Abstract
Eukaryotic microalgae serve as indicators of environmental change when exposed to severe seasonal fluctuations. Several environmental stress conditions are known to produce reactive oxygen species in cellular compartments, resulting in oxidative damage and apoptosis. The study of cell death in higher plants and animals has revealed the existence of an active ‘programmed cell death’ (PCD) process and similarities between such processes suggest an evolutionary origin. A study was undertaken to examine the morphological, biochemical and molecular responses of the unicellular green alga Chlamydomonas reinhardtii after exposure to oxidative (10 mM H2O2) and osmotic (200 mM NaCl and 360 mM sorbitol) stress. Concentrations of H2O2 (2–50 mM), NaCl and sorbitol (100–800 mM) were negatively correlated with growth. Biochemical analyses showed an increase in intracellular H2O2 production (2.2-fold with H2O2 and ~1.2–1.4-fold with NaCl and sorbitol) and activities of some antioxidant enzymes [super oxide dismutase (SOD), catalase (CAT) and ascorbate peroxidase (APX)]. Alteration of mitochondrial membrane potential (MMP) was observed upon treatment with H2O2 and NaCl, but not with sorbitol, indicating that the ionic stress component of NaCl altered the MMP. In addition, H2O2 led to the activation of a caspase-3-like protein, increase in the cleavage of a poly(ADP) ribose polymerase-1 (PARP-1)-like enzyme and formation of DNA nicks and laddering. With NaCl and sorbitol, no caspase activation, nor oligonucleosomal DNA laddering was observed, indicating non-apoptotic death. However, genomic DNA of NaCl (800 mM)-stressed cells, but not those of sorbitol-treated cells showed complete shearing. We conclude that the ionic rather than the osmotic component of NaCl leads to necrosis. These results unequivocally suggest that the vegetative cells of C. reinhardtii respond differentially to various stress agents, leading to different death types in the same organism. Moreover, unlike most other organisms, when exposed to NaCl this alga does not undergo PCD.
INTRODUCTION
Plants and algae are often exposed to environmental fluctuations. Some of these changes are drastic enough to affect their growth and such conditions are termed as stress. This stress could be abiotic (high salt, anaerobic conditions, high and low temperatures, heavy metals) or biotic. If these stress conditions become intense, they cause damage to crops and flora, resulting in considerable environmental and economic losses worldwide. Additionally, in the course of evolution, and due to targeted selection, many plant species have developed the capacity to adapt to these unfavourable conditions. Such adaptive processes are aimed at the sustainability of the plant cell and thus, on survival of the whole organism. Plants have also developed different mechanisms that are aimed at the selective death of some cells and tissues under abiotic and biotic stress conditions. This kind of selective cell death ultimately gives survival benefits for the entire organism (Jackson & Armstrong, Citation1999; Drew et al., Citation2000). Therefore, understanding the molecular mechanism of how plants and microorganisms sense environmental signals and communicate these to the cellular machinery to activate appropriate responses is of fundamental importance in biology (Xiong & Zhu, Citation2002).
Oxidative and osmotic stresses are known to differ significantly even while displaying related responses. In general, oxidative stress gets triggered by the intracellular accumulation of reactive oxygen species (ROS) (Moradas-Ferreira et al., Citation1996) or with disruption of the cellular redox state (Teixeira de Mattos & Neijssel, Citation1997). The oxidative defence mechanisms encompass both enzymatic (SOD, CAT, peroxidases) and non-enzymatic (glutathione, thioredoxin) detoxification that can remove ROS or restore the redox balance. Oxidative stress signals are known to come either from the environment, or to be generated internally, and cause molecular damage to, for example, membranes, DNA and proteins (Moradas-Ferreira et al., Citation1996). It is well established that ROS have a direct effect on the mitochondrial morphology, probably leading to PCD (Logan Citation2006). Moreover, various studies have shown that experimental exposure of plant cells to different concentrations of H2O2 may induce different cell death pathways (Houot et al., Citation2001; Gechev & Hille, Citation2005; Gechev et al., Citation2006; de Pinto et al., Citation2006; Darehshouri et al., Citation2008).
On the other hand, salinity stress is one of the major abiotic stress agents that encumbers plant productivity (Boyer, Citation1982). In general, high salt concentrations encompass ionic and osmotic stress (Zhu, Citation2002). High concentrations of NaCl in the environment affect plants in many ways. Such effects can lower photosynthetic productivity, prevent water and nutrient uptake and cause an imbalance of cellular ions in both aquatic and terrestrial plants (Huh et al., Citation2002; Mahajan & Tuteja, Citation2005). This in turn leads to inhibition of plant growth and even death (Tuteja, Citation2007). Therefore, to cope with and survive under various environmental conditions, plants must acclimatize to stress situations by invoking diverse physiological and molecular mechanisms. The common strategies that plants use to counteract hyperosmotic stress induced by excessive salinity are (i) to prevent water loss by synthesizing and accumulating counter-osmolytes, and (ii) to maintain the internal concentration of Na+ by regulating the corresponding transporters or pump (Bohnert et al., Citation1999). However, under high salinity stress, plants produce second messengers such as Ca2+ and ROS (Witzel et al., Citation2009; Miller et al., Citation2010). These important players use effector molecules to start a phosphorylation cascade that activates the major stress-responsive genes leading to acclimation, thereby helping the plant to cope with unfavourable conditions (Mahajan & Tuteja, Citation2005). An important aspect is the role of cytosolic K+ homeostasis under hyper-saline conditions. Cytosolic K+ homeostasis is important for normal cell metabolism and functioning. Various studies have now shown that under hyper-saline conditions, there is a drastic reduction in plant K+ content, both in roots and shoots (Aziz & Khan, Citation2001; Chen et al., Citation2005; Tavakkoli et al., Citation2011; Shabala & Pottosin, Citation2014). Many studies have also suggested the existence of a strong correlation between shoot K+ content and plant salinity tolerance (Chen et al., Citation2005; Garthwaite et al., Citation2005). Chen and co-workers (2005) showed that NaCl treatment of barley roots resulted in a massive K+ loss (due to plasma membrane depolarization), while isotonic mannitol stress resulted in membrane hyperpolarization and increased net K+ uptake. Maintaining K+ in the roots has been shown as one of the key features conferring salinity stress tolerance in barley (Chen et al., Citation2005, Citation2007), wheat (Cuin et al., Citation2008, Citation2011) and lucerne (Smethurst et al., Citation2008). Taken together the cellular ionic concentration of K+, H+ and Na+ play a vital role in salinity stress and might dictate the potential of plants to survive under these conditions. For instance, the proper control of K+ and H+ flux across the plasma membrane by K+-permeable channels, the accurate maintenance of K+/Na+ ratio in the cytosol and the compartmentation of excessive Na+ by the tonoplast Na+/H+ exchanger have been shown to be the key determinants of salt tolerance in several plants (Shabala et al., Citation2007). Although this response seems to be the common denominator among plant species, some variability in salinity tolerance has been observed (Turan & Tripathy, Citation2012). Since these responses start from a complex network of cellular events, such diversity in salt tolerance may originate from minor differences at the molecular level. Thus, understanding the molecular mechanism of how different plant species behave to salt stress may offer a better reasoning of how certain plants successfully adapt to the high saline environment, while others cannot. In land plants and some algae, it has been reported in the past that high salinity leads to PCD. This could be considered as a salt acclimation response (Huh et al., Citation2002). In Arabidopsis and tobacco, the salt-induced PCD is mediated by ion disequilibrium (Huh et al., Citation2002; Shabala et al., Citation2007). Various candidates such as ROS, antioxidant enzymes, mitochondria permeability transition and the secondary messenger Ca2+ have been thought to be involved in the signalling pathway of salt-induced plant PCD (Lin et al., Citation2005, Citation2006; Li et al., 2007; Chen et al., Citation2009). However, most of the studies on salt stress have been performed in higher plants; data on the type of cell death response induced upon salt stress in green algae are rather limited. Moreover, as PCD is one of the stress-tolerant strategies in higher plants under oxidative and osmotic stress conditions (Huh et al., Citation2002), understanding PCD might be of ecological relevance in a unicellular alga – a physiological response that might guarantee survival of the population under unfavourable conditions.
Over the past two decades, evidence has accumulated showing that various single-celled organisms, both prokaryotes (Lewis, Citation2000; Kolodkin-Gal et al., Citation2007; Bidle et al., Citation2010) and eukaryotes, and from algae to yeasts (Ameisen et al., Citation1995; Vardi et al., Citation1999; Das et al., Citation2001; Lee et al., Citation2002; Madeo et al., Citation2002; Franklin & Berges, Citation2004; Hardwick & Cheng, Citation2004; Moharikar et al., Citation2006; Tchernov et al., Citation2011; Sirisha et al., Citation2014) undergo genetically controlled cell death in response to environmental stress. The wide diversity of organisms in which at least some PCD machinery components were observed to be present may indicate its early appearance (Nedelcu, Citation2009). Despite intensive efforts, the mechanisms whereby the environmental signals induce PCD in unicellular eukaryotes and the cascades of events involved are both poorly understood, as is the evolutionary role of altruistic suicidal behaviour in unicellular organisms (Vardi et al., Citation1999; Franklin et al., Citation2006; Murik & Kaplan, Citation2009; Nedelcu et al., Citation2011). Several homologues of genes involved in mammalian apoptosis have been identified in unicellular lineages (Madeo et al., Citation2002; Fahrenkrog et al., Citation2004; Wissing et al., Citation2004; Walter et al., Citation2006; Moharikar et al., Citation2007). However, many others are reportedly not explored (Shemarova, Citation2010) in those algae that play an important role in the equilibrium of aquatic ecosystems and represent highly suitable biological indicators of environmental changes because of their exposure to seasonal fluctuations.
The programme that ultimately leads to self-destruction of the cell seems to be distinct, ranging from autophagy, apoptosis and necrosis to intermediate forms of these processes, and has led to an active debate in the literature. Necrosis may be defined as a cell death mechanism that would normally occur in the absence of apoptosis (Leist & Jaattela, Citation2001). Necrosis as a process was described to occur as a result of severe physicochemical stress, such as mechanical stress, heat, freeze-thawing, osmotic shock and high concentration of hydrogen peroxide. Under these circumstances, cell death occurs rigorously and is attributable to the direct effect of the stress on the cell, and therefore this cell death process has been described as an accidental and uncontrolled process (Krysko et al., Citation2008). However, to date, a specific pathway of necrotic cell death has not been elucidated. Moreover, no clear biochemical definition of necrotic cell death exists and consequently no positive biochemical makers that unambiguously discriminate necrosis from apoptosis. Several earlier reports suggest that those stimuli that induce apoptosis can also induce necrosis at a higher concentration (Kirschnek et al., Citation2004). This has been distinctly demonstrated for signalling through death receptors such as TNFR1 (Vercammen et al., Citation1998) and CD95 (Holler et al., Citation2000). Therefore, elucidating the cell death pathways is genetically more amenable and easier using a unicellular algal system, such as Chlamydomonas reinhardtii (Chlorophyta).
