Abstract
Investigators developed and evaluated a dilution method for the LeadCare II analyzer (LCII) for blood lead levels >65 μg/dL, the analyzer’s maximum reporting value. Venous blood samples from lead-poisoned children were initially analyzed in the field using the dilution method. Split samples were analyzed at the US Centers for Disease Control and Prevention (CDC) laboratory using both the dilution method and inductively coupled plasma-mass spectrometry (ICP-MS). The concordance correlation coefficient of CDC LCII vs. ICP-MS values (N = 211) was 0.976 (95 % confidence interval (CI) 0.970–0.981); of Field LCII vs. ICP-MS (N = 68) was 0.910 (95% CI 0.861–0.942), and CDC LCII vs. Field LCII (N = 53) was 0.721 (95% CI 0.565–0.827). Sixty percent of CDC and 54% of Field LCII values were within ±10% of the ICP-MS value. Results from the dilution method approximated ICP-MS values and were useful for field-based decision-making. Specific recommendations for additional evaluation are provided.
Keywords:
Introduction
An expanding body of literature has demonstrated that lead negatively impacts human health (Laraque et al. Citation1990; Centers for Disease Control and Prevention [CDC] Citation1994, Citation2002; Advisory Committee on Childhood Lead Poisoning Prevention Citation2007). The developing organs of children are particularly vulnerable to lead poisoning. Low levels of lead poisoning cause long-term brain damage and behavior problems while higher levels cause kidney damage, neurological impairment with encephalopathy, and possibly death (Dart et al. Citation2004; Henretig Citation2006; CDC Citation2007). Screening in the USA is often done using point-of-care blood lead testing instrumentation (CDC Citation2002; Stanton et al. Citation2006). Currently, the only commercially available point-of-care blood lead testing analyzer in the USA is the LeadCare II analyzer (LCII), manufactured by Magellan Biosciences (Shannon & Rifai Citation1997). This analyzer is approved by the US Food and Drug Administration (FDA) for blood lead analysis for the range 3.3–65 μg lead/dL of blood. The FDA has determined the LCII to be “substantially equivalent” to graphite furnace atomic absorption spectrometry results, yet simple enough to be waived from standard Clinical Laboratory Improvement Amendment (CLIA) requirements for proficiency testing (FDA Citation2005; Shannon & Rifai Citation1997). Development of the LCII was a milestone in advancing point-of-care measurement for blood lead. The LCII has markedly decreased the time from diagnosis to treatment and has allowed for lead-poisoning investigations to proceed quickly in low-resource settings.
Figure 1. Field LCII vs. ICP-MS values with ±10% error bounds from ICP-MS results*. Triangle: Field LCII vs. ICP-MS values. Dashed lines: ±10% of ICP-MS value. Note: *One point is excluded from this chart for the sake of clarity. It had an ICP-MS result of 286 µg/dL with a Field LCII result of 262.5 µg/dL. This value was within ±10% of the ICP-MS values.
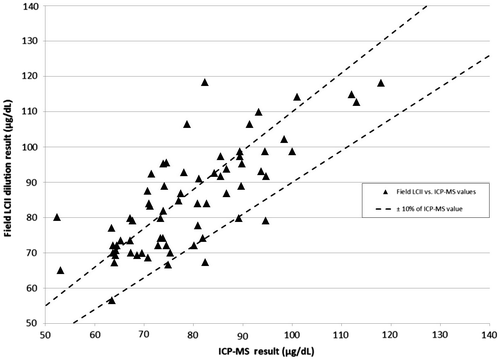
Figure 2. Difference between Field LCII and ICP-MS value as measured against ICP-MS*: Triangle: Field LCII-ICP-MS value. Dashed lines: 95% Level of agreement (−14.6, +25.7). Dot-dash line: Average difference Field LCII – ICP-MS (+5.5). Note: *One point is excluded from this chart as it appeared to be a reporting error. This point had an ICP-MS result of 694 µg/dL with a bias of −234.1.
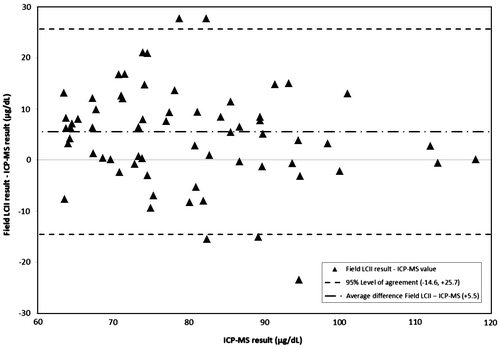
Figure 3. CDC LCII vs. ICP-MS values with ±10% error bounds from ICP-MS results. Triangle: CDC LCII vs. ICP-MS values. Dashed lines: ±10% of ICP-MS value.
