ABSTRACT
UV radiation can affect the physiology of seaweeds and their associated microorganisms. In this study we exposed different Chilean populations of the agarophyte Gelidium lingulatum to PAR or PAR+UV and monitored their photosynthetic performance using in vivo chlorophyll fluorescence for 5 days to determine changes in abundance and composition of epibiotic bacteria. Before the experiment, the seaweeds had been acclimated for more than 1 year in outdoor tanks with flowing seawater. At the beginning of the experiment, maximum quantum yield (Fv/Fm) did not vary among different populations. During the recovery period, Fv/Fm increase was higher in thalli from La Pampilla (30°S) in comparison with other populations (33°S and 42°S). The lowest total amount of bacteria (22.2 ± 2.5 × 103 cell mm–2) on thalli was observed at the beginning of the experiment. At the end of the experiment, the density of bacteria was not significantly different among thalli from different populations, as well as among UV-exposed and non-exposed seaweeds. The bacterial community in seawater was different from that on the seaweed thalli as shown by MiSeq Illumina reads of 16S rRNA. UV exposure changed the composition of microbial communities on the thalli and led to a reduction in community diversity and evenness. Relative abundance of Alteromonas sp. increased but abundances of Methylophaga sp. and Colwellia sp. decreased during UV exposure. Microbes associated with G. lingulatum may play an important ecological role in seaweed-microbial interactions. Future studies should explore the ecological function of microbial communities associated with seaweeds.
Introduction
Any substratum submersed in seawater is quickly colonized by micro- and macro-fouling organisms in a process known as biofouling (Wahl, Citation1989), which on the surface of living organisms is called epibiosis. Bacteria are the most abundant group of microorganisms on the surface of seaweeds (Wahl et al., Citation2012; Hollants et al., Citation2013) and several studies have suggested that the composition of these bacterial communities is unique and differs from those on nearby substrata (Michelou et al., Citation2013; Paix et al., Citation2020; Saha et al., Citation2020). These complex microbial communities on the surface of seaweeds are called holobiomes (Egan et al., Citation2013). It has been suggested that seaweeds can be colonized not only by host-specific bacteria but also by any suitable bacterium present in the environment (Campbell et al., Citation2015; Lemay et al., Citation2018; de Mesquita et al., Citation2019). In this context, the ecology of seaweeds can only be fully understood by investigation of these microbe–seaweed interactions (Egan et al., Citation2013).
The function of bacteria on the surface of seaweeds (commonly known as epibacteria) is not well investigated (but see Wichard, Citation2015; Saha & Weinberger, Citation2019; Longford et al., Citation2019). It is reported that epibiotic bacteria can consume carbon-rich extracellular seaweed products, contributing to the microbial food web and carbon cycling (Michelou et al., Citation2013). Micro- and macro-epibiosis reduce access to light, gas exchange, nutrient uptake and photosynthesis, and can enhance the risk of diseases in seaweeds (Harder, Citation2009; Wahl et al., Citation2012; Singh & Reddy, Citation2014; Florez et al., Citation2017; Hudson et al., Citation2019). On the other hand, bacterial epibionts can provide nutrients and vitamins to seaweeds and produce favourable shading (Dobretsov & Qian, Citation2002; Hepburn & Hurd, Citation2005; Singh et al., Citation2011; Florez et al., Citation2017). Many studies have shown that bacterial isolates from the surface of seaweeds produce bioactive compounds that can prevent biofouling and growth of pathogens on seaweeds (reviewed by Egan et al., Citation2008; Wahl et al., Citation2012; Saha & Weinberger, Citation2019). Additionally, epibiotic bacteria have been associated with the induction of morphogenesis and growth patterns of seaweeds (Matsuo et al., Citation2005; Singh & Reddy, Citation2014).