The unicellular photoautotroph Chlamydomonas reinhardtii is a species of green algae (Chlorophyta) found all over the world as a fresh water and soil inhabitant (www.chlamy.org). These single-celled individuals are always in contact with the environment, encountering high oxidative and salt stress in the surrounding water. Hence, the microalgae can serve as good models for investigation of plant stress response at the cellular and molecular level. Chlamydomonas reinhardtii is an established model organism to study various important physiological processes such as respiration, photosynthesis, cell division, genome stability and adaptive response in the presence of radiation and chemical mutagens. Physiological responses to stress, such as high and low temperature, osmotic and oxidative conditions, have been scored as well (Harris, Citation2001; Hanikenne, Citation2003; Hema et al., Citation2007; Dimova et al., Citation2008; Mastrobuoni et al., Citation2012). Chlamydomonas reinhardtii has also been employed in investigations of PCD (Moharikar et al., Citation2006, Citation2007; Yordanova et al., Citation2009, Citation2010, Citation2013; Pérez-Pérez et al., Citation2010; Durand et al., Citation2011; Zuo et al., Citation2012; Sirisha et al., Citation2014). When exposed to UV-C, it has been shown to induce hallmarks akin to animal apoptosis such as DNA laddering, externalization of phosphatidylserine and occurrence of terminal deoxynucleotidyl transferase-mediated dUTP nick end-labelling (TUNEL)-positive nuclei (Moharikar et al., Citation2006). Some of the abiotic stress conditions such as menadione stress (Sirisha et al., Citation2014) and acetic acid stress (Zuo et al., Citation2012) also showed similar features of cell death. Previous studies indicated that in C. reinhardtii, H2O2-induced cell death associated with some morphological features of PCD (Murik & Kaplan, Citation2009). Earlier reports in other algae also showed that both oxidative and osmotic stress agents induce PCD (Bidle & Falkowski, Citation2004; Zuppini et al., Citation2007; Darehshouri et al., Citation2008; Affenzeller et al., Citation2009; Andronis & Roubelakis-Angelakis, Citation2010; Wang et al., Citation2010; Yazdani & Mahdieh, Citation2012; Li et al., Citation2014; Monetti et al., Citation2014). However, the molecular mechanism through which H2O2 triggers PCD remains elusive. Thus far, there has been no information on the NaCl-induced necrosis in C. reinhardtii. Therefore, understanding the mechanism of oxidative and osmotic-induced cell death pathways in a representative algal system such as C. reinhardtii may help understand the response of plants to various stress agents. Hence, elucidating the mode of action of abiotic stress on PCD in algae will expand the existing information on general questions regarding the altruistic biological role of PCD as a survival mechanism in algal populations and might provide more insight into the evolutionarily conserved/diversified mechanisms of PCD in the eukaryotic cells.
The aim of this study has been to characterize morphological, biochemical and molecular determinants upon H2O2 and NaCl-treated C. reinhardtii, with a focus on the possible dependency of cell death on caspase-like proteases, involvement of mitochondria, PARP, DNA disintegration and the phenotypic appearance of cell death. To resolve the effect of the osmotic component of NaCl that encompasses both ionic and osmotic, the physiological response of cells exposed to sorbitol alone was also monitored.
MATERIALS AND METHODS
Cell culture conditions and exposure to H2O2 and NaCl
Chlamydomonas reinhardtii (strain CC-124) was obtained from the Chlamydomonas Genetic Centre, Duke University, USA. Cells were grown and maintained by periodic transfers in tris acetate phosphate (TAP, pH 7) medium as mentioned by Khona et al. (Citation2013). For all experiments, ~1× 108 cells ml−1 were exposed to different concentrations of H2O2 (2, 5, 10 and 50 mM), NaCl and sorbitol (100, 200, 400 and 800 mM). After exposure, the cells were incubated at 23ºC on a gyratory shaker for various time-points (1, 3, 6, 12, 18 and 24 h) to allow expression of morphological and biochemical alterations.
Cell death assay using Evans blue dye
After exposing the cells to different concentrations of H2O2 (2–50 mM), NaCl and sorbitol (100–800 mM) for various time periods (1–24 h), cell death was assayed by incubating an aliquot of the cell suspension for 15 min with 1% Evans blue (Thomas Baker, Mumbai, India). Extensive washing with TAP medium removed the unbound dye, whereas dye bound to dead cells was solubilized in a solution containing 50% (v/v) methanol and 1% sodium dodecyl sulphate (SDS) for 30 min at 50ºC and quantified by absorbance at 600 nm (Delledone et al., Citation2001) with Infinite® 200 PRO-Tecan, (Männedorf, Switzerland). The absorbance values recovered for heat-treated cells (100% death) were taken as the positive control and percentages for treated cells were calculated accordingly. For oxidative stress, 10 mM H2O2 was used and for salt treatment, 200 mM NaCl was added to the culture medium. To discriminate from osmotic effects, sorbitol concentration (360 mM) that is iso-osmotic to 200 mM NaCl, was used for all the experiments.
Determination of intracellular H2O2 content
For determination of cellular H2O2, 25 ml cell suspension was collected at stipulated time-points and harvested by centrifugation. The algal pellets were re-suspended in 3 ml cold acetone, crushed with a mortar and pestle, and centrifuged at 10 000×g for 10 min at 4°C. Two ml distilled water was added into 1 ml supernatant, and the solution was extracted with a mixture of carbon tetrachloride (CCl4) and chloroform (CHCl3) in a 3:1 ratio. The mixture was centrifuged at 4000×g for 1 min, and the upper aqueous phase was used for H2O2 determination that was measured at OD560 using xylenol orange (Jianga et al., Citation1990) with Infinite® 200 PRO- Tecan (Männedorf, Switzerland).
Measurement of antioxidant enzyme activities and analyses of transcripts
The algal pellets centrifuged from 25 ml C. reinhardtii cultures were re-suspended in 1 ml of 50 mM buffer solution, containing 4.6 mM potassium dihydrogen phosphate (KH2PO4) and 45.4 mM dipotassium hydrogen phosphate (K2HPO4), crushed with a mortar and pestle, and centrifuged at 12 000×g for 25 min at 4°C. The supernatant was subsequently used to determine the activities of antioxidant enzymes. The SOD, CAT and APX activities were determined respectively, according to the methods of Beauchamp & Fridovixh (Citation1971), Nakano & Asada (Citation1981) and Aebi (Citation1984).
For the analyses of transcripts of these antioxidant enzymes, cells were incubated independently with 10 mM H2O2, 200 mM NaCl and 360 mM sorbitol at different time points (0–24 h). Total RNA was extracted using Trizol (Merck, India) according to the manufacturer’s protocol. For first-strand synthesis, reverse transcription was carried out at 42°C in a 25 µl reaction mixture including 2 µg of RNA, 15 pmoles oligo (dT) primers, 10 mM dNTPs, 20 units of RNase inhibitor and 200 units of RevertAid M-MuLV Reverse Transcriptase. The cDNA was used as a template for RT-PCR amplification to analyse transcript level. Actin was used as an internal standard. The primers that were used to amplify the genes are written in parentheses, Mn-SOD (sense: 5’-CAA GGT CCT TGA GCT CGG G-3’ and antisense: 5’-AGC ACC ACC AGA CCT ACG TGA-3’), CAT (sense: 5’-TCC GCG GGG TCC ATG GTC TG-3’ and antisense: 5’-CGC CCG TCA TCG TGC GGT TC-3’), APX (sense: 5’-ACT TCT CAG CAT AGG GGC GGA AC-3’ and antisense: 5’-GAC GTG TCG TAC GCC GAC CT-3’); Actin (sense: 5’-CCC ATT GAG CAC GGT ATT G-3’ and antisense: 5’-GTG GTG GTG AAC GAG TAG CC-3’). All the primers were synthesized (Bangalore Genei, India). The PCR products were electrophoresed on a 1% agarose gel stained with ethidium bromide and viewed using a BIORAD Gel DocTM XR+ system (Singapore).
DNA extraction and analysis
The DNA was extracted according to the method of Saghai-Maroof et al. (Citation1984) and LoSchiavo et al. (Citation2000), with some modifications. An aliquot of preheated (60ºC) hexadecyltrimethyl ammonium bromide (CTAB) isolation buffer (2% w/v CTAB, 1.4 M NaCl, 0.2% v/v b-mercaptoethanol, 20 mM EDTA, 100 mM Tris-HCl, pH 8.0) was added to the cells. After incubation at 60ºC for 40 min, DNA was extracted with chloroform–isoamyl alcohol (24:1 v/v) and centrifuged at 1030 ×g for 15 min at room temperature (RT). The upper aqueous phase was mixed with cold isopropanol (2/3 volume) and centrifuged at 22 500 ×g for 20 min at RT. The nucleic acid pellet was washed with 70% (v/v) ethanol, dried, re-suspended in TE (10 mM Tris-HCl, pH 7.4, 1 mM EDTA) containing 0.1 µg ml−1 RNase A and incubated at 37ºC for 2 h. The DNA was resolved in 1% agarose gels containing ethidium bromide and viewed using the BIORAD Gel DocTM XR+ system (Singapore).
Caspase-3-like activity
Caspase-3-like activity was measured as per the Caspase 3 Assay kit (Sigma, USA) the 2× 108 cells ml−1 of C. reinhardtii, both treated/untreated were collected at various time-points (1, 3, 6, 12, 18 and 24 h). Cells were harvested by centrifugation at 1100×g for 5 min at 25°C, washed in 1× phosphate buffered saline (PBS) and centrifuged at 5000×g for 5 min at 4°C. They were then incubated in chilled lysis buffer (supplied in the kit) on ice for 20 min and centrifuged at 16 000×g for 20 min at 4°C to precipitate cellular debris. Clear lysates were used for measuring caspase-3-like activity. Specific activity was expressed as nanomoles of pNA released per mg of protein per min. For determination of the fold increase in activity, the ratios of the caspase-3-like activity of treated and untreated cells from four independent experiments were calculated and means calculated. Additionally, four independent experiments were performed to show that the caspase inhibitor Ac-DEVDCHO was able to abrogate caspase-3-like activity in H2O2 treated cells.
Determining the mitochondrial membrane potential
Cell cultures were exposed to 10 mM H2O2, 200 mM NaCl and 360 mM sorbitol for different time points (1, 3, 6 and 24 hours). Mitochondrial membrane potential was determined using mitochondrial transmembrane potential apoptosis detection kit according to the manufacturer’s protocol (Abcam, USA). Approximately 1×105 cells were spread on polyethyleneamine (PEI)-coated slides and incubated at room temperature for 15 minutes. Then, the slides were dipped in methanol to remove chlorophyll. Mitocapture reagent (1 µl ml−1) was added and incubated at 37ºC for 20 minutes, followed by washing with incubation buffer. The cells were then observed under 100× magnification using a Nikon Eclipse 90i microscope (Melville, NY, USA).
Cleavage of PARP-1 like enzyme
PARP-1 cleavage was determined according to the manufacturer’s protocol (Abcam, USA). Briefly, cells were pelleted, solubilized (2×107 cells ml−1) in 1× extraction buffer and incubated on ice for 20 min, centrifuged and the supernatant was added into the well and incubated for 2 hours. After washing with 1× wash buffer, detector antibody was added and incubated for 1 h. The mixture was then incubated with 1× HRP, developed with 3,3′,5,5′-tetramethylbenzidine (TMB) a development solution and the absorption read at 600 nm using Infinite® 200 PRO- Tecan (Männedorf, Switzerland).
Terminal deoxynucleotidyl transferase-mediated dUTP nick end labelling (TUNEL) staining
For the in situ detection of nuclear fragmentation, a modified protocol of Danon & Gallois (Citation1998) was used. A 0.5 × 108 cells ml‒1, both treated/untreated cells, were transferred to polyethyleneimine (PEI)-coated slides. Cells were fixed with freshly prepared 4% paraformaldehyde for 15 min. After washing thoroughly with phosphate buffer (PB) pH 7.4, cells were permeabilized with 0.1% phosphate buffered triton X (PBST) for 15 min at RT. The cells were washed thoroughly with PB and the TUNEL assay was carried out using a TUNEL kit (DeadEnd Colorimetric TUNEL System, Promega Madison, WI, USA). The DNA was stained with 10 mg ml−1 4, 6-diamidino-2-phenylindole (DAPI, Molecular Probes, Eugene, OR, USA) for 30 min. The cells were washed thoroughly with PB and viewed under a fluorescence microscope (Nikon Eclipse 90i, Melville, NY, USA), with UV for 4’,6’-diamino-2-phenylindole (DAPI) detection and a green filter for fluorescein isothiocyanate (FITC).
