper-containing MNPs had better S. aureus removal capacity than the silver ones.
Figure 7. S. aureus removal tests in continuous system (N = 3 assays). Error bars represent standard deviations.
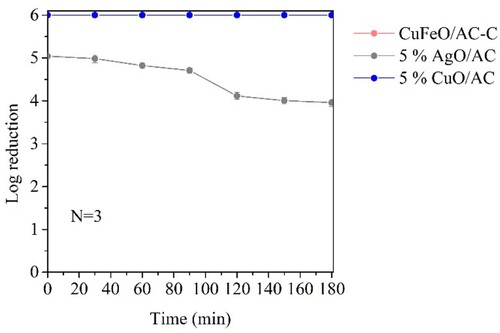
Comparing the obtained results of E. coli and S. aureus removal with the legal admissible limits of log reduction occurring during disinfection [Citation89], we may conclude that all the three most promising materials were efficient (see ), with CuFeO/AC-C achieving the best performance.
Table 3. Log reduction of 106 CFU mL−1 E. coli and 106 CFU mL−1 S. aureus, separately, after 180 min using the three most promising nanocomposites and respective legal limits by WHO [Citation78].
According to the TGA characterization, CuFeO/AC-C showed the highest amount of metal (75%, see ). The antimicrobial activity of copper is well described in the literature [Citation90, Citation91]. Therefore, the high amount of copper in CuFeO/AC-C may have contributed to the obtained results.
3.3.3 Simultaneous removal of E. coli and S. aureus
The bacteria removal performance of CuFeO/AC-C was also evaluated by mixing the tested bacteria in the same suspension (maintaining the final density of 106 CFU mL−1). Complete removal of E. coli and S. aureus was achieved with CuFeO/AC-C with a 6 log reduction all over 180 min ((a)). Slight variations in the experimental set-up (e.g. exact number of initial cells, mass of nanocomposite in the fixed bed) may explain the differences found between this assay and the one carried out with axenic suspensions of E. coli (∼ 5 log reduction after 120 min up to 180 min).
Figure 8. Bacteria removal test of a mixture of 5 × 105 CFU mL−1 of E. coli and 5 × 105 CFU mL−1 of S. aureus in continuous system using CuFeO/AC-C opering for (a) 180 min; (b) 540 min. N = 1 assay.
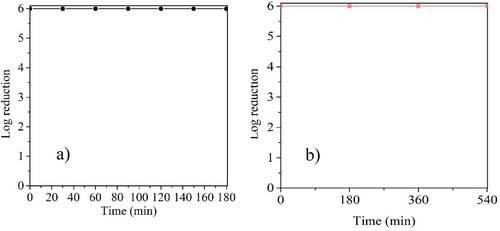
Due to the promising results of CuFeO/AC-C in bacteria removal during 180 min, an assay with 540 passage times was performed to assess the stability of CuFeO/AC-C ((b)) During all the assay, a 6 log reduction was achieved and no colonies were observed in the cultures of the residual treated suspension (see Online Resource, Figure S4), demonstrating the stability of the CuFeO/AC-C nanocomposite.
3.3.4 Heavy metals removal
The performance of CuFeO/AC-C was evaluated for Cd2+ and Pb2+ removal in a continuous system and with a mixture of both metals. In this assay, CuFeO/AC-C instead of CuFeO/AC (tested in batch systems) was applied due to the promising results of CuFeO/AC-C in bacteria removal and to establish a comparison between heavy metals and bacteria removal. Also, it must be noted that the conditions used in the continuous assay (mass of CuFeO/AC-C and solution volume) were carried out with different ratios: 1:5 (144 mL for 700 mg) in continuous system, and 1:1 (20 mL for 20 mg in batch reaction). reveals that CuFeO/AC-C presented better results during heavy metals removal (at 120 min 99.9% of Pb2+ removal and 94.6% of Cd2+ removal) under continuous conditions than CuFeO/AC in batch reaction (at 120 min 92.7% of Pb2+ removal and 58.1% of Cd2+ removal), proving that a 1:5 ratio of material and solution volume enhances the adsorption of both metals even they being in the same solution with a total concentration of 50 mg L−1. Also, CuFeO/AC-C provided better results than CuFeO/AC due to the better textural properties, which provided better adsorption (). These removal results did not achieve the legal limits admissible for each heavy metal (at 120 min 0.025 mg L−1 of Pb2+ and 1.35 mg L−1 of Cd2+). However, it must be highlighted that in this assay, a very high concentration of heavy metals (50 mg L−1) was used, which contributed to an inefficient total adsorption due to the possible saturation of CuFeO/AC-C adsorption sites, which provided a loss of adsorption capacity over the time, mostly verified for cadmium removal. Comparing the results obtained in bacteria removal () and heavy metals removal () it is possible to verify that CuFeO/AC-C achieved better performance for bacteria than for heavy metals.