Seaweeds and their holobiomes constitute a complex functional unit that can be affected by environmental stressors such as increases in temperature, partial pressure of carbon dioxide (pCO2) and metal pollution (Hengst et al., Citation2010; Miranda et al., Citation2013; van der Loos et al., Citation2019). An increase in light intensity can promote photosynthesis of seaweeds or inhibit it if radiation is too strong (Bischof et al., Citation2000). Additionally, changes in solar radiation can affect the defence of seaweeds against microfoulers (Wahl et al., Citation2010). Solar radiation, especially ultra-violet (UV) radiation, and temperature are the main factors driving the distribution, growth and reproductive pattern of seaweeds, and their physiology and photosynthetic activity (Falkowski & LaRoche, Citation1991; Bischof et al., Citation2000; Wiencke et al., Citation2007). Seaweeds have developed different mechanisms, biochemical, physiological and morphological, to deal with solar radiation changes (Hurd et al., Citation2014). For instance, a significant proportion of genes in the transcriptome of the intertidal seaweed Laurencia dendroidea were identified as functioning in DNA replication, recombination and repair, which are important to mitigate negative effects of UV radiation for this species (de Oliveira et al., Citation2012). Biofilms on the surface of seaweeds may serve as a protective cover reducing incoming UV radiation (Wahl et al., Citation2012; Raddatz et al., Citation2017) but these effects have not been experimentally confirmed. Thus, it is important to study how (i) UV radiation affects microbial communities associated with seaweeds, and (ii) whether these microbes mitigate UV effects on seaweeds.
Changes of bacterial epibiotic communities on the surface of seaweeds have been investigated using molecular approaches, such as denaturing gradient gel electrophoresis (DGGE) and restriction fragment polymorphism (RFLP), as well as cloning and hybridization methods (Wahl et al., Citation2010; Lachnit et al., Citation2011; Longford et al., Citation2019). Recently, the massive parallel next generation sequencing technology has been employed to investigate the detailed structure of epibiotic bacterial communities on seaweeds (Stratil et al., Citation2013; Chen & Parfrey, Citation2018; Paix et al., Citation2019; Saha et al., Citation2020). This tool permits in-depth analyses of compositional shifts of epibiotic bacterial communities associated with seaweeds under different environmental conditions (Longford et al., Citation2019; Saha et al., Citation2020).
Here, we examined epibacterial communities of the seaweed Gelidium lingulatum along the Chilean coast. The Chilean coast (18–56°S) is characterized by a latitudinal gradient in solar radiation (Vernet et al., Citation2009) and sea surface temperatures (Ramos-Rodríguez et al., Citation2012). Within this large-scale gradient there is also meso-scale variation (10–100s km) in oceanographic and atmospheric nearshore conditions (Hernández et al., Citation2012; Tapia et al., Citation2014). These environmental conditions generate particular habitat niches for seaweeds with wide geographic distribution ranges (e.g. Véliz et al., Citation2020).
Gelidium lingulatum is a commercially important agarophyte seaweed distributed along the south-east Pacific coast of Chile (Buschmann et al., Citation2001) where it has been reported from the coast of Antofagasta (23°S) to Tierra del Fuego (56°S), although its current distribution does not seem to extend north of 29°S (Ramírez & Santelices, Citation1991; Macaya et al., Citation2016; López et al., Citation2017). Usually, G. lingulatum lives on rocky shores of the mid-low intertidal zone, forming monospecific beds at wave-exposed sites. It is attached to calcareous substrata, such as mussel shells, and to holdfasts of large kelps, such as the bull kelp Durvillaea antarctica (Santelices & Varela, Citation1994; López et al., Citation2017). The wide distribution of G. lingulatum, as well as its high genetic diversity, has been attributed to its common occurrence as a secondary rafter on floating D. antarctica (López et al., Citation2017). During rafting dispersal, microbial communities on G. lingulatum might move together with their host in this complex system (floating rafters and non-floating epibionts), and thus it might be expected that the distribution of specific microbial communities matches that of their host populations.
We hypothesized that UV radiation affects photosynthetic performance of seaweeds, modifies their associated bacterial communities and suppresses the abundance of epibiotic bacteria. To our knowledge, this investigation is the first to study the effect of UV radiation on the microbial communities of seaweeds. This study was conducted on the intertidal seaweed G. lingulatum collected from different locations along the Chilean coast (30–42°S), where slight differences in growth and physiological responses to UV have been observed (Véliz et al., Citation2020). The aims of the study were to: (i) investigate and compare the effect of UV radiation on photosynthetic performance of different G. lingulatum populations from three latitudes (30°S, 33°S, 42°S), (ii) compare densities of bacteria on the thalli of G. lingulatum from the three populations, and (iii) study the effect of UV radiation on the density of bacteria and the composition of epibacterial communities on the surface of G. lingulatum.