RESULTS
Effect of H2O2, NaCl and sorbitol on cell death
Chlamydomonas reinhardtii cells treated independently with different concentrations of H2O2 (0–50 mM) NaCl and sorbitol (0–800 mM) exhibited a rise in Evans blue retention as a function of dose and time. The signal was significantly above the background and reflected a trend of progressive cellular damage, leading to death, with an increasing dose of stress and incubation time (3–24 h; ). Marginal (~9%) death was observed in the control cells, whereas cells treated for 24 h with 50 mM H2O2 showed highest death (82%; ). Cells exposed to 800 mM NaCl for 24 h caused ~76% cell death (), while cells exposed to 800 mM sorbitol for 24 h showed ~ 40% cell death (). Under both oxidative and osmotic stress conditions, a concomitant increase in cell death was observed with increasing time-point and dose of exposure (); this ranged from 30–82% for H2O2, 25–76% for NaCl and 21–40% for sorbitol after 24 hours. Further, concentrations of stress agents were selected based on the quantitative results obtained in this assay as well as those that have been followed by researchers in the field (Affenzeller et al., Citation2009; 200 mM NaCl at 24 h). With 200 mM NaCl at 24 h, maximum death of 60% was observed (). Moreover, in other plants and algal species this concentration (200 mM) was reported to induce PCD. Hence, in the present study 200 mM NaCl was used for subsequent experiments to check other PCD hallmarks. In comparison, maximum death was observed at 24 h when cells were exposed to H2O2. Hence, the dose and time-point chosen for H2O2 was at 10 mM and 6 h where the death observed was 50%.
Fig. 1. Effect of H2O2, NaCl and sorbitol on Chlamydomonas reinhardtii cell death. Cell death was monitored using the Evans blue dye exclusion assay. The absorbance values recovered for heat-treated cells (100% death) was taken as positive control and percentage death for H2O2, NaCl and sorbitol-exposed cells were calculated. Data are means of three independent experiments ± SE.
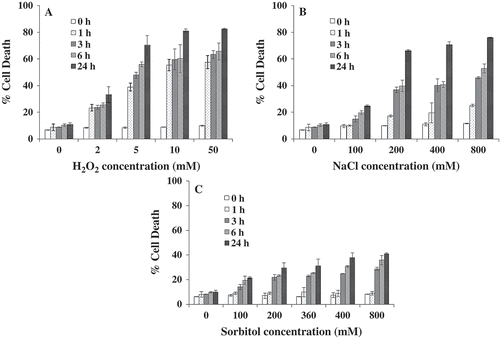
Intracellular H2O2 content after H2O2, NaCl and sorbitol exposures
It is now well-established that both oxidative and osmotic stress conditions lead to the production of ROS (Pallavi et al., Citation2012) in several cells. Therefore, production of intracellular ROS (H2O2) was measured in C. reinhardtii cells after independent exposure to H2O2, NaCl and sorbitol. After 15 min treatment with 10 mM H2O2, the content of H2O2 was significantly (P < 0.01) higher (~2.2 fold) than that of the control (). While, for individual exposure to 200 mM NaCl and 360 mM sorbitol, a significant increase in the intracellular H2O2 content was observed after 1 h. The increase over the control with NaCl- and sorbitol-treated cells was ~1.4 and 1.2, respectively (). Relative to the control cells, the content of H2O2 always remained higher until 24 h of H2O2, NaCl and sorbitol treatments (). Such increases in the cellular H2O2 are known to invoke the defence machinery that activates antioxidant enzymes. Therefore, the enzymatic activities and transcripts of three antioxidant enzymes were then measured.
Effect of oxidative and osmotic stress agents on antioxidant enzyme activity and analyses of their transcript levels
Activities of antioxidant enzymes in C. reinhardtii substantially changed when the cells were exposed to both oxidative and osmotic stress agents. Within 1 h of exposure to 10 mM H2O2, a 5-fold increase in the Mn-SOD activity was observed, whereas a treatment of 200 mM NaCl and 360 mM sorbitol experienced a gradual increase from 1 h until 24 h, with a maximum of 4.5-fold and 3-fold increase at 24 h (). When the catalase activity was measured, cells treated with 10 mM H2O2, showed 10-fold increase after 1 h of exposure, whereas 200 mM NaCl and 360 mM sorbitol showed a gradual increase from 3 h until 24 h with a maximum of 9.8-fold and 5-fold increase at 24 h (). Upon measuring the activity of another antioxidant enzyme such as APX, the pattern of activity was similar to that of catalase (). However, the peak activity of both the enzymes differed ().
Fig. 3. Effect of H2O2, NaCl and sorbitol on antioxidant gene expression and enzyme activity. (A) SOD activity (B) CAT activity (C) APX activity in Chlamydomonas reinhardtii vegetative cells exposed/unexposed to H2O2 and NaCl, independently. Data are the means of three independent experiments ± SE. (D, E & F) Effect of H2O2, NaCl and sorbitol on the transcript abundance of Mn-SOD, CAT and APX in C. reinhardtii. The cells were independently treated with H2O2 and NaCl at 1, 3, 6, 12, 18 and 24 h; total RNAs were extracted from the cells and RT-PCR assays were performed. Actin was used for cDNA normalization.
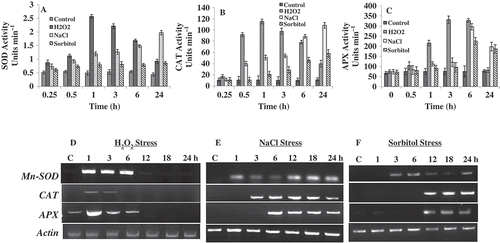
To determine if oxidative and osmotic stress were able to regulate the expression of genes responsible for the antioxidation process, an RT-PCR-based assay was performed. Our analyses showed that, when compared with the control cells collected at 24 h, expression of all the genes coding for Mn-SOD, CAT and APX were up-regulated under H2O2, NaCl and sorbitol stress (). In case of H2O2, the transcript levels of Mn-SOD, CAT and APX showed a progressive increase up to 6 h, after which it gradually dissipated (). However, for NaCl and sorbitol the transcript levels of all the enzymes increased progressively up to 24 h (). It was observed that in all cases, the transcript levels correlated with the enzymatic activities and the intracellular response to H2O2 stress was faster and higher than that of the osmotic stress ().
Genomic DNA laddering after exposure to stress
In order to check whether the ensuing death is apoptotic or necrotic, occurrence of DNA laddering was performed after H2O2, NaCl and sorbitol treatments, at different time points (3–24 h). In the case of 10 mM H2O2 characteristic DNA laddering was observed after 6 h (). However, with different concentrations of NaCl (100–400 mM) for different time points (1–24 h), characteristic DNA laddering was not observed, but 800 mM NaCl after 24 h exposure showed a complete smearing of the genomic DNA (), indicating a non-apoptotic and probably a necrotic form of death in C. reinhardtii post NaCl stress. However, DNA from cells exposed to different concentrations of sorbitol (100–800 mM) at different time points, did not fragment nor smear the DNA during the 24 h period ().
Fig. 4. Effect of H2O2, NaCl and sorbitol on degradation of total genomic DNA of Chlamydomonas reinhardtii. (A) Time-dependent effect on DNA laddering after exposure to 10 mM H2O2. (B) Effect of ZnSO4 on DNA laddering: C. reinhardtii cells were pre-treated for 7 h at two different concentrations of ZnSO4 and then exposed to 10 mM H2O2 for 6 h. The genomic DNA from such cells was electrophoresed for checking the inhibition of the DNA ladder. (C) Dose-dependent effect of NaCl after exposing cells for 24 h. Note maximum shearing of genomic DNA at 800 mM NaCl. (D) Dose-dependent effect of sorbitol after exposing cells for 24 h.
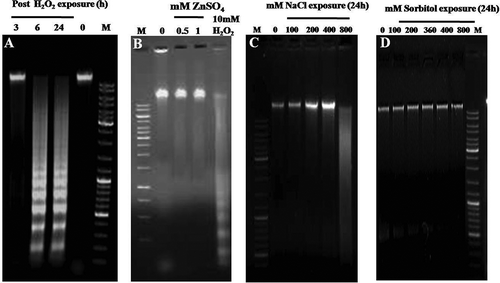
DNA laddering in stressed cells was prevented by pre-treating the cells with 0.5 mM and 1 mM Zn2+ treatment for 7 h and then exposed to 10 mM H2O2 for 6 h (). Subsequent work was therefore targeted at resolving the death types for the H2O2-, NaCl- and sorbitol-exposed cells.
Change in mitochondrial membrane potential
In the control/healthy cells, the cationic dye, mitocapture, accumulated and aggregated in the mitochondria, giving off a bright red fluorescence (). H2O2-treated samples exhibited intense green and weak red/orange fluorescence. This signal started from 1 h in the case of H2O2 exposure, with maximum intensity at 3h (). Hence, the disruption of mitochondrial membrane potential occurs very rapidly with H2O2 stress. Also, 200 mM NaCl showed altered mitochondrial membrane potential at 24 h as indicated by bright green fluorescence (). Conversely, cells treated with 360 mM sorbitol showed red fluorescence, as in the controls ().
Fig. 5. Effect of H2O2, NaCl and sorbitol on mitochondrial membrane potential in Chlamydomonas reinhardtii. The control cells are fluorescing red indicating healthy and intact mitochondria (A); the cells treated with 10 mM H2O2 showed gradual increase in green fluorescence from 1 h till 3 h indicating altered mitochondrial membrane potential (D). Cells treated with 200 mM NaCl also showed altered mitochondrial membrane potential at 24 h (F) while sorbitol-treated cells are fluorescing red (H). Scale bar is 10 μm.
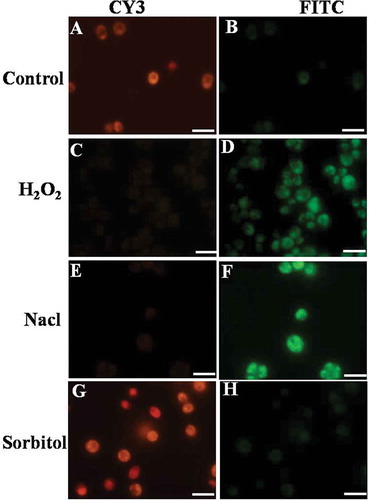
Caspase-3-like activity
Cells treated with 10 mM H2O2 showed increased caspase-3-like activity from 1 h onward until 18 h, but the activity gradually dissipated after 3 h (). However, in the cells pretreated for 1 h with a specific inhibitor of the mammalian caspase-3, Ac-DEVD-CHO, the caspase-3-like activity showed significant uniform inhibition at all time-points (P < 0.05). However, 200 mM NaCl and 360 mM sorbitol-stressed cells failed to show any caspase activity during the 24 h period (data not shown).
Fig. 6. Caspase-3-like activity in Chlamydomonas reinhardtii. Data represent the values of caspase-3-like activity with or without inhibitor (Ac-DEVD-CHO) in Chlamydomonas calculated from three independent experiments ± SE.
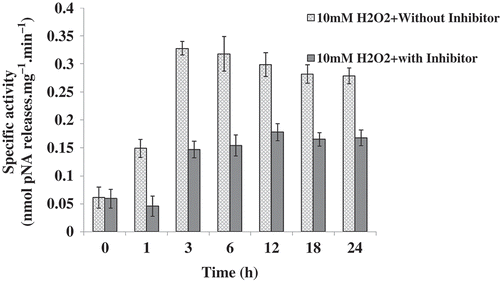
Taken together, the results obtained with DNA laddering/smearing and caspase activation/inactivation confirmed the presence as well as the involvement of a caspase-dependent PCD pathway operating in the H2O2-treated cells and a probable necrotic-like cell death in NaCl-treated cells of C. reinhardtii.
PARP-1 cleavage
Subsequent to the observed increase in the caspase-3-like activity, PARP-1 was the next enzyme in the line of activation. It was therefore assayed in H2O2-treated cells and the PARP-1 cleavage was expressed in relative per cent value. Chlamydomonas reinhardtii cells exposed to 10 mM H2O2 showed maximum PARP-1 cleavage (614 relative per cent value) after 6 h, after which the levels started to decline ().