3.3.5 Bacteria and heavy metals removal
A mixture containing bacteria and heavy metals was prepared, maintaining the concentrations of each contaminant equal to the previous assays, i.e. 106 CFU mL−1 of bacteria (∼ 5 × 105 CFU mL−1 each) and 25 mg L−1 of each heavy metal. Under these conditions, a slightly lower log reduction value (5.82) was obtained immediately at the beginning of the assay and was kept for 180 min. (). This decrease was also observed in the control sample (data not shown), which suggests that the bacterial density decreased during the preparation of the test mixture. The hypothesis behind this decrease was the presence of high concentrations of toxic heavy metals in the medium that can provide some cellular death [Citation92]. Comparing the performance of CuFeO/AC-C for bacteria removal and heavy metals removal, better results for bacteria removal (5.82 log reduction in all passage times) than heavy metals were obtained. Indeed, a decrease in the adsorption of Cd2+ and Pb2+ after 60 min occurred (). Also, a higher decrease in the removal efficiency of each heavy metal occurred in this assay (at 180 min 56.3% for Cd2+ and 98.3% for Pb2+) than without bacteria but with both heavy metals in solution (at 180 min 86.1% for Cd2+ and 99.9 for Pb2+). This suggests that bacteria removal by CuFeO/AC-C involves an adsorption step, which blocks the porosity of the material and the access of metals oxides, hindering the adsorption of the heavy metals. Cadmium removal, once again, demonstrated to be more difficult to be removed than lead, which evidenced the heavy metals adsorption selectivity. However, in this assay, the presence of bacteria in the same mixture may have also affected the Cadmium adsorption as it is reported that ROS species provided by CuFeO/AC-C in contact with bacteria can react with Cd2+ forming Cd(OH)2 [Citation92].
3.5. Simulation of a real water sample
To access the removal of Cd2+ and Pb2+ at concentrations detected in real water samples (0.10 mg L−1), CuFeO/AC-C was tested in batch (for each metal, separately) and in the continuous system with both metals simultaneously (). These assays confirmed that the mechanism of Cd2+ removal is different from Pb2+, since the first times of contact with CuFeO/AC-C were sufficient to remove Pb2+ (>99.9%). After 180 min, the concentration of Pb2+ was 1.0 × 10−5 mg L−1 and of Cd2+ was 3.7 × 10−4 mg L-1. With these results, CuFeO/AC demonstrated to be a promising material capable of removing Cd2 + and Pb2+ to values matching the legally admissible limits [Citation4].
Figure 11. Kinetic experiments of CuFeO/AC-C in batch at (a) 0.10 mg L−1 Cd2+ and (b) 0.10 mg L−1 Pb2+ and (c) in continuous system with 0.10 mg L−1 Cd2+ 0.10 mg L−1 Pb2+.
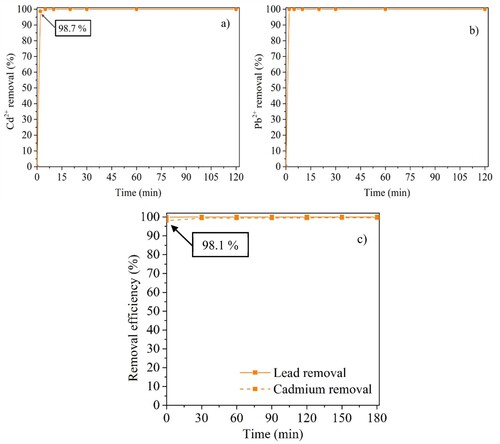
Considering the promising performance of CuFeO/AC-C in heavy metals removal at real water concentrations, another assay with a mix of bacteria and heavy metals, simulating real water, was carried out (). The obtained results showed a complete bacteria removal, i.e. 6 log reduction, which indicated that 0.10 mg L-1 of Pb2+ and Cd2+ was a non-toxic concentration for E. coli and S. aureus due to the non-observed decrease of cellular density of the control sample (data not shown). Lead was practically removed (>99.9%) and cadmium showed a slight decrease of removal (between 97 and 98%) after 90 passage times. However, when compared with the performed assay with higher heavy metals concentration (), CuFeO/AC-C demonstrated a better performance in the water treatment with these lower concentrations of heavy metals. In the Figure S5 of the Online Resource is presented the suggested mechanism for bacteria and heavy metals removal in the experimental conditions of this study.
Figure 12. Bacteria and heavy metals removal using CuFeO/AC-C in continuous system assay (5 × 105 CFU mL−1 of E. coli, 5 × 105 CFU mL−1 of S. aureus, 0.10 mg L−1of Cd2 + and 0.10 mg L−1of Pb2+). (N = 1).