Materials and methods
Seaweed collection and culture conditions
Adult thalli of G. lingulatum were collected from three Chilean coastal populations, La Pampilla (29°57′S 71°21′W), Maitencillo (32°37′S 71°25′W), and Mar Brava (41°56′S 74°01′W) during austral summer 2016 (January/February) (). Different haplotypes for mitochondrial COI and chloroplast rbcL markers were detected in separate G. lingulatum populations, indicating low geographic connectivity among them and high genetic diversity (López et al., Citation2017). All collected samples were free from macrofouling organisms, as visible to the naked eye. Values of PAR (μmol photons m–2 s–1) at sampling sites of G. lingulatum were obtained from MODIS satellite data (NASA website http://oceancolor.gsf.nasa.gov/cgi/I3) () and showed significant variation among sites (two-way ANOVA F2,24 = 37.75; p > 0.0001) and between seasons (two-way ANOVA F3,24 = 452.87; p > 0.0001). Samples from the intertidal zone were transported in cool boxes with cooling elements to the Universidad Católica del Norte (UCN) Marine Botany Laboratory, Coquimbo (30°S) within 48 h after collection. Stoloniferous thalli of G. lingulatum composed of several erect axes were suspended over scallop shells () to allow vegetative propagation by creeping axes (Véliz et al., Citation2019). Thalli were acclimated in one 2000 l outdoor tank from February 2016 to July 2017. In this tank, three rectangular containers with shells (one container for each G. lingulatum population) were maintained. The standard cultivation conditions included continuous flow of seawater (2 l min–1) from La Herradura Bay, Coquimbo (29°58’S; next to La Pampilla) and permanent aeration. The outdoor tanks were covered with sun-shading nets to reduce the incident photosynthetically active photon flux density (PPFD) to ~50–150 μmol photons m–2 s–1 from natural sunlight. Additionally, thalli of G. lingulatum were collected in July 2017 from La Pampilla (29°57’S 71°21’W) and used to compare epibacterial communities between acclimated and non-acclimated seaweeds using scanning electron microscopy analysis and MiSeq DNA sequencing (see below).
Fig. 1 Experimental analysis of Gelidium lingulatum: (a) geographic location of sampling sites along the Chilean coast, (b) annual mean values of photosynthetically active radiation (PAR) at seaweed sampling sites, (c) view of G. lingulatum growing on shells during acclimatization to outdoor conditions, (d) view of seaweed thallus where the rectangle indicates the sector used for photosynthesis and bacterial analysis, (e) view of bacterial community on the thallus surface of G. lingulatum using SEM
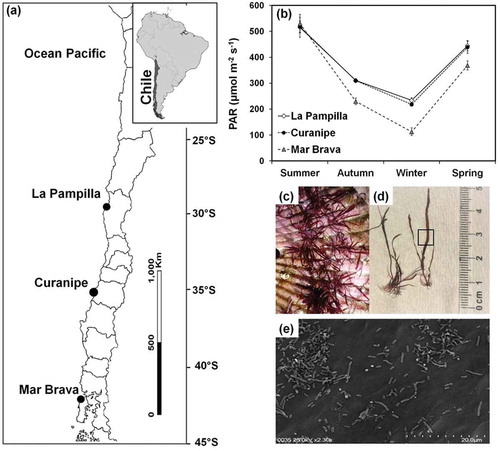
Exposure of Gelidium lingulatum to UV radiation
Prior to the experiment, apical segments of 2–3 cm length (i.e. seaweed samples) were obtained from individual thalli of each population of G. lingulatum (La Pampilla, Maitencillo and Mar Brava) pre-acclimated in the outdoor tank. Seaweed samples were maintained in independent plastic beakers (herein called experimental containers) each containing 350 ml of autoclaved seawater enriched with von Stosch medium (Yokoya & Handro, Citation2002). The seaweed samples were maintained in the experimental containers for 7 days before experimental trials in a temperature-controlled room (14 ± 1°C) with constant aeration, light exposure of 60 ± 5 μmol photons m–2 s–1 and photoperiod of 12:12 day:night. Enriched seawater was refreshed every 2 days.