Detection of TUNEL-positive nuclei
The nicks of nuclear DNA caused due to H2O2 exposure can be detected by the incorporation of fluorescein 12-dUTP at free 3′-OH ends in DNA of the samples. Almost all the control cells exhibited TUNEL-negative nuclei (). However, the cells treated with 10 mM H2O2 started showing TUNEL-positive nuclei after 1 and 3 h treatment, respectively (). To confirm the position of the nucleus, both control and treated cells were stained with DAPI. It is noteworthy that the nuclei of control cells stained clearly with DAPI (), whereas the TUNEL-positive nuclear region (DAPI-stained) in the cells exposed to either H2O2 always coincided with DAPI-positive region (). However, 200 mM NaCl and 360 mM sorbitol stressed cells did not show any DNA nicks during the 24 h period (, ).
DISCUSSION
When monitored for death by the Evans blue dye exclusion method, the H2O2-induced death overrode that of the osmotic-induced death (). The pattern of cell death observed was directly dependent on the stress concentration and exposure time, with high cell death (~41–82%; ) observed after 24 h. Previous studies also showed similar patterns of cell death with H2O2 and osmotic stress (Houot et al., Citation2001; Casolo et al., Citation2005; Gechev & Hille, Citation2005; Gechev et al., Citation2006; de Pinto et al., Citation2006; Darehshouri et al., Citation2008; Affenzeller et al., Citation2009; Sun et al., Citation2012). Since cell death was observed with increasing stress exposure of the cells, it was proposed that this death could be either apoptotic or necrotic. For a better understanding of oxidative and osmotic stress effects on algae, more investigations on the biochemical or molecular aspects are needed.
Reactive oxygen species such as O2−•, H2O2, or OH• are constantly generated as by-products via metabolism, predominantly in chloroplasts, mitochondria and peroxisomes (Apel & Hirt, Citation2004). Under normal physiological conditions, ROS production is well controlled by the antioxidant enzymes present in several organelles (del Río et al., Citation2006). However, under stress conditions, when excess ROS are produced, the risks of serious cellular damage may arise. These excess ROS affect many cellular functions through modifying nucleic acids, oxidizing proteins and inducing lipid peroxidation (Mittler et al., Citation2004; Akça & Samsunlu, Citation2012). Earlier reports have shown that both oxidative and osmotic stress conditions induce ROS production [H2O2 and superoxide radicals (O2-)] (Singh et al., Citation2007; Affenzeller et al., Citation2009; Abogadallah et al., Citation2010) that in turn play an important role in PCD (Darehshouri et al., Citation2008; Zuo et al., Citation2012; Sirisha et al., Citation2014). The accumulation of ROS destroys the cellular components, such as DNA, protein and lipids (López et al., Citation2006). Therefore, as H2O2 is the primary molecule causing PCD in several organisms (Giannattasio et al., Citation2005; Criddle et al., Citation2006; Loor et al., Citation2010; Sharma et al., 2012), it was decided to quantify the production of this molecule upon stress exposure. The present study showed the significantly increased level of ROS in the cells exposed to 10 mM H2O2 () compared with those exposed to 200 mM NaCl and 360 mM sorbitol. The same phenomenon was observed when C. reinhardtii cells were exposed to another oxidative stress agent such as menadione (Sirisha et al., Citation2014). Taken together, and when compared with NaCl and sorbitol, it is the oxidative stress that produced the highest amount of intracellular H2O2 that was measured in this assay ().
It has been reported for a long time that the oxidative- and osmotic stress-induced ROS is counteracted by an intrinsic antioxidant system in plants (Elbaz et al., Citation2010) and involves a collection of enzymatic scavengers that include SOD, the first line of defence against O2−• which converts O2−• to O2 and H2O2 (Alscher et al., Citation2002), CAT and APX are considered the most dominant enzymes that catalyse H2O2 to H2O or the other non-toxic products (Nakano & Asada, Citation1981; Mittler et al., Citation2004; del Río et al., Citation2006; Elbaz et al., Citation2010). Our results indicate that enzymatic activities and transcript levels of SOD, CAT and APX were enhanced in the alga when exposed to 10 mM H2O2, 200 mM NaCl and 360 mM sorbitol () suggesting that SOD, CAT and APX in coordination can remove ROS. Earlier reports also suggested that both oxidative and osmotic stress conditions increase the activities of various antioxidant enzymes (Yoshida et al., Citation2003, Citation2004; Vega et al., Citation2006; Murik & Kaplan, Citation2009; Urzica et al., Citation2012; Guangfeng et al., Citation2012; Murik et al., Citation2014). To check whether the cell death and ROS production induced by oxidative and osmotic stress agents was apoptotic or non-apoptotic, some hallmarks of apoptosis were then recorded.
One of the classical hallmarks of PCD in animals and plants is DNA laddering (Danon et al., Citation2004), but not all the plant cells produce DNA ladders in PCD (Azad et al., Citation2008). Oxidative stress-induced cells showing clear fragmentation of DNA have been reported in previous studies; for example, increased oxidative stress induced apoptosis in mammalian cells (Kim et al., Citation2007; Singh et al., Citation2007; Rottner et al., Citation2011). H2O2 of about 250 µM causes cell membrane leakage and DNA damage in human colon cells (Wijeratne et al., Citation2005). In higher multicellular plants such as barley, tobacco and Arabidopsis, DNA fragmentation pattern was evident with H2O2 stress (Wang et al., Citation1996a, b; Krishnamurthy et al., Citation2000; Malerba et al., Citation2003). This has also been the case among unicellular eukaryotes such as Micrasterias (Darehshouri et al., Citation2008) and Chlamydomonas (Murik & Kaplan, Citation2009). Similarly in the present study, cells exposed to 10 mM H2O2 showed fragmentation of DNA into a ladder from 6 h () until 24 h. It has been known for a long time that Zn2+ can prevent Ca2+-dependent endonucleases which are involved with not only apoptosis (Torriglia et al., Citation1997) but also plant PCD (Sugiyama et al., Citation2004). Our results showed a clear reduction of DNA degradation after H2O2 stress by Zn2+ pre-treatment and the level of DNA degradation was reduced much more strongly by 1 mM than by 0.5 mM Zn2+ (). This indicated that the process of DNA fragmentation was operated by Ca2+-dependent endonucleases. This result also showed that the death of C. reinhardtii by H2O2 was an initiative PCD, i.e. in accordance with other oxidative stress agents inducing PCD (Moharikar et al., Citation2006; Darehshouri et al., Citation2008; Zuo et al., Citation2012; Sirisha et al., Citation2014). Additionally, osmotic stress-induced DNA fragmentation was also reported in plants such as Thellungiella halophila, tobacco, rice, peppermint (Andronis et al., Citation2010; Wang et al., Citation2010; Yazdani & Mahdieh, Citation2012; Li et al., Citation2014; Monetti et al., Citation2014) and unicellular eukaryotes such as Micrasterias denticulata (Affenzeller et al., Citation2009). However, in the present study, different concentrations (100–400 mM) of NaCl-treated cells have not shown any distinctive DNA ladder, although at 800 mM NaCl, a clear genomic DNA smear was observed (). This is unlike Micrasterias (Affenzeller et al., Citation2009) and plants such as Thellungiella halophila (Wang et al., Citation2010) and tobacco (Monetti et al., Citation2014) where 200 mM of NaCl induced PCD showing the characteristic DNA ladder. We therefore conclude that the DNA smear produced by exposure of C. reinhardtii to 800 mM of NaCl might be a characteristic feature of necrotic-like cell death (; Cobb et al., Citation1996). On the other hand, different concentrations of sorbitol (100–800 mM) at different time points (1–24 h) have neither led to DNA fragmentation nor DNA shearing (). Hence, it is demonstrated that the ionic rather than the osmotic component of salt stress (NaCl) causes necrosis in Chlamydomonas.
Another early event that occurs following induction of apoptosis in metazoans is the alteration of mitochondrial membrane potential (MMP) (Kallio et al., Citation2005; Shrivastava et al., Citation2006). Hunter et al. (Citation1976) who first characterized the mitochondrial permeability transition (MPT), reported that the onset of MPT has been implicated as a key mechanism underlying both necrosis and apoptotic cell death (Kim et al., Citation2003). Cells that have disrupted Δψm are irreversibly bound to undergo death, even when the apoptosis trigger is withdrawn (Kim et al., Citation2003). Thus, MMP marks the point-of-no return of the cell death process. Mitochondrial permeability transition is the destruction of transmembrane potential of the mitochondrial inner membrane (Haworth & Hunter, Citation1979; Hunter & Haworth, Citation1979a, b). MPT through oxidative phosphorylation leads to the failure of the proton gradient and stops ATP generation. In response to stimuli such as alkaline pH, ROS, increased intracellular Ca2+ and inorganic phosphate, the permeability transition (PT) pores open in the mitochondrial inner membrane (Zong & Thompson, Citation2006). The physiological function of the PT pore core complex remains elusive. A cell cannot generate ATP by oxidative phosphorylation, as long as the PT pore is open. The opening of the PT pore has been proposed to intensify apoptosis by releasing mitochondrial apoptogenic factors. Our results clearly indicate that H2O2 induces an alteration in MMP, as evidenced by the monomeric form of the mitocapture dye that remains in the cytoplasm (). Altered mitochondrial membrane potential has been correlated to an increase of ROS (Shrivastava et al., Citation2006). Such an alteration in MMP upon H2O2 exposure has been clearly demonstrated in animals and plants (Jian-Ming et al., Citation2003; Li et al., Citation2003; Yin et al., Citation2005; Ma & Yang, Citation2006; Cole et al., Citation2010; He et al., Citation2013). It is also reported that mitochondrial dysfunction is a critical part of the necrotic pathway (Choi et al., 2009). Earlier reports showed that continuous MPT opening leads to necrotic cell death (Marzo et al., Citation1998; Narita et al., Citation1998; Pastorino et al., Citation1998). Our results with 200 mM NaCl also showed altered MMP that is evidenced by bright green fluorescence of the mitocapture dye that remained in the cytoplasm (). MPT is hence very crucial to apoptosis and necrosis, depending on the bioenergetic condition (Kim et al., Citation2003; Yang et al., Citation2007). The cascade of events post mitochondrial membrane potential alteration is the release of cytochrome c from mitochondria and formation of apoptosome in classical PCD.
It has been reported earlier that elevated cytosolic Ca2+ and oxidative stress both contribute to the opening of the mitochondrial permeability transition pore (PTP), which depolarizes the mitochondria and leads to mitochondrial swelling and subsequent release of cytochrome c from the intermembrane space (Goldstein et al., Citation2000). Cytochrome c normally functions as part of the respiratory chain but when released into the cytosol (as a result of PTP opening), it becomes an important component of the apoptosis execution machinery, where it activates caspases (cysteine aspartate proteases) and (if ATP is available) causes apoptotic cell death (Thornberry & Lazebnik, Citation1998; Krause & Durner, Citation2004). In the present study, a commercial mouse anti-cytochrome c antibody was used to detect C. reinhardtii cytochrome c in two independent assays, viz. immunoblotting and ELISA. However, we were unable to detect cytochrome c in both these assay conditions. Furthermore, formation of apoptosome activates caspase-3 enzyme, another key molecule of PCD. Also, studies on experimental models suggested that activated caspase cleaves a host of cellular substrates leading to apoptosis. Previous reports in plants and unicellular algae could detect the presence of caspase-like protein or their activity (del Pozo & Lam, Citation1998; Segovia et al., Citation2003; Moharikar et al., Citation2006; Zuppini et al., Citation2007; Darehshouri et al., Citation2008; Yordanova et al., Citation2013). Our results with H2O2 stress also show the activation of a caspase-3-like protein (). Activation of caspases by H2O2 has been reported in animals, plants and unicellular eukaryotes (Bortner & Cidlowski, Citation1999; Yamakawa et al., Citation2000; Jiang et al., Citation2001; Cai et al., Citation2008; Darehshouri et al., Citation2008), but with 200 mM NaCl and 360 mM sorbitol in our study, no caspase activation was detected (data not shown). Similarly in Micrasterias, with salt and osmotic stress decreased caspase-3-like activity was briefly observed which then went back almost to control level (Affenzeller et al., Citation2009). However, there have been reports to the contrary in some plants that suggest the involvement of a plant caspase-like activity after exposure to salt stress (Andronis et al., Citation2010; Wang et al., Citation2010; Zuppini et al., Citation2010).