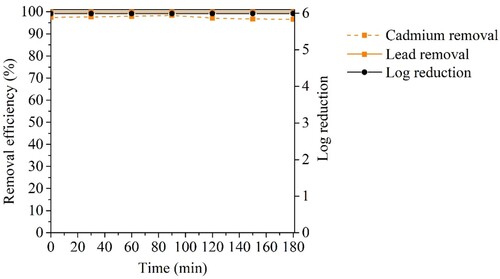
compares the efficiency of the materials analysed in this study with others reported in the literature for bacteria and/or heavy metals removal (separated into three groups: bacteria, heavy metals, and both in removal tests). Like the present study, most of the data summarized in was obtained with solutions/suspensions simulating real conditions, corresponding to preliminary research works. Comparing the performance of CuFeO/AC-C with the listed reported materials, it is possible to conclude that this material is a promising adsorbent to be used in water treatment technology. However, practical applications and simulating industrial wastewater treatment are still lacking.
Table 4. Comparison of the efficiency of the materials of the present study with others reported in the literature for for E. coli, S. aureus, Cd2+ and Pb2+ separated and/or simultaneous removal.
3.6 Metal nanoparticles leaching detection
Even though nanocomposites can achieve promising results in bacteria and heavy metals removal, their use in water treatment processes must guarantee the water quality after treatment, i.e. without metal leaching since these metals are harmful not only to bacteria but also to other living beings, including humans [Citation93]. Another important fact is that leaching metals from nanocomposites disable their performance for re-utilization. To identify leaching phenomena, ICP analysis of collected samples (at 30, 120 and 180 min) in continuous assays was carried out, and the obtained results for each tested nanocomposite are summarized in Table S1, wherein for each metal are the corresponding legal admissible limit [Citation4]. The results suggest that all nanocomposites were stable after the three successive operation times. In terms of leaching concentrations, CuFeO/AC-C showed low values of Fe2+, not exceeding the legal limit and no leaching of Cu2+. The 5% AgO/AC sample showed concentrations of Ag+ higher than the admissible legal limit and 5% CuO/AC revealed no Cu2+ leaching. These results suggest that CuFeO/AC-C and 5% CuO/AC are passible to be used in water treatment; however, 5% AgO/AC is not indicated due to the leaching concentration of Ag+ above the legal limit, which could provide ecotoxicity and be harmful to human health [Citation94, Citation95].
Comparing CuFeO/AC-C with similar materials tested in previous studies using CNT as a support or only MNPs [Citation48, Citation54], this one presents advantages to be applied in a water treatment technology due to be less expensive, and the type of process tested is simple and effective to be employed in undeveloped countries.
4. Conclusions
This study had the purpose of preparing nanocomposites with bactericide properties to remove two different bacteria (E. coli Gram-negative and S. aureus Gram-positive) and two heavy metals with high toxicity for humans – cadmium and lead. The performance of the synthetized nanocomposites was compared to that of commercial carbon fibres. The heavy metals removal batch assays permitted to access the most promising material for this purpose – CuFeO/AC – with 58.1% and 92.7% of removal of 25 mg L-1 of Cd2+ and Pb2+, respectively, after 120 min of contact time. The bacteria removal tests in a continuous system permitted to conclude that AC nanocomposites containing metals and a calcination treatment showed enhanced removal efficiency. CuFeO/AC-C was the best material for E. coli and S. aureus removal, separately, achieving a 5/6 log reduction after 180 passage times and with a better performance than commercial CFs. This nanocomposite allowed the simultaneous removal of E. coli and S. aureus up to 540 passage times, demonstrating stability and accomplishing the legal requirements. CuFeO/AC-C was also able to remove both Cd2+ and Pb2+ from aqueous solutions with heavy metals at real water concentrations and bacteria, simulating real water; a 6 log reduction of bacteria and Pb2+ removal of >99.9% and Cd2+ removal between 97-98% over 180 passage times were observed. The metal leaching analysis also proved that CuFeO/AC-C was a stable nanocomposite without leaching metal nanoparticles at concentrations below the legally admissible limits.
Author contributions
Conceptualization, O.S.G.P.S., O.C.N. and A.G.G; methodology, O.S.G.P.S., O.C.N., A.S.G.G.S. and A.T.V.; investigation, J.R.M.B.; resources, O.S.G.P.S., M.F.R.P. and O.C.N.; writing – original draft preparation, J.R.M.B.; writing – review and editing, J.R.M.B., O.S.G.P.S., O.C.N and M.F.R.P.; supervision, O.S.G.P.S., O.C.N. and M.F.R.P.; funding acquisition, O.S.G.P.S., O.C.N. and M.F.R.P. . All authors have read and agreed to the published version of the manuscript.