To investigate the effect of UV radiation on different G. lingulatum populations, experimental containers were exposed to two radiation treatments: PAR (400–700 nm), which was considered as a control, and PAR+UV (280–700 nm). UV light was introduced using two artificial sources of UV irradiance corresponding to the UVA and UVB spectrum, UVB-313 and UVA-340 lamps (Q-Lab Co., Cleveland, Ohio, USA), while PAR was provided by daylight fluorescent lamps (Philips 40 W). Specific cut-off filters were placed above the experimental containers to achieve the experimental treatment settings: a 395 nm cut-off filter (Ultraphan 395, Digefra GmbH, Germany) in the PAR treatment, and a 295 nm cut-off filter (Ultraphan 295, Digefra GmbH, Germany) in the PAR+UV treatment. Three experimental containers (n = 3) per population were used in each radiation treatment. The PAR conditions were generated with a light period from 7:00 to 19:00 h (12:12, day:night photoperiod) at an irradiance of 60 ± 10 μmol photons m–2 s–1. In the UV treatment, UV-lamps were turned on for 2 h per day from 13:00 to 15:00 h, emitting ~2.0 W m–2 of UVB and 7.5 W m–2 of UVA according to Véliz et al. (Citation2006). The PAR irradiances were measured with a LI-190S quantum sensor connected to a LI-250 radiometer (LI-COR Inst., USA), and UV radiation was measured with an UV-sensor (MU-100 UV, Apogee Inst., USA). The experiment was conducted over a period of 5 consecutive days, with the seaweed samples being exposed to one daily pulse of UV radiation. This period of exposure was selected because it resembles natural conditions of solar irradiation of intertidal seaweeds along the central coast of Chile, when low tides occur during spring-summer days (see Wieters et al., Citation2013). After 5 days, the thalli were collected and epibiotic bacteria were analysed (see below).
Determination of photosynthetic performance of seaweeds
The photosynthetic performance of the middle part of G. lingulatum thalli () was measured every day by in vivo chlorophyll fluorescence of photosystem II (PSII) with a portable pulse amplitude modulated fluorometer (PAM 2500, Heinz Walz GmbH, Effeltrich, Germany), following the nomenclature and parameter calculation described by Enríquez & Borowitzka (Citation2010). Maximum quantum yield was calculated as the ratio of variable to maximal fluorescence (Fv/Fm) and was measured in seaweed samples dark adapted for 15 min (from three experimental containers, n = 3). A 5 s far-red light pulse was applied before and after dark acclimation in order to fully oxidize the electron transport chain. Fv/Fm values were registered every day immediately after 2 h of UV exposure, and then 19 h after the end of each UV exposure in order to assess recovery from photoinhibition. Photoinhibition by UV radiation and recovery of seaweeds was compared using two-way analysis of variance (ANOVA) with repeated measurements (between-subject effects = populations, and the within-subject factor = time). The normality of the data was verified using the Shapiro–Wilk’s test and homogeneity of variances was analysed using Levene’s test. Post-hoc Tukey’s HSD-test was used to test for significant differences between treatments. A p value < 0.05 was considered statistically significant.
Epibiotic bacteria on thalli of Gelidium lingulatum
The numbers of cocci and bacilli on thalli of G. lingulatum from different populations (La Pampilla, Maitencillo and Mar Brava) at the beginning and the end of the experiment were analysed using scanning electron microscopy (SEM) (). Additionally, next generation sequencing was used to compare and identify epibiotic bacteria on G. lingulatum collected from La Pampilla at the beginning and end of the experiment. The La Pampilla population was selected based on the results of our experiments, which showed highest potential of UV recovery for that population.