Caspase-mediated apoptotic cell death is achieved through the cleavage of several proteins required for cellular functioning and survival (Fischer et al., Citation2003); PARP-1 being one of them (Tewari et al., Citation1995). Proteolytic activity in plant cells undergoing PCD has also been studied using poly(ADP-ribose) polymerase (PARP), a well-characterized substrate for human caspase-3. Endogenous PARP cleavage occurs during menadione-induced PCD (Sun et al., Citation1999b; Sirisha et al., Citation2014); H2O2-induced PCD (Amor et al., Citation1998; Bakondi et al., Citation2002; Zákány et al., 2007) and in heat-shock-treated tobacco suspension cells (Tian et al., Citation2000). Having used the anti-PARP-1 from humans for our assays, we have also shown that it cross-reacts to a PARP-1-like enzyme from C. reinhardtii. Our results clearly demonstrated the cleavage of PARP-1-like protein into 84 kDa fragment (), indicating a caspase-activated PARP-1-mediated PCD-like cell death in this alga.
PCD was then verified by the formation of DNA nicks detected by the TUNEL assay, a technique for specific in situ labelling of the 3’-OH nicks of DNA that are triggered by endonucleases during PCD (Kressel & Groscurth Citation1994). An increase in the TUNEL-positive cells in the H2O2-treated population as compared with the control was found (), thereby suggesting a PCD-like process in these cells. Such TUNEL-positive nuclei have also been reported earlier with oxidative and osmotic stress-induced apoptosis (Singh et al., Citation2007; Darehshouri et al., Citation2008; Yazdani & Mahdieh, Citation2012). However, both 200 mM NaCl- and 360 mM sorbitol-treated cells did not show DNA nicks (, ), suggesting that osmotic stress leads to a necrotic-like-cell death. It has been shown recently that High Mobility Group Box-1 protein, HMGB-1 is secreted when metazoan cells undergo necrosis. HMGB-1 remains bound in apoptotic cells that undergo secondary necrosis. This release of HMGB-1 from mammalian necrotic cells may be detected by immunoblotting (Krysko et al., Citation2008). Preliminary attempts to detect the HMGB-1 protein in the NaCl stressed cell-free supernatants by Western blotting did not yield any cross-reactive polypeptide. Since the primary antibody used in this experiment was anti-human, cross-reactive epitopes might be absent in its C. reinhardtii homologue.
In summary, the current study has demonstrated unequivocally that Chlamydomonas reinhardtii vegetative cells upon oxidative (H2O2) stress showed PCD hallmarks such as DNA fragmentation, disruption of mitochondrial membrane potential, activation of caspase-3-like proteins and cleavage of PARP-1-like enzyme. The appearance of these changes was accompanied by an active metabolism measured by cell death assay and ROS production probably mediated via the Fenton reaction pointing towards a programmed cell death ().
Fig. 9. Schematic representation of PCD and probable necrotic-like cell death pathways in Chlamydomonas reinhardtii.

It is ambiguous as to whether the observed effects of H2O2 stress are direct or indirect. When H2O2 is added to the C. reinhardtii culture containing TAP medium, the reagent has a high likelihood of directly interacting either with the FeSO4/CuSO4 present in the trace elements of the TAP medium or with the transition metals (such as Fe or Cu) present in the C. reinhardtii cell wall, thereby leading to the formation of highly toxic hydroxyl radicals via the Fenton reaction (Rodrigo-Moreno et al., Citation2013). It is then plausible that these radicals might reduce the cytosolic K+ content (Demidchik et al., Citation2010) thereby activating caspase-like proteases (as shown in the current study). The scenario with NaCl stress is different and unique. While there is significant ROS production that alters the mitochondrial membrane potential, unlike other plant species, no caspase activity nor DNA laddering was observed when cells are exposed to 200 mM NaCl. Instead, when cells are stressed with higher concentrations of NaCl, they showed sheared genomic DNA, indicating a necrotic-like mode of cell death pathway. As the iso-osmotic sorbitol treatment does not result in these effects, it is the ionic, instead of the osmotic component of salt stress that leads to necrosis in Chlamydomonas (). Taken together, these and previous results suggest that Chlamydomonas cells are sensitive to oxidative stress such as UV-C (Moharikar et al., Citation2006), menadione (Sirisha et al., Citation2014) and H2O2 and might be able to cope better with low concentrations of osmotic stress. A probable reason could be that Chlamydomonas is naturally more exposed to high salt conditions than oxidative stress (Ramos et al., Citation2004), tuning it to reciprocate accordingly. Moreover, higher NaCl concentrations might be inducing a form of necrotic-like cell death. The physiological, biochemical and molecular changes observed in Chlamydomonas cells during salt stress-induced PCD differ in several features from those described after H2O2 induction. The distinctive morphologies during cell death (including PCD) probably reflect different ways in which cells may die (Morel & Dangle, Citation1997). We conclude that C. reinhardtii offers a simple model system for future studies to genetically analyse the mechanistic machinery that governs cell-to-cell communication while undergoing programmed cell death and necrosis, and our present study paves a way in that direction.
ACKNOWLEDGEMENTS
This work was supported by the Department of Atomic Energy, India.
Additional information
Notes on contributors
Sirisha L. Vavilala
Jacinta S. D’Souza- concept and editing manuscript Sirisha L. Vavilala- performed experiments (ROS production, Transcript abundance, MMP, caspase3-like activity and TUNEL assay) and drafted manuscript. Kanak K Gawde- performed Evans blue cell death assay and DNA laddering Mahuya Sinha- performed and analyzed antioxidant enzymes activity and PARP cleavage assay.
Kanak K. Gawde
Jacinta S. D’Souza- concept and editing manuscript Sirisha L. Vavilala- performed experiments (ROS production, Transcript abundance, MMP, caspase3-like activity and TUNEL assay) and drafted manuscript. Kanak K Gawde- performed Evans blue cell death assay and DNA laddering Mahuya Sinha- performed and analyzed antioxidant enzymes activity and PARP cleavage assay.
Mahuya Sinha
Jacinta S. D’Souza- concept and editing manuscript Sirisha L. Vavilala- performed experiments (ROS production, Transcript abundance, MMP, caspase3-like activity and TUNEL assay) and drafted manuscript. Kanak K Gawde- performed Evans blue cell death assay and DNA laddering Mahuya Sinha- performed and analyzed antioxidant enzymes activity and PARP cleavage assay.
Jacinta S. D’Souza
Jacinta S. D’Souza- concept and editing manuscript Sirisha L. Vavilala- performed experiments (ROS production, Transcript abundance, MMP, caspase3-like activity and TUNEL assay) and drafted manuscript. Kanak K Gawde- performed Evans blue cell death assay and DNA laddering Mahuya Sinha- performed and analyzed antioxidant enzymes activity and PARP cleavage assay.
REFERENCES
- Abogadallah, G.M., Serag, M. & Quick, W.P. (2010). Fine and coarse regulation of reactive oxygen species in the salt tolerant mutants of barnyard grass and their wild type parents under salt stress. Physiologia Plantarum, 138: 60–73.
- Aebi, H. (1984). Catalase in vitro. Methods in Enzymology, 105: 121–126.
- Affenzeller, M.J., Darehshouri, A., Andosch, A., Lütz, C. & Lütz-Meindl, U. (2009). Salt stress-induced cell death in the unicellular green alga Micrasterias denticulata. Journal of Experimental Botany, 60: 939–954.
- Akça, Y. & Samsunlu, E. (2012). The effect of salt stress on growth, chlorophyll content, proline and nutrient accumulation, and K/Na ratio in walnut. Pakistan Journal of Botany, 44: 1513–1520.
- Alscher, R.G., Erturk, N. & Heath, L.S. (2002). Role of superoxide dismutases (SODs) in controlling oxidative stress in plants. Journal of Experimental Botany, 53: 1331–1341.
- Ameisen, J.C., Idziorek,T., Billaut-Mulot, O., Loyens, M., Tissier, J-P., Potentier, A. & Ouaissi, A. (1995). Apoptosis in a unicellular eukaryote (Trypanozoma cruzi): implications for the evolutionary origin and role of programmed cell death in the control of cell proliferation, differentiation and survival. Cell Death and Differentiation, 2: 285–300.
- Amor, Y., Babiychuk, E., Inzé, D. & Levine, A. (1998). The involvement of poly(ADP-ribose) polymerase in the oxidative stress responses in plants. FEBS Letter, 440: 1–7.
- Andronis, E.A. & Roubelakis-Angelakis, K.A. (2010). Short-term salinity stress in tobacco plants leads to the onset of animal-like PCD hallmarks in planta in contrast to long-term stress. Planta, 231: 437–48.
- Apel, K. & Hirt, H. (2004). Reactive oxygen species: metabolism, oxidative stress, and signal transduction. Annual Review of Plant Biology, 55: 373–399.
- Azad, A.K., Ishikawa, T., Sawa, Y. & Shibata, H. (2008). Intracellular energy depletion triggers programmed cell death during petal senescence in tulip. Journal of Experimental Botany, 59: 2085–2095.
- Aziz, I. & Khan, M.A. (2001). Effect of seawater on the growth, ion content and water potential of Rhizophora mucronata Lam. Journal of Plant Research, 114: 369–373.
- Bakondi, E., Bai, P., Szabó, É., Hunyadi, J., Gergely, P., Szabó, C. & Virág, L. (2002). Detection of poly(ADP-ribose) polymerase activation in oxidatively stressed cells and tissues using biotinylated NAD substrate. Journal of Histochemistry and Cytochemistry, 50: 91–98.
- Beauchamp, C.H. & Fridovixh, I. (1971). Superoxide dismutase: improved assays and an assay applicable to acrylamide gels. Analytical Biochemistry, 44: 276–287.
- Bidle, K.D. & Falkowski, P.G. (2004). Cell death in planktonic, photosynthetic microorganisms. Nature Reviews of Microbiology, 2: 643–655.
- Bidle, K.A., Haramaty, L., Baggett, N., Nannen, J. & Bidle, K.D. (2010). Tantalizing evidence for caspase-like protein expression and activity in the cellular stress response of Archaea. Archives of Environmental Microbiology, 12: 1161–1172.
- Bohnert, H.J., Su, H. & Shen, B. (1999). Molecular mechanisms of salinity tolerance. In Molecular Responses to Cold, Drought, Heat, and Salt Stress in Higher Plants (K. Shinozaki & K. Yamaguchi-Shinozaki, editors), pp. 29–62. R.G. Landes Company, Austin, TX.
- Bortner, C.D. & Cidlowski, J.A. (1999). Caspase independent/dependent regulation of K +, cell shrinkage, and mitochondrial membrane potential during lymphocyte apoptosis. Journal of Biological Chemistry, 274: 21953–21962.
- Boyer, J.S. (1982). Plant productivity and environment. Science, 218: 443–448.
- Cai, L., Wang, H., Li, Q., Qian, Y. & Yao, W. (2008). Salidroside inhibits H2O2-induced apoptosis in PC12 cells by preventing cytochrome c release and inactivating of caspase cascade. Acta Biochimica Biophysica Sinica, 40: 796–802.