Supplementary_information.docx
Download MS Word (1.5 MB)Disclosure statement
No potential conflict of interest was reported by the author(s).
Data availability statement
The data that support the findings of this study are available from the corresponding author, [OSGPS], upon request.
Additional information
Funding
References
- Abdullah N, Shameli K, Ezzat Abdullah EC, et al. Solid matrices for fabrication of magnetic iron oxide nanocomposites: synthesis, properties, and application for the adsorption of heavy metal ions and dyes. Compos B: Eng. 2019;162:538–568. doi:10.1016/j.compositesb.2018.12.075
- Barnett T, Adam J, Lettenmaier D. Potential impacts of a warming climate on water availability in snow-dominated regions. Nature. 2005;438(7066):303–309. doi:10.1038/nature04141
- Ridoutt B, Pfister S. A revised approach to water footprinting to make transparent the impacts of consumption and production on global freshwater scarcity. Glob Environ Change. 2010;20(1):113–120. doi:10.1016/j.gloenvcha.2009.08.003
- World Health Organization. (2011). Guidelines for drinking-water quality (Vol. 1). Geneva: WHO Press.
- Rao RAK, Khan MA. Biosorption of bivalent metal ions from aqueous solution by an agricultural waste: kinetics: thermodynamics and environmental effects. Colloids Surf, A. 2009;332(2-3):121–128. doi:10.1016/j.colsurfa.2008.09.005
- Teoh YP, Khan MA, Choong TSY. Kinetic and isotherm studies for lead adsorption from aqueous phase on carbon coated monolith. Chem Eng J. 2013;217:248–255. doi:10.1016/j.cej.2012.12.013
- Elimelech M, Montgomery M. (2007). Water and sanitation in developing countries: including health in the equation.
- Serafini M, Almeida A, Junior A, et al. Avaliação de tecnologias de dessalinização de água a partir da análise dos pedidos de patentes. Geintec. 2012;2(1):42–51. doi:10.7198/S2237-07222012000100005
- Castro CS, Guerreiro MC, Gonçalves M, et al. Activated carbon/iron oxide composites for the removal of atrazine from aqueous medium. J Hazard Mater. 2009;164(2-3):609–614. doi:10.1016/j.jhazmat.2008.08.066
- Dinh NX, Chi DT, Lan NT, et al. Water-dispersible silver nanoparticles-decorated carbon nanomaterials: synthesis and enhanced antibacterial activity. Appl Phys A. 2015;119(1):85–95. doi:10.1007/s00339-014-8962-6
- Lofrano G, Carotenuto M, Libralato G, et al. Polymer functionalized nanocomposites for metals removal from water and wastewater: an overview. Water Res. 2016;92:22–37. doi:10.1016/j.watres.2016.01.033
- Raghunath A, Perumal E. Metal oxide nanoparticles as antimicrobial agents: a promise for the future. Int J Antimicrob Agents. 2017;49(2):137–152. doi:10.1016/j.ijantimicag.2016.11.011
- Zheng K, Setyawati MI, Leong DT, et al. Antimicrobial silver nanomaterials. Coord Chem Rev. 2018;357:1–17. doi:10.1016/j.ccr.2017.11.019
- Chung Y, Su Y, Chen C, et al. Relationship between antibacterial activity of chitosan and surface characteristics of cell wall. Acta Pharmacol Sin. 2004;25(7):932–936.
- Liu Y, He L, Mustapha A, et al. Antibacterial activities of zinc oxide nanoparticles against Escherichia coli O157:H7. J Appl Microbiol. 2009;107(4):1193–1201. doi:10.1111/j.1365-2672.2009.04303.x
- Rezaei-Zarchi S, Javed A, Ghani MJ, et al. Comparative study of antimicrobial activities of TiO2 and CdO nanoparticles against the pathogenic strain of Escherichia coli. Iran J Pathol. 2010;5(2):83–89.