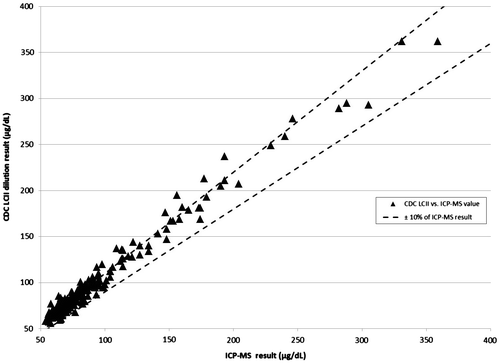
Figure 4. Difference between CDC LCII and ICP-MS value as measured against ICP-MS. Triangle: Field LCII-ICP-MS value. Dashed lines: 95% Level of agreement (−7.9, +23.5). Dot-dash line: Average difference Field LCII – ICP-MS (+7.8).
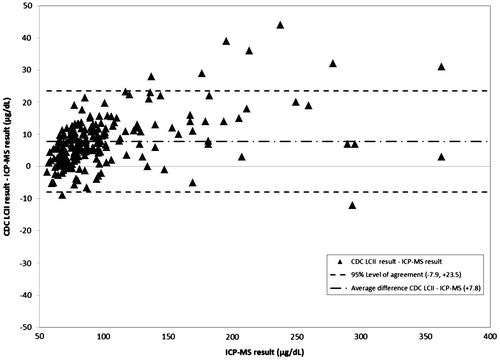
Childhood lead poisoning occurs globally and is often found to be much more severe in low-resource countries compared to the USA. A recent investigation in Nigeria revealed over 100 child fatalities related to lead poisoning (Dooyema et al. Citation2011; Lo et al. Citation2012). There are also reports of lead-related fatalities from Senegal (Haefliger et al. Citation2009), where the World Health Organization (WHO) confirmed at least 18 children died from lead poisoning. Additionally, there are many reports of extremely elevated blood lead levels from other countries (Nduka et al. Citation2008; Orisakwe and Nduka Citation2009; Shi et al. Citation2009; Brown et al. Citation2010; Niu Citation2010; Norman et al. Citation2010; Ramos et al. Citation2010; Rowley Citation2010). In most of these reports, the average BLLs reported were >65 μg/dL, levels considered to be life-threatening to children (CDC Citation2002).
In March 2010, Médecins Sans Frontières (MSF) reported to local health authorities an unusually high number of children under five years of age dying in mining communities in Northern Nigeria. In May 2010, the government of Nigeria requested assistance from the US Centers for Disease Control and Prevention (CDC) to join the effort in identifying and addressing lead poisoning in Northern Nigeria. Investigations revealed that children were being exposed to high levels of finely ground lead-contaminated dust resulting from artisanal gold mining processes. Teams used LCII at the point-of-care to confirm the diagnosis of lead poisoning, triage children for life-saving chelation treatment, and monitor the efficacy of treatment (CDC Citation2010; Dooyema et al. Citation2011; Von Lindern et al. Citation2011). Eighty-five percent of 204 venous blood samples taken from children in the field exceeded the upper reportable limits of the LCII (> 65 μg/dL) (CDC Citation2010, Dooyema et al. Citation2011). Prioritization of medical needs required immediate determination of BLL rather than waiting for inductively coupled plasma-mass spectrometry (ICP-MS) results. This led investigators in the field to collaborate with the CDC laboratory and LCII manufacturer to evaluate methods to modify the LCII method with an additional dilution step to extend the reporting range. Initially, blood was diluted with saline prior to LCII analysis. However, the results of this method consistently overestimated ICP-MS values by twofold or greater and the concordance correlation coefficient (CCC) was poor (0.423, 95% confidence interval (CI) 0.202–0.603, N = 29). A dilution step that used whole blood with lead levels verified to be < 3.3 μg/dL demonstrated markedly improved agreement with ICP-MS results, deserving further attention. This article compares the agreement of the values resulting from a blood dilution method used in the field and laboratory on LCII to ICP-MS values using blood from lead-poisoned children with extremely high BLLs.