- Casolo, V., Petrussa, E., Krajňáková, J., Macrì, F. & Vianello, A. (2005). Involvement of the mitochondrial K+ATP channel in H2O2- or NO- induced programmed death of soybean suspension cell cultures. Journal of Experimental Botany, 56: 997–1006.
- Chen, J., Shiyab, S., Han, F.X., Monts, D.L., Waggoner, C.A., Yang, Z.M. & Su, Y. (2009). Bioaccumulation and physiological effects of mercury in Pteris vittata and Nephrolepis exaltata. Ecotoxicology, 18: 110–121.
- Chen, Z., Newman, I., Zhou, M., Mendham, N., Zhang, G. & Shabala, S. (2005). Screening plants for salt tolerance by measuring K+ flux: a case study for barley. Plant Cell Environment, 28: 1230–1246.
- Chen, Z., Cuin, T.A., Zhou, M., Twomey, A., Naidu, B.P. & Shabala, S. (2007). Compatible solute accumulation and stress-mitigating effects in barley genotypes contrasting in their salt tolerance. Journal of Experimental Botany, 58: 4245–4255.
- Choi, K., Kim, J., Kim, G.W. & Choi, C. (2009). Oxidative stress-induced necrotic cell death via mitochondria-dependent burst of reactive oxygen species. Curr Neurovasc Res., 6: 213–222.
- Cobb, J.P., Hotchkiss, R.F., Karl, I.E. & Buchman. T.G. (1996). Mechanisms of cell injury and death. British Journal of Anaesthesia, 77: 3–10.
- Cole, N.B., Daniels, M.P., Levine, R.L. & Kim, G. (2010). Oxidative stress causes reversible changes in mitochondrial permeability and structure. Experimental Gerontology, 45: 596–602.
- Criddle, D.N., Gillies, S., Baumgartner-Wilson, H.K., Jaffar, M., Chinje, E.C., Passmore, S., Chvanov, M., Barrow, S., Gerasimenko, O.V., Tepikin, A.V., Sutton, R. & Petersen, O.H. (2006). Menadione-induced reactive oxygen species generation via redox cycling promotes apoptosis of murine pancreatic acinar cells. Journal of Biological Chemistry, 281: 40485–40492.
- Cuin, T.A., Betts, S.A., Chalmandrier, R. & Shabala, S. (2008). A root’s ability to retain K+ correlates with salt tolerance in wheat. Journal of Experimental Botany, 59: 2697–2706.
- Cuin, T. A., Zhou, M., Parsons, D. & Shabala, S. (2011). Genetic behaviour of physiological traits conferring cytosolic K+/Na+ homeostasis in wheat. Plant Biology, 14: 438–446.
- Danon, A. & Gallois, P. (1998). UV-C radiation induced apoptotic like changes in Arabidopsis thaliana. FEBS Letter, 437: 131–136.
- Danon, A., Rotari, V.I., Gordon, A., Mailhac, N. & Gallois, P. (2004). Ultraviolet-C overexposure induces programmed cell death in Arabidopsis, which is mediated by caspase-like activities and which can be suppressed by caspase inhibitors, pp35 and Defender against apoptotic death. Journal of Biological Chemistry, 279: 779–787.
- Darehshouri, A., Affenzeller, M. & Lütz, M.U. (2008). Cell death upon H2O2 induction in the unicellular green alga Micrasterias. Plant Biology, 10: 732–45.
- Das, M., Mukherjee, S.B. & Shaha, C. (2001). Hydrogen peroxide induces apoptosis like death in Leishmania donovani promastigotes. Journal of Cell Science, 114: 2461–2469.
- de Pinto, M.C., Paradiso, A., Leonetti, P. & De Gara, L. (2006). Hydrogen peroxide, nitric oxide and cytosolic ascorbate peroxidase at the crossroad between defence and cell death. Plant Journal, 48: 784–95.
- del Pozo, O. & Lam, E. (1998). Caspases and programmed cell death in the hypersensitive response of plants to pathogens. Current Biology, 8: 1129–1132.
- del Río, L.A., Sandalio, L.M., Corpas, F.J., Palma, J.M. & Barroso, J.B. (2006). Reactive oxygen species and reactive nitrogen species in peroxisomes, production, scavenging, and role in cell signaling. Plant Physiology, 141: 330–335.
- Delledone, M., Zeier, J., Marocco, A. & Lamb, C, (2001). Signal interactions between nitric oxide and reactive oxygen intermediates in the plant hypersensitive disease resistance response. Proceedings of the National Academy of Sciences USA, 98: 13454–13459.
- Demidchik, V., Cuin, T. A., Svistunenko, D., Smith, S.J., Miller, A.J., Shabala, S., Sokolik, A. & Yurin, V. (2010). Arabidopsis root K+-efflux conductance activated by hydroxyl radicals: single-channel properties, genetic basis and involvement in stress-induced cell death. Journal of Cell Science, 123: 1468–1479.
- Dimova, E.G., Bryant, P.E. & Chankova, S.G. (2008). Adaptive response – some underlying mechanisms and open questions. Genetics and Molecular Biology, 31: 396–408.
- Drew, M.C., He, C.J. & Morgan, P.W. (2000). Programmed cell death and aerenchyma formation in roots. Trends in Plant Science, 5: 123–127.
- Durand, P.M., Rashidi, A. & Michod, R.E. (2011). How an organism dies affects the fitness of its neighbours. American Naturalist, 177: 224–232.
- Elbaz, A., Wei, Y.Y., Meng, Q., Zheng, Q. & Yang, Z.M. (2010). Mercury-induced oxidative stress and impact on antioxidant enzymes in Chlamydomonas reinhardtii. Ecotoxicology, 19: 1285–1293.
- Fahrenkrog, B., Sauder, U. & Aebi, U. (2004). The S. cerevisiae HtrA-like protein Nma111p is a nuclear serine protease that mediates yeast apoptosis. Journal of Cell Science, 117: 115–126.
- Fischer, U., Janicke, R.U. & Schulze-Osthoff, K. (2003). Many cuts to ruin: a comprehensive update of caspase substrates. Cell Death and Differentiation, 10: 76–100.
- Franklin, D.J. & Berges, J.A (2004). Mortality in cultures of the dinoflagellate Amphidinium carterae during culture senescence and darkness. Proceedings of the Royal Society B: Biological Science, 271: 2099–2107.
- Franklin, D.J., Brussaard, C.P.D. & Berges, J.A. (2006). What is the role and nature of programmed cell death in phytoplankton ecology? European Journal of Phycology, 41: 1–14.
- Garthwaite, A.J., von Bothmer, R. & Colmer, T.D. (2005). Salt tolerance in wild Hordeum species is associated with restricted entry of Na+ and Cl– into the shoots. Journal of Experimental Botany, 56: 2365–2378.
- Gechev, T.S. & Hille, J. (2005). Hydrogen peroxide as a signal controlling plant programmed cell death. Journal of Cell Biology, 168: 17–20.
- Gechev, T.S., Van Breusegem, F., Stone, J.M., Denev, I. & Laloi, C. (2006). Reactive oxygen species as signals that modulate plant stress responses and programmed cell death. Bioessays, 11: 1091–1101.
- Giannattasio, S., Guaragnella, N., Corte-Real, M., Passarella, S. & Marra, E. (2005). Acid stress adaptation protects Saccharomyces cerevisiae from acetic acid-induced programmed cell death. Gene, 354: 93–98.
- Goldstein, J.C., Waterhouse, N.J., Juin, P., Evan, G.I., and Green, D.R. (2000). The coordinate release of cytochrome c during apoptosis is rapid, complete and kinetically invariant. Nature Cell Biology, 2: 156–162.
- Guangfeng, K., Cuijan, S., Xiaofei, W., Qiuju, X., Min, W., Xinlei, W. & Jinlai, M. (2012). Acclimatory responses to high-salt stress in Chlamydomonas (Chlorophyta, Chlorophyceae) from Antarctica. Acta Oceanologica Sinica, 31: 116–124.
- Hanikenne, M. (2003). Chlamydomonas reinhardtii as a eukaryotic photosynthetic model for studies of heavy metal homeostasis and tolerance. New Phytologist, 159: 331–340.
- Hardwick, J.M. & Cheng, W.C. (2004). Mitochondrial programmed cell death pathways in yeast. Developmental Cell, 7: 630–632.
- Harris, E.H. (2001). Chlamydomonas as a model organism. Annual Review of Plant Physiology and Plant Molecular Biology, 52: 363–406.
- Haworth, R.A. & Hunter, D.R. (1979). The Ca2+-induced membrane transition in mitochondria. II. Nature of the Ca2+ trigger site. Archives of Biochemistry and Biophysics, 195: 460–467.
- He, X.Q., Hui, J., Xi-long, H. & Qiao-ling, L. (2013). Adverse effect of H2O2 change on morphology, mitochondrial membrane permeability and antioxidant enzyme in root of Dianthus chinensis L. under salt stress. Advance Journal of Food Science and Technology, 5: 445–448.
- Hema, R., Senthil-Kumar, M., Shivakumar, S., Chandrasekhara Reddy, P. & Udayakumar, M. (2007). Chlamydomonas reinhardtii, a model system for functional validation of abiotic stress responsive genes. Planta, 226: 655–670.
- Holler, N., Zaru, R., Micheau, O., Thome, M., Attinge, A., Valitutti, S., Bodmer, J.L., Schneider, P., Seed, B. & Tschopp, J. (2000). Fas triggers an alternative, caspase-8-independent cell death pathway using the kinase RIP as effector molecule. Nature Immunology, 1: 489–495.
- Houot, V., Etienne, P., Petitot, A-S., Barbier, S., Blein, J-P. & Suty, L. (2001). Hydrogen peroxide induces programmed cell death features in cultured tobacco BY-2 cells, in a dose dependent manner. Journal of Experimental Botany, 52: 1721–1730.
- Huh, G.H., Damsz, B., Matsumoto, T.K., Muppala, P.R., Rus, A.M., Ibeas, J.I., Meena, L. Narasimhan, R., Bressan, A. & Hasegawa, P.M. (2002). Salt causes ion disequilibrium-induced programmed cell death in yeast and plants. Plant Journal, 29: 649–659.
- Hunter, D.R. & Haworth, R.A. (1979a). The Ca2+-induced membrane transition in mitochondria. I. The protective mechanisms. Archives of Biochemistry and Biophysics, 195: 453–459.
- Hunter, D.R. & Haworth, R.A. (1979b). The Ca2+-induced membrane transition in mitochondria. III. Transitional Ca2+ release. Archives of Biochemistry and Biophysics, 195: 468–477.
- Hunter, D.R., Haworth, R.A. & Southard, J.H. (1976). Relationship between configuration, function, and permeability in calcium-treated mitochondria. Journal of Biological Chemistry, 251: 5069–5077.
- Jackson, M.B. & Armstrong, W. (1999). Formation of aerenchyma and the processes of plant ventilation in relation to soil flooding and submergence. Plant Biology, 1: 1438–8677.
- Jiang, D., Jha, N., Boonplueang, R. & Andersen, J.K. (2001). Caspase 3 inhibition attenuates hydrogen peroxide-induced DNA fragmentation but not cell death in neuronal PC12 cells. Journal of Neurochemistry, 76: 1745–1755.
- Jianga, Z.Y., Woollarda, A.C.S. & Wolff, S.P. (1990). Hydrogen peroxide production during experimental protein glycation. FEBS Letter, 268: 69–71.
- Jian-Ming, L., Qian, H.Z. & Xiao, C.G-X. (2003). Role of mitochondrial dysfunction in hydrogen peroxide-induced apoptosis of intestinal epithelial cells. World Journal of Gastroenterology, 9: 562–567.
- Kallio, A., Zheng, A., Dahllund, J., Heiskanen, K.M. & Härkönen, P. (2005). Role of mitochondria in tamoxifen-induced rapid death of MCF-7 breast cancer cells. Apoptosis, 10: 1395–1410.