- Nel A, Xia T, Mädler L, et al. Toxic potential of materials at the nanolevel. Science. 2006;311(5761):622–627. doi:10.1126/science.1114397
- Hajipour MJ, Fromm KM, Akbar Ashkarran A, et al. Antibacterial properties of nanoparticles. Trends Biotechnol. 2012;30(10):499–511. doi:10.1016/j.tibtech.2012.06.004
- Kohanski MA, Dwyer DJ, Hayete B, et al. A common mechanism of cellular death induced by bactericidal antibiotics. Cell. 2007;130(5):797–810. doi:10.1016/j.cell.2007.06.049
- Kumar SR, Imlay JA. How escherichia coli tolerates profuse hydrogen peroxide formation by a catabolic pathway. J Bacteriol. 2013;195(20):4569–4579. doi:10.1128/JB.00737-13
- Ghasemzadeh G, Momenpour M, Omidi F, et al. Applications of nanomaterials in water treatment and environmental remediation. Front Environ Sci Eng. 2014;8(4):471–482. doi:10.1007/s11783-014-0654-0
- Hlongwane GN, Sekoai PT, Meyyappan M, et al. Simultaneous removal of pollutants from water using nanoparticles: a shift from single pollutant control to multiple pollutant control. Sci Total Environ. 2019;656:808–833. doi:10.1016/j.scitotenv.2018.11.257
- Jin Y, Liu F, Shan C, et al. Efficient bacterial capture with amino acid modified magnetic nanoparticles. Water Res. 2014;50:124–134. doi:10.1016/j.watres.2013.11.045
- Hatchett DW, White HS. Electrochemistry of sulfur adlayers on the low-index faces of silver. J Phys Chem. 1996;100(23):9854–9859. doi:10.1021/jp953757z
- Kirstein J, Turgay K. A New tyrosine phosphorylation mechanism involved in signal transduction in bacillus subtilis. Microbial Physiology. 2005;9(3-4):182–188. doi:10.1159/000089646
- Shrivastava S, Bera T, Roy A, et al. Characterization of enhanced antibacterial effects of novel silver nanoparticles. Nanotechnology. 2007;18(22). doi:10.1088/0957-4484/18/22/225103
- Dua V, Surwade SP, Ammu S, et al. All-Organic vapor sensor using inkjet-printed reduced graphene oxide. Angew Chem, Int Ed. 2010;49(12):2154–2157. doi:10.1002/anie.200905089
- Epand RM, Vogel HJ. Diversity of antimicrobial peptides and their mechanisms of action. Biochim Biophys Acta (BBA) - Biomembr. 1999;1462(1-2):11–28. doi:10.1016/S0005-2736(99)00198-4
- Bhatnagar A, Hogland W, Marques M, et al. An overview of the modification methods of activated carbon for its water treatment applications. Chem Eng J. 2013;219:499–511. doi:10.1016/j.cej.2012.12.038
- Shim J-W, Park S-J, Ryu S-K. Effect of modification with HNO3 and NaOH on metal adsorption by pitch-based activated carbon fibers. Carbon N Y. 2001;39(11):1635–1642. doi:10.1016/S0008-6223(00)00290-6
- Chen C, Wang X. Adsorption of Ni(II) from aqueous solution using oxidized multiwall carbon nanotubes. Ind Eng Chem Res. 2006;45(26):9144–9149. doi:10.1021/ie060791z
- Mohammed L, Gomaa HG, Ragab D, et al. Magnetic nanoparticles for environmental and biomedical applications: a review. Particuology. 2017;30:1–14. doi:10.1016/j.partic.2016.06.001
- Gupta AK, Gupta M. Synthesis and surface engineering of iron oxide nanoparticles for biomedical applications. Biomaterials. 2005;26(18):3995–4021. doi:10.1016/j.biomaterials.2004.10.012
- Ambashta RD, Sillanpaa M. Water purification using magnetic assistance: a review. J Hazard Mater. 2010;180(1-3):38–49. doi:10.1016/j.jhazmat.2010.04.105
- Park S, Park HH, Ko YS, et al. Disinfection of various bacterial pathogens using novel silver nanoparticle-decorated magnetic hybrid colloids. Sci Total Environ. 2017;609:289–296. doi:10.1016/j.scitotenv.2017.07.071
- Abdolmaleki A, Mallakpour S, Mahmoudian M, et al. A new polyamide adjusted triazinyl-β-cyclodextrin side group embedded magnetic nanoparticles for bacterial capture. Chem Eng J. 2017;309:321–329. doi:10.1016/j.cej.2016.10.063
- Khan MA, Alqadami AA, Otero M, et al. Heteroatom-doped magnetic hydrochar to remove post-transition and transition metals from water: synthesis: characterization, and adsorption studies. Chemosphere. 2019;218:1089–1099. doi:10.1016/j.chemosphere.2018.11.210
- Kell A, Stewart G, Ryan S, et al. Vancomycin-modified nanoparticles for efficient targeting and preconcentrationof gram-positive and gram-negative bacteria. ACS Nano. 2008;2(9):1777–1788. doi:10.1021/nn700183g
- Huang Y-F, Wang Y-F, Yan X-P. Amine-functionalized magnetic nanoparticles for rapid capture and removal of bacterial pathogens. Environ Sci Technol. 2010;44(20):7908–7913. doi:10.1021/es102285n
- Suzuki M. Activated carbon fiber: fundamentals and applications. Carbon N Y. 1994;32(4):577–586. doi:10.1016/0008-6223(94)90075-2
- Brasquet C, Cloirec P. Adsorption onto activated carbon fibers: application to water and air treatments. Carbon N Y. 1997;35(9):1307–1313. doi:10.1016/S0008-6223(97)00079-1
- Frank E, Ingildeev D, Buchmeiser MR. (2017). High-performance PAN-based carbon fibers and their performance requirements. 7-30.