Scanning electron microscopy
Four seaweed samples from each G. lingulatum population were taken at the beginning and the end of the experiment (PAR and PAR+UV treatments). Additionally, four seaweed samples from the field were collected in July 2017 from La Pampilla (29°57′S 71°21′W). All seaweed samples were fixed in 2.5% glutaraldehyde in artificial seawater free of calcium and magnesium, and then dehydrated using an alcohol series, dried in a critical-point dryer (Tousimis, Samdri-780A) using CO2 and coated with gold in a fine coat ion sputter (JEOL JFC-100). The seaweed samples were examined using a scanning electron microscope (SEM, SU3500, Hitachi) at magnification ×1000 (). The total number of bacteria, cocci (round and oval in shape) and bacilli (rod-shaped) were counted in 12 randomly selected fields of view of each thallus. The densities of bacteria were calculated as the mean number of cells per mm2 of thallus surface. To compare the densities of bacteria a two-way analysis of variance (ANOVA) was followed by Tukey’s HSD-test. Prior to the analysis, the normality of the data was verified using the Shapiro-Wilk’s test (Zar, Citation2010).
Composition of microbial communities
Microbial communities were analysed on seaweed samples from the La Pampilla population (29°57′S 71°21′W) at the beginning and end of the experiment (i.e. after exposure to the PAR and PAR+UV treatments), using a MiSeq Illumina sequencing platform with 16S rDNA genes. Three seaweed samples were used for each treatment (n = 3). To make sure that bacteria on the surface of G. lingulatum are not coming from seawater, 10 ml of seawater were collected from seaweed tanks (n = 3) and freeze-dried. To compare microbial communities associated with acclimated and un-acclimated seaweeds (the latter will be termed ‘field’ from here on), additional samples (n = 3) of G. lingulatum were collected from La Pampilla in July 2017.
To remove adhered seawater, seaweed thalli were gently wiped with sterile paper tissue after which they were freeze-dried. Dried seaweed and seawater samples were kept individually at −80°C until the analysis of microbial communities using Illumina MiSeq next generation sequencing. DNA from seaweed samples with associated microbes as well as from seawater was extracted using a DNeasy PowerBiofilm kit (Qiagen, USA) following the manufacturer’s protocol. The purity and concentration of DNA was measured using a Nanodrop (Thermo Scientific, USA).
DNA was sequenced and analysed at the Molecular Research (MR DNA) company (Shallowater, TX, USA). Bacterial V3–V4 hypervariable regions of 16S rRNA genes were sequenced using pair ended (2 × 300) PE Illumina MiSeq platform with the primers 515F (5’-GTGCCAGCMGCCGCGGTAA-3’) and 806R (5’-GGACTACHVGGGTWTCTAAT-3’). Briefly, PCR amplifications were conducted using a single step 30 cycle PCR using the HotStarTaq Plus Master Mix Kit (Qiagen, USA) under the following conditions: 94°C for 3 min, followed by 28 cycles of 94°C for 30 s, 53°C for 40 s and 72°C for 1 min, and a final elongation step at 72°C for 5 min. Sequence data were processed using the MR DNA analysis pipeline (MR DNA, Shallowater, Texas, USA) following the manufacturer’s guidelines. Raw samples were de-multiplexed and barcodes were eliminated (Dowd et al., Citation2008). Sequences were joined, clipped and filtered. Sequences < 150 bp and sequences with ambiguous base calls were removed. Operational Taxonomic Units (OTUs) were generated and sequence chimaeras were removed. OTUs were defined by clustering at 3% divergence and 97% similarity. Chloroplast, mitochondrial and eukaryotic sequences were removed. Final OTUs were taxonomically classified using BLASTn against a curated database derived from RDPII and NCBI (http://rdp.cme.msu.edu, http://ncbi.nlm.nih.gov). Alpha-diversity indices (Shannon and Simpson) and evenness were calculated using the PAST program (Paleontological Statistics, ver. 1.47) with bootstrap of 9999 permutations. Diversity indices were compared using ANOVA followed by a post-hoc LSD test. A Bray–Curtis similarity matrix of abundances of OTUs in microbial communities was constructed (Clarke, Citation2003). Before calculation, the OTU abundances were square-root transformed. Hierarchical agglomerative clustering dendrograms of samples were generated with Primer® software.