Methods
Patients and samples
This study analyzed venous blood samples taken between 14 July 2010 and 24 September 2012 from 227 children aged < 5 years admitted for chelation therapy to the MSF ward. Consent for treatment was obtained from childrens’ parents. Eligible sample values were from those known to have undergone the LCII dilution method either in the field or the CDC laboratory.
Blood for analysis at CDC was drawn directly from the patient into a separate tube and sent to the CDC Inorganic Analytical Toxicology Laboratory in Atlanta, Georgia, USA. Samples were shipped to CDC in batches using ambient-temperature shipping, the only method readily available.
Analytical methods
The CDC laboratory first analyzed samples with ICP-MS (Jones et al. Citation2009) using a modification of a method of Nixon et al. (Citation1999) for analyses of metals in biological matrices. If sufficient blood remained, samples were then analyzed on LCII. Samples whose LCII results were >65 μg/dL (reported as “HI” on the LCII) were prepared and analyzed using the blood dilution method as described below. To ensure quality control and ensure precision of the ICP-MS method, the CDC laboratory undertook repeated ICP-MS analyses of quality control materials during the time of sample processing. Precision (2 SD) of quality control analyses was 7% at 10 μg/dL, 9% at 100 μg/dL, and 4% at 400 μg/dL.
The goal of the dilution method was to dilute the sample to within the operating range of the LCII (3.3–65 μg/dL) without changing the matrix of the blood and reagent-mixture against which the analyzer is calibrated. The method presented here required the diluent be a human blood sample verified to have a BLL < 3.3 μg/dL (reported on the analyzer as “LOW”) using standard LCII analytic methods on a machine calibrated using the test kit materials (ESA Biosciences Inc Citation2007). This will be referred to as “low blood” in the subsequent steps. “Low blood” specimens obtained at each site were typically fresh (drawn within previous 24 hours), but were sometimes reused up to seven days, being stored at 2–8 °C when not in use. The “low blood” reagent-mixture to be used was prepared for analysis in an LCII reagent vial per the standard LCII protocol: 50 μL of the “low blood” specimen was transferred from the mixed (ideally with a vortex) tube of blood obtained from the patient verified to have a BLL < 3.3 μg/dL to an unused LCII reagent vial using an LCII capillary tube and plunger. This was followed by thorough mixing to create a “low blood” reagent-mixture with a total volume of 300 μL (250 μL of reagent and 50 μL of “low blood”). A “high blood” reagent-mixture was then prepared by using a capillary tube and plunger to transfer 50 μL of blood from the tube of the specimen previously determined by LCII analysis to have a BLL > 65 μg/dL (reported as “HI” on the LCII) into a separate, unused, LCII reagent vial. Next, 50 μL of the “high blood” reagent-mixture was transferred to the vial containing 300 μL of “low blood” reagent-mixture using an unused LCII capillary tube and plunger, mixing well after the transfer. One drop of this final mixture was then analyzed on the LCII. Note that the LCII is calibrated to give results given a 1 + 5 dilution of blood upon adding 50 μL of blood to a 250 μL reagent container (50 μL in 300 μL of total solution = sixfold dilution). By adding an already diluted 50–300 μL to create a total volume of 350 μL, there was an additional 1 + 6 dilution (50 μL in 350 μL of total solution = sevenfold dilution). Hence, to obtain the measured blood lead concentration in the “HI” sample, investigators simply multiplied the LCII result by 7.
Statistical analysis
Field and CDC LCII dilution values were independently compared to ICP-MS values as well as each other if available. For the Field LCII values, only those known to have resulted from the dilution procedure described above were considered in this analysis.
Agreement was determined using three approaches. First, the CCC, a common agreement coefficient used in medical device validation studies which takes into account the uncertainties of both methods, was applied to compare the data from either the Field or Laboratory LCII dilution values to the ICP-MS results, as well as to each other (Lin Citation1989; Lin Citation2008). CCC results can range from 0 to 1.0, with 0 indicating no agreement and 1.0 indicating perfect agreement. A CCC result of ≥0.7 was used to indicate good agreement. Next, to help healthcare providers in the field interpret a LCII value from the dilution method in relation to the ICP-MS value, investigators determined the percent of the LCII dilution values that were within ±10% of the ICP-MS values. Finally, values were evaluated as to their positive or negative bias in comparison to ICP-MS values using the mean bias observed in all samples as well as the mean bias in quartiles based upon ICP-MS values, similar to that used by Taylor et al. (Citation2001). This information was used to depict the differences between the Field and CDC LCII dilution values in comparison to ICP-MS results (Field/CDC LCII dilution value – ICP-MS) with boundaries of ±1.96 standard deviations to indicate the range of variability in 95% of the samples. Information was entered in Microsoft Excel 2010 (Microsoft Corp., Redmond, WA) and analyzed in SAS version 9.3 (SAS Institute Inc. Carey, NC) (SAS Institute Citation2002–2010).