- Expósito I, Minervini F, Amigo L, et al. Identification of antibacterial peptides from bovine k-casein. J Food Prot. 2006;69(12):2992–2997. doi:10.4315/0362-028X-69.12.2992
- McCann KB, Shiell BJ, Michalski WP, et al. Isolation and characterisation of a novel antibacterial peptide from bovine αS1-casein. Int Dairy J. 2006;16(4):316–323. doi:10.1016/j.idairyj.2005.05.005
- Wang Y, Ma J, Xu Q, et al. Fabrication of antibacterial casein-based ZnO nanocomposite for flexible coatings. Mater Des. 2017;113:240–245. doi:10.1016/j.matdes.2016.09.082
- Belkhir K, Pillon C, Cayla A, et al. Antibacterial textile based on hydrolyzed milk casein. Materials (Basel). 2021;14(2):251. https://www.mdpi.com/1996-1944/14/2/251. doi:10.3390/ma14020251
- Pereira L, Dias P, Soares OSGP, et al. Synthesis: characterization and application of magnetic carbon materials as electron shuttles for the biological and chemical reduction of the azo dye Acid Orange 10. Appl Catal, B. 2017;212:175–184. doi:10.1016/j.apcatb.2017.04.060
- Pinto M, Ramalho PSF, Moreira NFF, et al. Application of magnetic nanoparticles for water purification. Environ Adv. 2020;2:100010. doi:10.1016/j.envadv.2020.100010
- Serp, P., & Figueiredo, J. Surface chemistry of carbon materials. In: P. Serp, J. Figueiredo, editors. Carbon materials for catalysis. New Jersey & Canada: John Wiley & Sons, Inc; 2009. p. 45–92.
- Sousa J, Pereira M, Figueiredo J. Catalytic oxidation of NO to NO 2 on N-doped activated carbons. Catal Today. 2011;176(1):383–387. doi:10.1016/j.cattod.2010.11.040
- Jones N, Ray B, Ranjit KT, et al. Antibacterial activity of ZnO nanoparticle suspensions on a broad spectrum of microorganisms. FEMS Microbiol Lett. 2008;279(1):71–76. doi:10.1111/j.1574-6968.2007.01012.x
- Khezerlou A, Alizadeh-Sani M, Azizi-Lalabadi M, et al. Nanoparticles and their antimicrobial properties against pathogens including bacteria: fungi, parasites and viruses. Microb Pathog. 2018;123:505–526. doi:10.1016/j.micpath.2018.08.008
- Webster TJ, Seil I. Antimicrobial applications of nanotechnology: methods and literature. Int J Nanomed. 2012;2767. doi:10.1533/9780857096449
- Santos ASGG, Ramalho PSF, Viana AT, et al. Feasibility of using magnetic nanoparticles in water disinfection. J Environ Manag. 2021;288:112410. doi:10.1016/j.jenvman.2021.112410
- Lowell, S., Shields, J. E., Thomas, M. A., etal Surface area analysis from the langmuir and BET theories. In: S. Lowell, J. Shields, M Thomas, editors. Characterization of porous solids and powders: surface area, pore size and density. Dordrecht: Springer; 2004b. Vol. 16, p. 58–81.
- Lowell, S., Shields, J. E., Thomas, M. A., etal Micropore analysis. In: S. Lowell, J. Shields, M. Thomas, editors. Characterization of porous soldis and powders: surface area, pore and density. Dordrecht: Springer; 2004a. Vol. 16, p. 129–156.
- Jafari Kang A, Baghdadi M, Pardakhti A. Removal of cadmium and lead form aqueous solutions by acid-treated activated carbon nanocomposite. Desalin Water Treat. 2015;57(40):18782–18798. doi:10.1080/19443994.2015.1095123
- Word Health Organization. Cadmium in drinking-water. In: M. Sheffer, editor. 4th (Ed.), Guidelines for drinking-water quality. Geneva: WHO Press; 2011a. p. 5–6.
- Word Health Organization. Lead in driking-water. In: M. Sheffer, editor. 4th (Ed.), Guidelines for drinking-water quality. Geneva: WHO Press; 2011b. p. 10.