Results
Effect of UV exposure on photosynthesis
Fv/Fm did not vary among the populations of G. lingulatum at the beginning of the experiment (one-way ANOVA F2,6 = 0.06; p = 0.943; Supplementary fig. 1, day 0). After 2 h of exposure to PAR+UV, the Fv/Fm decreased in all populations in relation to the control (only PAR; , Supplementary fig. 1). This UV-induced inhibition of Fv/Fm, calculated as the decrease in percentage of the corresponding control, ranged from 35.2 to 57.4% and was significantly higher (p < 0.05) during the first two days of the experiment with no differences among populations (; ).
Table 1. Results of the two-way ANOVA with repeated measurements for the between-subject effects of the factors Population (La Pampilla, Maitencillo and Mar Brava) and the within-subject factor Time (days of experimentation) on: (a) Inhibition of maximal quantum yield (Fv/Fm) after 2 h of exposure, and (b) Fv/Fm under recovery (values as % of control). * indicates significant differences
Fig. 2 Changes in percentages of inhibition and recovery of maximum quantum yield (Fv/Fm) of G. lingulatum populations. (a) UV-induced inhibition of Fv/Fm after 2 h of exposure (calculated as the decrease in per cent of the mean Fv/Fm value of the corresponding control under PAR), and (b) Fv/Fm as percentage of the corresponding control after 19 h of recovery post UV-exposure. Values are mean (n = 3) ± SD. Values that are significantly different (Tukey, ANOVA) between each other at p < 0.05 are indicated by different letters above the bars. Similar values are joined by a horizontal line
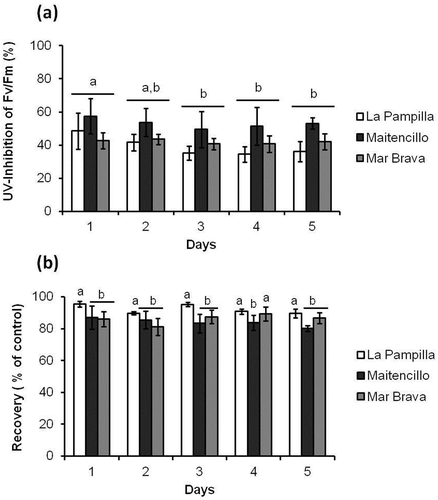
All populations showed an increase in Fv/Fm values during the recovery period, but this Fv/Fm increase was higher in samples from La Pampilla in comparison with Maitencillo and Mar Brava (). There was a significant influence of time of the experiment on the recovery of photosynthetic performance ().
Density of epibiotic bacteria on Gelidium lingulatum
Epibiotic microorganisms on the surface of G. lingulatum were only represented by bacteria (Supplementary fig. 2), with the total number of bacteria on the thalli ranging from 22.2 ± 2.5 × 103 to 96.1 ± 25.6 × 103 cells mm–2 (). The lowest total numbers of bacteria on thalli were recorded at the beginning of the experiment in all populations. During the experiment, the total density of bacteria increased. At the end of the experiment, the total density of bacteria did not differ (p > 0.05) among populations or between UV-exposed (PAR+UV) and non-exposed (PAR) thalli (, ). The total densities of bacteria on the un-acclimated samples collected from La Pampilla (‘field’) were not significantly different from ones on the acclimated seaweeds from the same location exposed and non-exposed to UV (one-way ANOVA F2,2 = 1.25; p = 0.31; ).