Results
A total of 227 samples were analyzed by the CDC laboratory using ICP-MS. Sixteen samples could not be further analyzed using the dilution method at CDC due to lack of sufficient sample volume and 158 samples from the field had no information regarding either the result or the dilution method used. This resulted in a total of 69 Field LCII dilution values that could be verified as having been done using the method reported above; the reported Field LCII values range was 56.5–262.5 μg/dL. There were 211 CDC LCII dilution values (range 56.0–362.0 μg/dL). Field and CDC LCII values were compared to ICP-MS values (geometric mean 86.9, range 52.4–694.0 μg/dL). There were 53 Field LCII values for comparison to CDC LCII values.
Method comparison
The CCC of CDC LCII vs. ICP-MS values was 0.976 (95% confidence interval (CI) 0.970–0.981); of Field LCII vs. ICP-MS was 0.910 (95% CI 0.861–0.942); and of CDC LCII vs. Field LCII was 0.721 (95% CI 0.565–0.827) (Table ).
Table 1. CCC results comparing each site vs. ICP-MS as well as to each other.
The ICP-MS quartiles were ≤66.6, 66.7–77.5, 77.6–94.7 and >94.7 μg/dL. Overall, 60% of CDC LCII laboratory values were within ±10% of the ICP-MS value; as were 54% of Field LCII values (Figures –). There were no statistically significant differences between quartiles in regard to the percent within ±10% of the ICP-MS value from either laboratory (Table ).
Table 2. Number and percent of CDC and Field LCII dilution procedure values within ±10% of the ICP-MS value by ICP-MS quartile.
CDC LCII values overestimated ICP-MS values by an average of 7.8 μg/dL (8% of the average ICP-MS value), with 95% of values falling between −7.9 and + 23.5 μg/dL. Field LCII values overestimated ICP-MS values by an average of 5.5 μg/dL (7% of the average ICP-MS value), with 95% of values falling between −14.6 and 25.7 μg/dL. One outlying Field LCII value skewed the quartile and overall bias for the Field LCII values; this measured value was 459.0 μg/dL and ICP-MS value was 694.0. This value was excluded from analysis or figures as it was not possible under the dilution procedure (i.e. at most the measured value could have been 65 μg/dL × 7 = 455 μg/dL).
Discussion
As a number of factors – including time from sampling to analysis, field analysis and shipping conditions, field staff training, and quality control – affected the findings, they are reported here to provide a better context prior to discussing results.
Factors affecting results
The long times between blood being drawn and being analyzed by LCII at CDC (approximately 2–3 weeks) as well as the high ambient temperatures for analysis and shipping in the field were certainly outside of manufacturer-recommended conditions for analysis of blood on LCIIs in either laboratory. The LCIIs used in this investigation have an operational temperature range 12.2–36.1 °C and relative humidity range 12–80% (ESA Biosciences Inc Citation2007). The investigation area bordered the Sahara desert and local radio reports indicated daily temperatures well above 38 °C. The closest town with easily accessible temperature records (Kano, Nigeria), approximately 200 miles away from the MSF field station, reported an average daily high of 37.2 °C and a monthly record high of 43.9 °C with average relative humidity ranging between 33 and 81% for May–June (BBC Citation2010). These conditions were not often amenable to the proper functioning of the LCIIs in the field. When daily indoor temperatures in the field laboratory exceeded the LCII temperature parameters, the LCIIs would simply turnoff. This required analyses to be undertaken in the cooler mornings and late afternoons. Electricity was initially unavailable, thus neither indoor air conditioning (which provided both temperature and dust control) nor external power for the LCIIs was available; the analyzers were operated using batteries. Manufacturer instructions included in the LCII indicate that unrefrigerated samples should be analyzed “within 24 hours stored at 10.0 –32.2 °C.” Given the conditions noted above, these requirements could not always be met in the field and, despite the best efforts at using a timely cold-chain technique, could not be met during transit of samples to the CDC laboratory.