- Qi P, Wickham E, Farrell H. Thermal and alkaline denaturation of bovine beta-casein. The Protein Jounal. 2004;23(6):389–402. doi:10.1023/B:JOPC.0000039553.66233.3f
- Allison DG. A review: taking the sterile out of sterility. J Appl Microbiol. 1999;87(6):789–793. doi:10.1046/j.1365-2672.1999.00948.x
- De Faria AF, Martinez DST, Meira SMM, et al. Anti-adhesion and antibacterial activity of silver nanoparticles supported on graphene oxide sheets. Colloids Surf B. 2014;113:115–124. doi:10.1016/j.colsurfb.2013.08.006
- Karimov KA, Kritskii AV, Naboichenko SS, et al. Autoclave precipitation of iron from zinc sulfate solutions. KnE Mater Sci. 2017;2(2):76. doi:10.18502/kms.v2i2.950
- Ristić M, Popović S, Musić S. Formation and properties of Cd(OH)2 and CdO particles. Mater Lett. 2004;58(20):2494–2499. doi:10.1016/j.matlet.2004.03.016
- Yang S, Sun Y, Chen L, et al. Porous iron oxide ribbons grown on graphene for high-performance lithium storage. Sci Rep. 2012;2(1).
- Oliveira L, Rios R, Fabris J, et al. Activated carbon/iron oxide magnetic composites for the adsorption of contaminants in water. Carbon N Y. 2002;40(12):2177–2183. doi:10.1016/S0008-6223(02)00076-3
- Zhu L, Li H, Liu Z, et al. Synthesis of the 0D/3D CuO/ZnO heterojunction with enhanced photocatalytic activity. J Phys Chem C. 2018;122(17):9531–9539. doi:10.1021/acs.jpcc.8b01933
- Sapkota KP, Lee I, Hanif MA, et al. Enhanced visible-light photocatalysis of nanocomposites of copper oxide and single-walled carbon nanotubes for the degradation of methylene blue. Catalysts. 2020;10(3):297–313. doi:10.3390/catal10030297
- Teixeira A, Tristão J, Araujo M, et al. Iron a versatile element to produce materials for environmental applications. J Braz Chem Soc. 2012;23(9):1579–1593. doi:10.1590/S0103-50532012005000039
- Halim MYA, Tan WL, Bakar N, et al. Surface characteristics and catalytic activity of copper deposited porous silicon powder. Materials (Basel). 2014;7(12):7737–7751. doi:10.3390/ma7127737
- Figueiredo JL, Ribeiro FR. (2007). Actividade e Selectividade. In Catálise Heterogénea. Fundação Calouste Gulbenkian, 2ª Edição.
- Rioseco F, Radovic L, García X, et al. Effect of Ag addition on thermal stability and catalytic properties of LaFeO3 perovskite. J Chil Chem Soc. 2010;55(1):44–49. doi:10.4067/S0717-97072010000100011
- Katikaneani P, Vaddepally AK, Reddy Tippana N, et al. Phase transformation of iron oxide nanoparticles from hematite to maghemite in presence of polyethylene glycol: application as corrosion resistant nanoparticle paints. J Nanosci. 2016;2016:1–6. doi:10.1155/2016/1328463
- Scheinost, A. C. Metal oxides. In D. Hillel, editor. Encyclopedia of soils in the environment. New York: Elsevier; 2005. p. 428–438.
- Ma H, Liu Y, Fu Y, et al. Improved photocatalytic activity of copper heterostructure composites (Cu–Cu2O–CuO/AC) prepared by simple carbothermal reduction. Aust J Chem. 2014;67(5):749–756. doi:10.1071/CH13456
- U Picoli S, Durán M, F Andrade P, et al. Silver nanoparticles/silver chloride (Ag/AgCl) synthesized from Fusarium oxysporum acting against Klebsiella pneumouniae carbapenemase (KPC) and extended spectrum beta-lactamase (ESBL). Front Nanosci Nanotechnol. 2016;2(2):107–110. doi:10.15761/FNN.1000117
- Hidayu AR, Muda N. Preparation and characterization of impregnated activated carbon from palm kernel shell and coconut shell for CO2 capture. Procedia Eng. 2016;148:106–113. doi:10.1016/j.proeng.2016.06.463
- Tang Y-B, Liu Q, Chen F-Y. Preparation and characterization of activated carbon from waste ramulus mori. Chem Eng J. 2012;203:19–24. doi:10.1016/j.cej.2012.07.007
- Lester JN. Heavy metals in wastewater and sludge treatment processes. Vol. 1. Florida: CRC Press; 1987. p. 12–14.
- Wanna Y, Chindaduang A, Tumcharern G, et al. Efficiency of SPIONs functionalized with polyethylene glycol bis(amine) for heavy metal removal. J Magn Magn Mater. 2016;414:32–37. doi:10.1016/j.jmmm.2016.04.064
- Al-Khaldi FA, Abu-Sharkh B, Abulkibash AM, et al. Cadmium removal by activated carbon: carbon nanotubes, carbon nanofibers, and carbon fly ash: a comparative study. Desalin Water Treat. 2015;53(5):1417–1429.