Table 2. Results of the two-way ANOVA for the factors Population (La Pampilla, Maitencillo and Mar Brava) and Radiation Condition (initial before start experiment, and after 5 days under PAR and PAR+UV) on densities of bacteria (cell mm–2)
Fig. 3 The densities (cells mm–2) of bacteria on Gelidium lingulatum thalli. Initial – acclimated thalli samples before the experiment. UV – thalli exposed to PAR+UV. Control – thalli exposed to PAR only. Field – field thalli samples. Values are mean ± SD (n = 12). Different letters above the bars indicated significant differences according to the Tukey post hoc test at p < 0.05
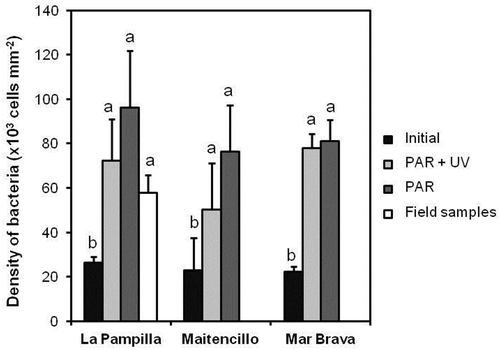
Two main morphological groups of bacteria were observed under SEM: spherical (coccus) and rod-shaped (bacillus) (Supplementary figs. 2, 3). The density of cocci differed significantly (p < 0.05) among seaweeds from different locations and radiation treatments (, Supplementary fig. 3). Seaweeds from La Pampilla had significantly higher coccal densities than Maitencillo samples (Tukey test, p < 0.05, ). The densities of bacilli and cocci were influenced by the UV treatment (). The samples from Maitencillo had lower densities of cocci under UV exposure (PAR+UV) than the control seaweeds and G. lingulatum at the beginning of the experiment (Tukey test, p < 0.05) (Supplementary fig. 3). The density of bacilli was also influenced by the UV treatment, with high densities of bacilli in samples under UV exposure (Supplementary fig. 3).
Composition and diversity of epibiotic bacteria on Gelidium lingulatum
In total, 1 608 067 OTUs were obtained at 97% identity. The highest number of OTUs (140 321) were observed for filed samples of G. lingulatum from La Pampilla. These sequences correspond to seven phyla, 82 classes, 314 families and 686 genera.
Diversity of bacterial communities
Comparison of community composition using a Bray–Curtis similarity matrix revealed clear differences between epibiotic communities on thalli of field and acclimated initial G. lingulatum (). Epibiotic communities on initial G. lingulatum shared less than 60% similarity with communities at the end of the experiment. Communities on G. lingulatum exposed to PAR+UV were slightly (< 20%) different from control G. lingulatum. The bacterial community present in seawater differed from epibiotic communities on seaweeds. Similarity of epibiotic communities between replicates of the same treatment was high (~90%).
Fig. 4 Hierarchical agglomerative clustering based on Bray–Curtis similarity of microbial communities associated with Gelidium lingulatum thalli from La Pampilla before (initial) and after the experiment (UV and control) and present in seawater during the period of the study. Field – microbial communities associated with field seaweeds. Individual replicates denominated by different numbers (1–3)
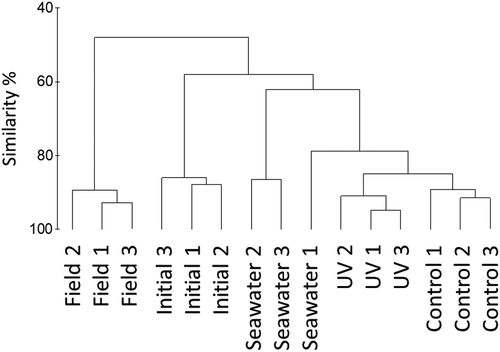
Based on OTUs, G. lingulatum epibiotic communities exposed to PAR+UV had significantly lower Shannon and Simpson diversity indices and evenness (p < 0.05) compared to communities on control and field G. lingulatum (). Diversity of microbial communities on initial G. lingulatum and exposed to PAR+UV was not significantly different (p > 0.05), but high microbial diversity was recorded on field G. lingulatum ().
Table 3. Diversity of microbial communities (mean ± SD) associated with Gelidium lingulatum thalli from La Pampilla before (initial) and after the experiment (UV and control) and present in seawater during the period of the study. Field – microbial communities associated with field thalli. Individual replicates denominated by different numbers (1–3). Different letters indicated significant differences according to the LSD post hoc test at p < 0.05
Composition of microbial communities
Bacteria belonging to the phylum Proteobacteria comprised about 70% of all sequences. The classes Alphaproteobacteria (13–53% of all sequences) and Gammaproteobacteria (17–51% of all sequences) dominated in all samples (). Microbial communities associated with seawater were mainly composed of two classes of bacteria (Alpha- and Gammaproteobacteria). In contrast, Alpha-, Gamma- and Deltaproteobacteria, Flavobacteria and Actinobacteria were dominant on thalli of G. lingulatum (). The class Sphingobacteria was dominant (25% of all sequences) on field G. lingulatum from La Pampilla, but the relative abundance of this class was dramatically reduced during acclimation (). During the experiment, the proportion of Alphaproteobacteria decreased, while the relative abundance of Gammaproteobacteria increased. UV exposure did not cause a significant difference in the relative abundance of any class.