As can be expected with any novel method developed and implemented in the field, the development, implementation, and refining of laboratory procedures, including quality assurance, occurred over time. Unfortunately, the operational constraints inherent in the start-up of any large medical intervention did not allow for investigators to completely control which blood draw equipment was used and not all equipment was pre-screened for lead. Investigators estimate that approximately 70% of the samples taken used blood draw and storage materials pre-screened for lead. Also as a consequence of a new operation, proper sampling, liquid transfer, storage, and transportation procedures for blood were reviewed and put in place over time. Having health care workers with limited experience operating in a low-resource setting implement a novel method in the field laboratory may have introduced some operator errors. In addition, the small sample volumes used and the temperatures encountered in the field could have caused some evaporation of blood samples, leading to false high readings on the Field LCII due to concentrated blood. Lastly, while practical on a short-term basis, a method relying on a constant supply of blood from donors with BLLs below 3.3 μg/dL would likely be difficult to sustain over longer time periods in highly lead-contaminated settings.
Yet, despite these extremes of time, temperature, and quality assurance in the field there appeared to be remarkable correlation and moderately good approximation of blood lead levels when using this dilution method. CCC analyses for both laboratories in relation to the ICP-MS as well as to each other were good and the agreement analyses also indicated concordance within a range that would be helpful to make clinical decisions.
Clinically, both the Field and CDC LCII dilution values consistently overestimated BLLs determined by ICP-MS but not so much as to be misleading for diagnosis or treatment. Finding that 95% of the differences for CDC LCII values vs. ICP-MS were −11.2–26.6 μg/dL and that 60% of these samples were within ±10% of the ICP-MS value was promising considering an average BLL of 86.9 μg/dL, given the observed precision of the ICP-MS method quality control analyses. Data with this amount of difference from laboratory-based methods would still be considered helpful to at least initiate and monitor life-saving chelation therapy under appropriate protocols until values came within a range that could be measured using standard LCII procedures. Additionally, 95% of the differences for Field LCII values vs. ICP-MS values were −14.6–25.7 μg/dL and approximately half (54%) of Field LCII values were within ±10% of the ICP-MS value, this added assurance as to the clinical applicability of the values resulting from this method. Finally, as there were no significant differences between ICP-MS quartiles for either laboratory it indicated to investigators that this bias was not related to the concentration of lead in the blood analyzed.
While these results are promising, there was still considerable variability and bias in the findings presented; a variety of factors likely contributed to these findings. Despite the best of efforts, ambient lead levels in the field laboratory may have been higher than those in the CDC laboratory. Small volumes used in combination with higher temperatures in the field likely caused evaporation and concentration of the sample in the LCII, which may have resulted in a reported concentration greater than the actual concentration. Use of a laboratory pipettes with disposable tips instead of the capillary tubes and plungers that come with the LCII test kits would provide more reproducible liquid transfer during the dilution method steps. However, these supplies were not available at the point-of-care, so use of LCII test kit supplies was required in the field (and maintained at CDC to mirror the procedures being used in the field). Finally, prolonged shipping times and high temperatures may also have affected the blood prior to it being analyzed on the LCII at CDC laboratories.
CDC and MSF strongly recommend all laboratories adhere to a rigorous quality assurance program. This program should include internal quality control procedures, using materials provided by the manufacturer that are evaluated for lead content and rigorous standard operating procedures. The LCII, when operated in its normal manner, should not need external quality control with a reference laboratory. In Nigeria, the LCII was being used outside of the manufacturer’s instructions and operating environment. For this reason, MSF developed a pragmatic quality control protocol with reference laboratories that included repeat analysis of every 10th BLL > 65 μg/dL by a separate technician, over-sampling of blood specimens requiring the dilution method for the reference laboratories and, the use of small, frequent batches of samples for validation to allow the program to make data-driven decisions quickly. The low statistical power of such a protocol can be overcome by retrospective quartile analysis of samples collected over time (Klarkowski and Orozco Citation2010). As of October 2012, 55 samples that required the use of the dilution method had been analyzed by two separate technicians in the field laboratory. The CCC between values from the two technicians was 0.976 (95% CI 0.965–0.984), indicating good agreement. These results indicate that a reliable field-based blood lead testing program utilizing the dilution procedure is achievable.