- Jusoh A, Su Shiung L, Ali NA, et al. A simulation study of the removal efficiency of granular activated carbon on cadmium and lead. Desalination. 2007;206(1-3):9–16. doi:10.1016/j.desal.2006.04.048
- Yang J, Hou B, Wang J, et al. Nanomaterials for the removal of heavy metals from wastewater. Nanomaterials. 2019;9(3):424. doi:10.3390/nano9030424
- Giraldo L, Erto A, Moreno-Piraján JC. Magnetite nanoparticles for removal of heavy metals from aqueous solutions: synthesis and characterization. Adsorption. 2013;19(2-4):465–474. doi:10.1007/s10450-012-9468-1
- Zapata K, Carrasco-Marín F, Arias JP, et al. Novel biomaterial design based on pseudomonas stutzeri–carbon xerogel microspheres for hydrocarbon removal from oil-in-saltwater emulsions: a new proposed treatment of produced water in oilfields. Journal of Water Process Engineering. 2020;35:101222. doi:10.1016/j.jwpe.2020.101222
- Gehrke I, Geiser A, Somborn-Schulz A. Innovations in nanotechnology for water treatment. Nanotechnol: Sci Appl. 2015;8:1–17. doi:10.2147/NSA.S43773
- Quang DV, Sarawade PB, Jeon SJ, et al. Effective water disinfection using silver nanoparticle containing silica beads. Appl Surf Sci. 2013;266:280–287. doi:10.1016/j.apsusc.2012.11.168
- Ocsoy I, Temiz M, Celik C, et al. A green approach for formation of silver nanoparticles on magnetic graphene oxide and highly effective antimicrobial activity and reusability. J Mol Liq. 2017;227:147–152. doi:10.1016/j.molliq.2016.12.015
- World Health Organization. (2004). Water treatment and pathogen control: process efficiency in achieving safe drinking water.
- Anwar A, Perveen S, Ahmed S, et al. Silver nanoparticle conjugation with thiopyridine exhibited potent antibacterial activity against Escherichia coli and further enhanced by copper capping. Jundishapur J Microbiol. 2019;12(3):e74455.
- Mermod M, Magnani D, Solioz M, et al. The copper-inducible ComR (YcfQ) repressor regulates expression of ComC (YcfR): which affects copper permeability of the outer membrane of Escherichia coli. BioMetals. 2012;25(1):33–43. doi:10.1007/s10534-011-9510-x
- Cai Y, Li C, Wu D, et al. Highly active MgO nanoparticles for simultaneous bacterial inactivation and heavy metal removal from aqueous solution. Chem Eng J. 2017;312:158–166. doi:10.1016/j.cej.2016.11.134
- Yasuyuki M, Kunihiro K, Kurissery S, et al. Antibacterial properties of nine pure metals: a laboratory study using Staphylococcus aureus and Escherichia coli. Biofouling. 2010;26(7):851–858. doi:10.1080/08927014.2010.527000
- Hadrup N, Sharma AK, Loeschner K. Toxicity of silver ions: metallic silver, and silver nanoparticle materials after in vivo dermal and mucosal surface exposure: a review. Regul Toxicol Pharmacol. 2018;98:257–267. doi:10.1016/j.yrtph.2018.08.007
- Mukherjee SG, O’Claonadh N, Casey A, et al. Comparative in vitro cytotoxicity study of silver nanoparticle on two mammalian cell lines. Toxicol in Vitro. 2012;26(2):238–251. doi:10.1016/j.tiv.2011.12.004
- Agnihotri S, Mukherji S. Size-controlled silver nanoparticles synthesized over the range 5–100 nm using the same protocol and their antibacterial efficacy. RSC Adv. 2014;4(8):3974–3983. doi:10.1039/C3RA44507K
- Bogdanović U, Lazić V, Vodnik V, et al. Copper nanoparticles with high antimicrobial activity. Mater Lett. 2014;128:75–78. doi:10.1016/j.matlet.2014.04.106
- Kumar S, Nair RR, Pillai PB, et al. Graphene oxide–MnFe2O4 magnetic nanohybrids for efficient removal of lead and arsenic from water. ACS Appl Mater Interfaces. 2014;6(20):17426–17436. doi:10.1021/am504826q
- Salam MA, Makki MSI, Abdelaal MYA. Preparation and characterization of multi-walled carbon nanotubes/chitosan nanocomposite and its application for the removal of heavy metals from aqueous solution. J Alloys Compd. 2011;509(5):2582–2587. doi:10.1016/j.jallcom.2010.11.094