- Khona, D.K., Rao, V.G., Motiwalla, M.J., Varma, P.C., Kashyap, A.R., Das, K., Shirolikar, S.M., Borde, L., Dharmadhikari, J.A., Dharmadhikari, A.K., Mukhopadhyay, S., Mathur, D. & D’Souza, J.S. (2013). Anomalies in the motion dynamics of long-flagella mutants of Chlamydomonas reinhardtii. Journal of Biological Physics, 39: 1–14.
- Kim, J-S., He, L. & Lemasters, J.J. (2003). Mitochondrial permeability transition: a common pathway to necrosis and apoptosis. Biochemical and Biophysical Research Communications, 304: 463–470.
- Kim, Y.A., Xiao, D., Xiao, H., Powolny, A.A., Lew, K.L., Reilly, M.L., Zeng, Y., Wang, Z. & Singh, S.V. (2007). Mitochondria mediated apoptosis by diallyl trisulfide in human prostate cancer cells is associated with generation of reactive oxygen species and regulated by Bax/Bak. Molecular Cancer Therapeutics, 6: 1599–1609.
- Kirschnek, S., Scheffel, J., Heinzmann, U. & Häcker, G. (2004). Necrosis-like cell death induced by bacteria in mouse macrophages. European Journal of Immunology, 34: 1461–1471.
- Kolodkin-Gal, I., Hazan, R., Gaathon, A., Carmeli, S. & Engelberg-Kulka, H. (2007). A linear pentapeptide is a quorum-sensing factor required for mazEF-mediated cell death in Escherichia coli. Science, 318: 652–655.
- Krause, M. & Durner, J. 2004. Harpin activates mitochondria in Arabidopsis suspension cells. American Phytopathological Society, 2: 131–139.
- Kressel, M. & Groscurth, P. (1994). Distinction of apoptotic and necrotic cell death by in situ labeling of fragmented DNA. Cell and Tissue Research, 278: 549–556.
- Krishnamurthy, K.V., Krishnaraj, R., Chozhavendan, R. & Samuel, F.C. (2000). The program of cell death in plants and animals – a comparison. Current Science, 79: 1169–1181.
- Krysko, D.V., Vanden Berghe, T., Parthoens, E., D’Herde, K. & Vandenabeele, P. (2008). Methods for distinguishing apoptotic from necrotic cells and measuring their clearance. Methods in Enzymology, 442: 307–341.
- Lee, N., Berthelot, S., Debrabant, A., Muller, J., Duncan, R. & Nakhasi, H.L. (2002). Programmed cell death in the unicellular protozoan parasite Leishmania. Cell Death and Differentiation, 9: 53–64.
- Leist, M. & Jaattela, M. (2001). Four deaths and a funeral: from caspases to alternative mechanisms. Nature Reviews Molecular Cell Biology, 2: 589–598.
- Lewis, K. (2000). Programmed death in bacteria. Microbiology and Molecular Biology Reviews, 64: 503–514.
- Li, J.M., Zhou, H., Cai, Q. & Xiao, G.X. (2003). Role of mitochondrial dysfunction in hydrogen peroxide-induced apoptosis of intestinal epithelial cells. World Journal of Gastroenterology, 9: 562–567.
- Li, J.Y., Jiang, A.L. & Zhang, W. (2007). Salt stress-induced programmed cell death in rice root tip cells. J. Integr. Biol, 49: 481–486.
- Li, Z., Yang, H., Wu, X., Guo, K. & Li, J. (2014). Some aspects of salinity responses in peppermint (Mentha x pipertia L.) to NaCl treatment. Protoplasma, Nov 12. [Epub ahead of print].
- Lin, J., Wang, Y. & Wang, G. (2005). Salt stress-induced programmed cell death via Ca2+-mediated mitochondrial permeability transition in tobacco protoplasts. Plant Growth Regulation, 4: 243–250.
- Lin, J., Wang, Y. & Wang, G. (2006). Salt stress-induced programmed cell death in tobacco protoplasts is mediated by reactive oxygen species and mitochondrial permeability transition pore status. Journal of Plant Physiology, 163: 731–739.
- Logan, D.C. (2006). The mitochondrial compartment. Journal of Experimental Botany, 57: 1225–43.
- López, E., Arce, C., Oset-Gasque, M.J., Cañ adas, S. & González, M.P. (2006). Cadmium induces reactive oxygen species generation and lipid peroxidation in cortical neurons in culture. Free Radical Biology and Medicine, 40: 940–951.
- Loor, G., Kondapalli, J., Schriewer, J.M., Chandel, N.S., Vanden Hoek, T.L. & Schumacker, P.T. (2010). Menadione triggers cell death through ROS-dependent mechanisms involving PARP activation without requiring apoptosis. Free Radical Biology and Medicine, 49: 1925–36.
- LoSchiavo, F., Baldan, B., Compagnin, D., Ganz, R., Mariani, P. & Terzi, M. (2000). Spontaneous and induced apoptosis in embryogenic cell cultures of carrot (Daucus carota L.) in different physiological states. European Journal of Cell Biology, 79: 294–298.
- Ma, H.Y. & Yang, H.Q. (2006). The effect of exogenous H2O2 on mitochondrial membrane permeability and cell nuclear DNA in roots of Malus hupehensis. Journal of Plant Physiology and Molecular Biology, 32: 551–556.
- Madeo, F., Engelhardt, S., Herker, E., Lehmann, N., Maldener, C., Proksch, A., Wissing, S. & Frohlich, K.U. (2002). Apoptosis in yeast: a new model system with applications in cell biology and medicine. Current Genetics, 41: 208–216.
- Mahajan, S. & Tuteja, N. (2005). Cold, salinity and drought stresses: an overview. Archives of Biochemistry and Biophysics, 444: 139–158.
- Malerba, M., Cerana, R. & Crosti, P. (2003). Fusicoccin induces in plant cells a programmed cell death showing apoptotic features. Protoplasma, 222: 113–6.
- Marzo, I., Brenner, C., Zamzami, N., Jurgensmeier, J.M., Susin, S.A., Vieira, H.L., Prevost, M.C., Xie, Z., Matsuyama, S., Reed, J.C. & Kroemer, G. (1998). Bax and adenine nucleotide translocator cooperate in the mitochondrial control of apoptosis. Science, 281: 2027–2031.
- Mastrobuoni, G., Irqanq, S., Pietzke, M., Assmus, H.E., Wenzel, M., Schulze, W.X. & Kempa, S. (2012). Proteome dynamics and early salt stress response of the photosynthetic organism Chlamydomonas reinhardtii. BMC Genomics, 31: 13: 215.
- Miller, G., Suzuki, N., Ciftci-Yilmaz, S. & Mittler, R. (2010). Reactive oxygen species homeostasis and signalling during drought and salinity stresses. Plant Cell and Environment, 33: 453–467.
- Mittler, R., Vanderauwera, S., Gollery, M. & Van Breusegem, F. (2004). Reactive oxygen gene network of plants. Trends in Plant Science, 9: 490–498.
- Moharikar, S., D’Souza, J.S., Kulkarni, A.B. & Rao, B.J. (2006). Apoptotic-like cell death pathway is induced in unicellular chlorophyte Chlamydomonas reinhardtii (Chlorophyceae) cells following UV irradiation: detection and functional analysis. Journal of Phycology, 42: 423–433.
- Moharikar, S., D’Souza, J.S. & Rao, B.J. (2007). A homologue of the defender against the apoptotic death gene (dad1) in UV-exposed Chlamydomonas cells is down regulated with the onset of programmed cell death. Journal of Biosciences, 32: 261–270.
- Monetti, E., Kadono, T., Tran, D., Azzarello, E., Arbelet-Bonnin, D., Biligui, B., Briand, J., Kawano, T., Mancuso, S. & Bouteau, F. (2014). Deciphering early events involved in hyperosmotic stress-induced programmed cell death in tobacco BY-2 cells. Journal of Experimental Botany, 65: 1361–1375.
- Moradas-Ferreira, P., Costa, V., Piper, P. & Mager, W. (1996). The molecular defences against reactive oxygen species in yeast. Molecular Microbiology, 19: 651–658.
- Morel, J.B. & Dangl, J.L. (1997). The hypersensitive response and the induction of cell death in plants. Cell Death and Differentiation, 4: 671–683.
- Murik, O. & Kaplan, A. (2009). Paradoxically, prior acquisition of antioxidant activity enhances oxidative stress-induced cell death. Environmental Microbiology, 11: 2301–2309.
- Murik, O., Elboher, A. & Kaplan, A. (2014). Dehydroascorbate: a possible surveillance molecule of oxidative stress and programmed cell death in the green alga Chlamydomonas reinhardtii. New Phytologist, 202: 471–484.
- Nakano, Y. & Asada, K. (1981). Hydrogen peroxide is scavenged by ascorbate-specific peroxidase in spinach chloroplasts. Plant Cell Physiology, 22: 867–880.
- Narita, M., Shimizu, S., Ito, T., Chittenden, T., Lutz, R.J., Matsuda, H. and Tsujimoto, Y. (1998). Bax interacts with the permeability transition pore to induce permeability transition and cytochrome c release in isolated mitochondria. Proceedings of the National Academy of Sciences USA, 95: 14681–14686.
- Nedelcu, A.M. (2009). Comparative genomics of phylogenetically diverse unicellular eukaryotes provide new insights into the genetic basis for the evolution of the programmed cell death machinery. Journal of Molecular Evoloution, 68: 256–268.
- Nedelcu, A.M., Driscoll, W.W., Durand, P.M., Herron, M.D. & Rashidi, A. (2011). On the paradigm of altruistic suicide in the unicellular world. Evolution, 65: 3–20.
- Pallavi, S., Ambuj, B.J., Rama, S.D. & Mohammad, P. (2012). Reactive oxygen species, oxidative damage, and antioxidative defense mechanism in plants under stressful conditions. Journal of Botany, 1–26. doi:10.1155/2012/217037.
- Pastorino, J.G., Chen, S.T., Tafani, M., Snyder, J.W. & Farber, J.L. (1998). The overexpression of Bax produces cell death upon induction of the mitochondrial permeability transition. Journal of Biological Chemistry, 273: 7770–7775.
- Pérez-Pérez, M.E., Florencio, F.J. & Crespo, J.L. (2010). Inhibition of TOR signalling and stress activate autophagy in Chlamydomonas reinhardtii. Plant Physiology, 152: 1874–1888.
- Ramos, J., Lopez, M.J. & Benlloch, M. (2004). Effect of NaCl and KCl salts on the growth and solute accumulation of the halophyte Atriplex nummularia. Plant and Soil, 259: 163–168.
- Rodrigo-Moreno, A., Poschenrieder, C. & Shabala, S. (2013). Transition metals. A double edge sward in ROS generation and signalling. Plant Signalling and Behaviour, 8: e23425.
- Rottner, M., Tual-Chalot, S., Ahmed, H.M., Andriantsitohaina, R., Freyssinet, J.M. & Martínez, M.C. (2011). Increased oxidative stress induces apoptosis in human cystic fibrosis cells. PLoS ONE, 6: e24880.
- Saghai-Maroof, M.A., Soliman, K.M., Jorgesen, R.A. & Allard, R.W. (1984). Ribosomal DNA spacer-length polymorphisms in barley: Mendelian inheritance, chromosomal location and population dynamics. Proceedings of the National Academy Sciences USA, 81: 8014–8018.
- Segovia, M., Haramaty, L., Berges, J.A., Falkowski, P. G. (2003). Cell death in the unicellular chlorophyte Dunaliella tertiolecta. A hypothesis on the evolution of apoptosis in higher plants and metazoans. Plant Physiology, 132: 99–105.
- Shabala, S. & Pottosin, I. (2014). Regulation of potassium transport in plants under hostile conditions: implications for abiotic and biotic stress tolerance. Phyisologia Plantarum, 151: 257–279.
- Shabala, S., Cuin, T.A., Prismall, L. & Nemchinov, L.G. (2007). Expression of animal CED-9 anti-apoptotic gene in tobacco modifies plasma membrane ion fluxes in response to salinity and oxidative stress. Planta, 227: 189–197.