Conclusion
These results indicate that, while additional work is needed to evaluate this procedure and why results overestimated the ICP-MS values, it appears that this novel dilution method can be used to both inform clinical decisions regarding baseline BLLs and monitor the effectiveness of therapy when BLLs are > 65 μg/dL in the field. Future evaluations of this method should focus on a rigorous evaluation of field implementation in comparison to a reference laboratory so as to produce more accurate results for clinical decision-making in the field. Specifically, the issues that need to be addressed are (i) improving field reporting of BLLs to central laboratories (including test kit lot numbers, analytic method, technician identifier, and patient-tracking information); (ii) evaluation of the accuracy and precision of the LCII dilution method in the field with a reference instrument utilizing a more planned approach; (iii) protocols for screening all the blood sampling materials as well as for their storage in lead-contaminated environments; (iv) a process evaluation of the procedure to determine the most efficient means of implementing it; and (v) undertaking a search for a diluent that does not rely upon low-BLL human blood, as this resource in such settings is limited.
Acknowledgments
The authors wish to acknowledge the Nigerian Government, United States Agency for International Development, United Nations, WHO, and Terragraphics Environmental Engineering Inc. for their partnership in responding to this environmental disaster. The findings and conclusions in this report are those of the authors and do not necessarily represent the views of the CDC.
References
- Advisory Committee on Childhood Lead Poisoning Prevention. 2007. Interpreting and managing blood lead levels <10 μg/dL in children and reducing childhood exposures to lead: recommendations of CDC’s advisory committee on childhood lead poisoning prevention. MMWR Morb Mortal Wkly Rep. 56:1–14.
- BBC. 2010. BBC average weather conditions for Kano, Nigeria [Internet]. London, England; [cited 2011 Jan 25]. Available from: http://www.bbc.co.uk/weather/world/city_guides/results.shtml?tt=TT000500
- Brown MJ, McWeeney G, Kim R, Tahirukaj A, Bulat P, Syla S, Savic Z, Amitai Y, Dignam T, Kaluski DN. 2010. Lead poisoning among internally displaced Roma, Ashkali and Egyptian children in the United Nations-Administered Province of Kosovo. Eur J Public Health. 20:288–292.
- CDC. 1994. Blood lead levels–United States, 1988–1991. MMWR Morb Mortal Wkly Rep. 43:545–548.
- CDC. 2002. Managing elevated blood lead levels among young children: recommendations from the advisory committee on childhood lead poisoning prevention [Internet]. In: Centers for Disease Control and Prevention, editor. Available from: http://www.cdc.gov/nceh/lead/CaseManagement/caseManage_main.htm
- CDC. 2007. Interpreting and managing blood lead levels <10μg/dL in children and reducing childhood exposures to lead. MMWR Morb Mortal Wkly Rep. 56:1–14.
- CDC. 2010. Notes from the field: Outbreak of acute lead poisoning among children ages <5 years – Zamfara, Nigeria, 2010. MMWR Morb Mortal Wkly Rep. 59:846.
- Dart R, Hurlbut K, Boyer-Hassen L. 2004. Lead. In: Dart R, Caravati E, Mcguigan M, Macgregor Whyte IDawson A, Seifert S, Schonwald S, Yip L, Keyes D, Hurlbut K, Erdman A, editors. Medical toxicology. Philadelphia, PA: Lippincott, Williams & Wilkins; p. 1423–1431.
- Dooyema CA, Neri A, Lo Y-C, Durant J, Dargan PI, Swarthout T, Biya O, Gidado SO, Haladu S, Sani-Gwarzo N, et al. 2011. Outbreak of fatal childhood lead poisoning related to artisanal gold mining in Northwestern Nigeria, 2010. Env Health Persp. 120:601–607.
- ESA Biosciences Inc. 2007. Testing kit instructions for LeadCare II analyzer. Chelmsford (MA): ESA Biosciences.
- FDA. 2005. Recommendations for clinical laboratory improvement amendments of 1988 waiver applications [Internet]. In: FDA Center For Devices And Radiological Health, editor. Washington, DC: FDA. Available from: http://www.fda.gov/downloads/MedicalDevices/DeviceRegulationandGuidance/GuidanceDocuments/ucm070890.pdf
- Haefliger P, Mathieu-Nolf M, Lociciro S, Ndiaye C, Coly M, Diouf A, Faye AL, Sow A, Tempowski J, Pronczuk J, et al. 2009. Mass lead intoxication from informal used lead-acid battery recycling in dakar, senegal. Environ Health Perspect. 117:1535–1540.
- Henretig F. 2006. Lead. In: Wonsiewicz M, Edmonson K, Boyle P, editors. Goldfrank’s toxicologic emergencies. New York: McGraw-Hill.
- Jones RL, Homa DM, Meyer PA, Brody DJ, Caldwell KL, Pirkle JL, Brown MJ. 2009. Trends in blood lead levels and blood lead testing among US children aged 1 to 5 years, 1988–2004. Pediatrics. 123:e376–e385.
- Klarkowski DB, Orozco JD. 2010. Microscopy quality control in Medecins Sans Frontieres programs in resource-limited settings. PLoS Med. 7:e1000206.
- Laraque D, Mccormick M, Norman M, Taylor A, Weller SC, Karp J. 1990. Blood lead, calcium status, and behavior in preschool children. Am J Dis Child. 144:186–189.
- Lin L. 2008. Overview of agreement statistics for medical devices. J Biopharm Stat. 18:126–144.
- Lin LI. 1989. A concordance correlation-coefficient to evaluate reproducibility. Biometrics. 45:255–268.
- Lo YC, Dooyema CA, Neri A, Durant J, Jefferies T, Medina-Marino A, De Ravello L, Thoroughman D, Davis L, Dankoli RS2012. Childhood lead poisoning associated with gold ore processing: a village-level investigation – Zamfara State, Nigeria, October–November 2010. Env Health Persp. 120:1450–1455.
- Nduka JK, Orisakwe OE, Ezenweke LO, Chendo MN, Ezenwa TE. 2008. Heavy metal contamination of foods by refuse dump sites in Awka, southeastern Nigeria. Scientific World J. 8:941–948.
- Niu KY. 2010. An acute tetraethyl lead poisoning accident. Zhonghua Lao Dong Wei Sheng Zhi Ye Bing Za Zhi. 28:644.
- Nixon DE, Burritt MF, Moyer TP. 1999. The determination of mercury in whole blood and urine by inductively coupled plasma mass spectrometry. Spectrochim Acta Part B-Atomic Spectroscopy. 54:1141–1153.
- Norman R, Bradshaw D, Lewin S, Cairncross E, Nannan N, Vos T. 2010. Estimating the burden of disease attributable to four selected environmental risk factors in South Africa. Rev Environ Health. 25:87–119.
- Orisakwe OE, Nduka JK. 2009. Lead and cadmium levels of commonly administered pediatric syrups in Nigeria: a public health concern? Sci Total Environ. 407:5993–5996.
- Ramos CL, Barros HM, Stein AT, Da Costa JS. 2010. Risk factors contributing to childhood poisoning. J Pediatr (Rio J). 86:435–440.
- Rowley NE. 2010. Lead poisoning from Burmese medicines. Minn Med. 93:6.
- SAS Institute. 2002–2010. SAS v9.3. Carey, NC: SAS Institute.
- Shannon M, Rifai N. 1997. The accuracy of a portable instrument for analysis of blood lead in children. Ambulatory Child Health. 3: 249–254.
- Shi H, Jiang YM, Li JY, Liu F, Wang H, Yu F, Yang H. 2011. Environmental lead exposure among children in Chengdu, China, 2007–2009. Biol Trace Elem Res. 143:97–102.
- Stanton NV, Fritsch T, Geraghty C, Verostek MF, Weiner B, Parsons PJ. 2006. The role of proficiency testing in the detection and resolution of calibration bias in the LeadCare (R) blood lead analyzer; limitations of peer-group assessment. Accredit Qual Assur. 11:590–592.
- Taylor L, Jones RL, Kwan L, Deddens JA, Ashley K, Sanderson WT. 2001. Evaluation of a portable blood lead analyzer with occupationally exposed populations. Am J Ind Med. 40:354–362.
- Von Lindern IH, Von Braun MC, Tirima S, Bartrem C. 2011. Zamfara, Nigeria lead poisoning epidemic emergency environmental response, May 2010–March 2011, Final Report to the United Nations Childrens Fund (UNICEF) [Internet]. In: UNICEF Fund, editor. UNICEF programme cooperation agreement: YW-303(01). Available from: http://www.blacksmithinstitute.org/files/FileUpload/files/Additional%20Reports/Zamfara-Nigeria-Report.pdf