- Sharma, P., Jha, A.B., Dubey, R.S. & Pessarakli, M. (2012). Reactive oxygen species, oxidative damage, and antioxidative defense mechanism in plants under stressful conditions. J of Bot, doi:10.1155/2012/21703.
- Shemarova, I.V. (2010). Signaling mechanisms of apoptosis-like programmed cell death in unicellular eukaryotes. Comparative Biochemistry and Physiology Part B: Biochemistry and Molecular Bioogy, 155: 341–353.
- Shrivastava, A., Tiwari, M., Sinha, R.A., Kumar, A., Balapure, A.K., Bajpai, V.K., Sharma, R., Mitra, K., Tandon, A. & Godbole, M.M. (2006). Molecular iodine induces caspase-independent apoptosis in human breast carcinoma cells involving hemitochondria-mediated pathway. Journal of Biological Chemistry, 4: 19762–19771.
- Singh, S.V., Choi, S., Zeng, Y., Hahm, E. & Xiao, D. (2007). Guggulsterone-induced apoptosis in human prostate cancer cells is caused by reactive oxygen intermediate-dependent activation of c-Jun NH2-terminal kinase. Cancer Research, 67: 7439–7449.
- Sirisha, V.L., Sinha, M. & D’Souza, J.S. (2014). Menadione-induced caspase-dependent programmed cell death in the green chlorophyte Chlamydomonas reinhardtii. Journal of Phycology, 50: 587–601.
- Smethurst, C.F., Rix, K., Garnett, T., Auricht, G., Bayart, A., Lane, P., Wilson, S.J. & Shabala, S. (2008). Multiple traits associated with salt tolerance in lucerne: revealing the underlying cellular mechanisms. Functional Plant Biology, 35: 640–650.
- Sugiyama, M., Ito, J., Aoyagi, S. & Fukuda, H. (2004). Endonucleases. Plant Molecular Biology, 44: 387–397.
- Sun, L., Yau, H.Y., Wong, W.Y., Li, R.A., Huang, Y. & Yao, X. (2012). Role of TRPM2 in H2O2-induced cell apoptosis in endothelial cells. Plos ONE, 7: e43186. doi:10.1371/journal.pone.0043186.
- Sun, Y.L., Zhu, H.Z., Zhou, J., Dal, Y.R. & Zhai, Z-H. (1999b). Menadione-induced apoptosis and degradation of lamin-like proteins in tobacco protoplasts. Cellular and Molecular Life Sciences, 55: 310–316.
- Tavakkoli, E., Fatehi, F., Coventry, S., Rengasamy, P. & McDonald, G.K. (2011). Additive effects of a+ and Cl– ions on barley growth under salinity stress. Journal of Experimental Botany, 62: 2189–2203.
- Tchernov, D., Kvitt, H., Haramaty, L., Bibby, T.S., Gorbunov, M.Y., Rosenfeld, H. & Falkowski, P.G. (2011). Apoptosis and the selective survival of host animals following thermal bleaching in zooxanthellate corals. Proceedings of the National Academy of Sciences USA, 108: 9905–9909.
- Teixeira de Mattos, M.J. & Neijssel, O.M. (1997). Bioenergetic consequences of microbial adaptation to low-nutrient environments. Journal of Biotechnology, 59: 117–126.
- Tewari, M., Quan, L.T., O’Rourke, K., Desnoyers, S., Zeng, Z., Beidler, D.R., Poirier, G.G., Salvesen, G.S. & Dixit, V.M. (1995). Yama/CPP32 beta, a mammalian homolog of CED-3, is a CrmA-inhibitable protease that cleaves the death substrate poly(ADP-ribose) polymerase. Cell, 81: 801–809.
- Thornberry, N.A. & Lazebnik, Y. 1998. Caspases: enemies within. Science, 281: 1312–1316.
- Tian, R., Zhang, G., Yan, C. & Dai, Y. (2000). Involvement of poly(ADP-ribose) polymerase and activation of caspase-3- like protease in heat shock-induced apoptosis in tobacco suspension cells. FEBS Letter, 474: 11–15.
- Torriglia, A., Chaudun, E., Courtois, Y. & Counis, M.F. (1997). On the use of Zn2+ to discriminate endonucleases activated during apoptosis. Biochimie, 79: 435–438.
- Turan, S. & Tripathy, B.C. (2012). Salt and genotype impact on antioxidative enzymes and lipid peroxidation in two rice cultivars during de-etiolation. Protoplasma, 250: 209–222. doi:10.1007/s00709-012-0395-5.
- Tuteja, N. (2007). Mechanisms of high salinity tolerance in plants. Methods of Enzymology, 428: 419–438.
- Urzica, E.I., Adler, L.N., Page, M., Linster, C.L., Arbing, M.A., Casero, D., Pellegrini, M., Merchant, S.S. & Clarke, S.G. (2012). Impact of oxidative stress on ascorbate biosynthesis in Chlamydomonas via regulation of the VTC2 gene encoding a GDP-L-galactose phosphorylase. Journal of Biological Chemistry, 287: 14234–14245.
- Vardi, A., Berman-Frank, I., Rozenberg, T., Hadas, O., Kaplan, A. & Levine, A. (1999). Programmed cell death of the dinoflagellate Peridinium gatunense is mediated by CO2 limitation and oxidative stress. Current Biology, 9: 1061–1064.
- Vega, J.M., Garbayo, I., Domínguez, M.J. & Vigara, J. (2006). Effect of abiotic stress on photosynthesis and respiration in Chlamydomonas reinhardtii induction of oxidative stress. Enzyme and Microbial Technology, 40: 163–167.
- Vercammen, D., Brouckaert, G., Denecker, G., Van de Craen, M., Declercq, W., Fiers, W. & Vandenabeele, P. (1998). Dual signaling of the Fas receptor: initiation of both apoptotic and necrotic cell death pathways. Journal of Experimental Medicine, 188: 919–930.
- Walter, D., Wissing, S., Madeo, F. & Fahrenkrog, B. (2006). The inhibitor-of-apoptosis protein Bir1p protects against apoptosis in S. cerevisiae and is a substrate for the yeast homologue of Omi/HtrA2. Journal of Cell Science, 119: 1843–1851.
- Wang, H., Li, J., Bostock, R.M. & Gilchrist, D.G. (1996b). Apoptosis: a functional paradigm for programmed plant cell death induced by a host-selective phytotoxin and invoked during development. The Plant Cell, 8: 375–391.
- Wang, J., Li, X., Liu, Y. & Zhao, X. (2010). Salt stress induces programmed cell death in Thellungiella halophila suspension-cultured cells. Journal of Plant Physiology, 167: 1145–1151.
- Wang, M., Oppedijk, B.J., Lu, X., Van Duijn, B. & Schilperoort, R.A. (1996a). Apoptosis in barley aleurone during germination and its inhibition by abscisic acid. Plant Molecular Biology, 32: 1125–1134.
- Wijeratne, S.S.K., Cuppett, S.L. & Schlegel, V. (2005). Hydrogen peroxide induced oxidative stress damage and antioxidant enzyme response in Caco-2 human colon cells. Journal of Agricultural and Food Chemistry, 53: 8768–8774.
- Wissing, S., Ludovico, P., Herker, E., Buttner, S., Engelhardt, S.M., Decker, T., Link, A., Proksch, A., Rodrigues, F., Corte-Real, M., Frohlich, K.U., Manns, J., Cande, C., Sigrist, S.J., Kroemer, G. & Madeo, F. (2004). An AIF orthologue regulates apoptosis in yeast. Journal of Cell Biology, 166: 969–974.
- Witzel, K., Weidner, A., Surabhi, G.K., Börner, A. & Mock, H.P. (2009). Salt stress-induced alterations in the root proteome of barley genotypes with contrasting response towards salinity. Journal of Experimental Botany, 60: 3545–3557.
- Xiong, L. & Zhu, J.K. (2002). Molecular and genetic aspects of plant responses to osmotic stress. Plant Cell and Environment, 25: 131–139.
- Yamakawa, H., Ito, Y., Naganawa, T., Banno, Y., Nakashima, S., Yoshimura, S., Sawada, M., Nishimura, Y., Nozawa, Y. & Sakai, N. (2000). Activation of caspase-9 and -3 during H2O2-induced apoptosis of PC12 cells independent of ceramide formation. Neurological Research, 6: 556–64.
- Yang, P-M., Chen, H-C., Tsai, J-S. & Lin, L-Y. (2007). Cadmium induces Ca2+-dependent necrotic cell death through calpain-triggered mitochondrial depolarization and reactive oxygen species-mediated inhibition of nuclear factor-kB activity. Chemical Research in Toxicology, 20: 406–415.
- Yazdani, M. & Mahdieh, M. (2012). Salinity induced apoptosis in root meristematic cells of rice. International Journal of Bioscience, Biochemistry and Bioinformatics, 2: 40–43.
- Yin, L., Stearns, R. & González-Flecha, B. (2005). Lysosomal and mitochondrial pathways in H2O2-induced apoptosis of alveolar type II cells. Journal of Cell Biochemistry, 94: 433–445.
- Yordanova, Z.P., Kapchina-Toteva, V.M., Woltering, E.J., Cristescu, S.M., Harren, F.J.M. & Iakimova, E.T. (2009). Mastoparan-induced cell death signalling in Chlamydomonas reinhardtii. Biotechnology and Biotechnology Equipment, 23: 730–734.
- Yordanova, Z.P., Iakimova, E.T., Cristescu, S.M., Harren, F.J.M., Kapchina-Toteva, V.M. & Woltering, E.J. (2010). Involvement of ethylene and nitric oxide in cell death in mastoparan-treated unicellular alga Chlamydomonas reinhardtii. Cell Biology International, 34: 301–308.
- Yordanova, P.Z., Woltering, J.E., Kapchina-Toteva, M.V. & Iakimova, E.T. (2013). Mastoparan-induced programmed cell death in the unicellular alga Chlamydomonas reinhardtii. Annals of Botany, 111: 191–205.
- Yoshida, K., Igarashi, E., Mukai, M., Hirata, K. & Miyamoto, K. (2003). Induction of tolerance to oxidative stress in the green alga, Chlamydomonas reinhardtii, by abscisic acid. Plant Cell Environment, 26: 451–457.
- Yoshida, K., Igarashi, E., Wakatsuki, E., Miyamoto, K. & Hirata, K. (2004). Mitigation of osmotic and salt stresses by abscisic acid through reduction of stress-derived oxidative damage in Chlamydomonas reinhardtii. Plant Science, 167: 1335–1341.
- Zákány, R., Bakondi, E., Juhász, T., Matta, C., Szíjgyártó, Z., Erdélyi, K., Szabó, É., Módis, L., Virág, L. & Gergely, P. (2007). Oxidative stress-induced poly(ADP-ribosyl)ation in chick limb bud-derived chondrocytes. International Journal of Molecular Medicine, 19: 597–605.
- Zhu, J. K. (2002). Salt and drought stress signal transduction in plants. Annual Review of Plant Biology, 53: 247–273.
- Zong, W-X. & Thompson, C.B. (2006). Necrotic death as a cell fate. Genes and Development, 20: 1–15.
- Zuo, Z., Zhu, Y., Bai, Y. & Wang, Y. (2012). Acetic acid-induced programmed cell death and release of volatile organic compounds in Chlamydomonas reinhardtii. Plant Physiology and Biochemistry, 51: 175–184.
- Zuppini, A., Andreoli, C. & Balda, B. (2007). Heat stress: an inducer of programmed cell death in Chlorella saccharophila. Plant Cell Physiology, 48: 1000–9.
- Zuppini, A., Gerotto, C. & Baldan, B. (2010). Programmed cell death and adaptation: two different types of abiotic stress response in a unicellular chlorophyte. Plant Cell Physiology, 51: 884–895.