Fig. 5 The main classes of bacteria associated with Gelidium lingulatum from La Pampilla before (S) and after the experiment and present in seawater (SW) during the period of the study. F – microbial communities associated with field thalli. C – microbial communities on control (just PAR). UV – microbial communities on thalli that were exposed to PAR+UV. Data are the mean percentage (n = 3) of OTUs belonging to classes of bacteria
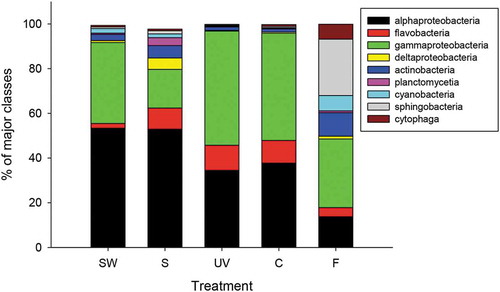
Almost all bacterial genera were present in our samples but in different quantities (). Unidentified species of cyanobacteria (23–34% of total sequences) dominated on thalli of G. lingulatum at the beginning of the study. At the end of the study, the most common OTU (13–45%) on seaweeds were assigned to Alteromonas sp. This genus was more dominant on seaweeds exposed to PAR+UV (> 32%) than on controls only exposed to PAR (13–24%; ). In contrast, the genera Methylophaga (4–6%) and Colwellia (12–19%) dominated on control thalli. Field G. lingulatum had completely different epibiotic microbial communities dominated by Granulosicoccus (23–28%) and Lewinella (10–13%). Sulfitobacter was the most dominant (13–38%) genus in seawater samples.
Fig. 6 The main genera of bacteria (> 5% of total abundance) associated with Gelidium lingulatum from La Pampilla before (S) and after the experiment and present in seawater (SW) during the period of the study. F – microbial communities associated with field thalli. C – microbial communities on control thalli (just PAR). UV – microbial communities on thalli that were exposed to PAR+UV. Different replicates denominated by different numbers
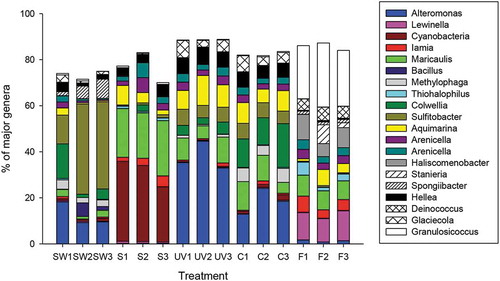
Discussion
The effect of UV radiation on the photosynthetic performance
In this study, we investigated the effect of UV radiation on the photosynthetic performance and associated microorganisms of the red seaweed G. lingulatum from different populations maintained in common conditions prior to the experiment. Thalli from La Pampilla (low latitude) showed higher recovery than thalli from higher latitudes after UV exposure. Genetic analyses of haplotypes identified by COI markers in G. lingulatum revealed no evidence of any strong phylogeographic break between 28°S and 42°S, with populations > 1000 kilometres apart being genetically similar (López et al., Citation2017). However, different haplotypes of G. lingulatum were found at the three studied localities (La Pampilla, Maitencillo and Mar Brava) from 30–42°S (López et al., Citation2017). Similarly, our SEM data showed differences between the compositions of epibacterial communities on G. lingulatum from different locations, even after one year of acclimation in common-garden conditions. The differential physiology of haplotypes, that allows or restricts acclimation to UV, and variation in epibacterial communities that may enhance seaweed UV resistance might explain differences in recovery capacity of populations of G. lingulatum from different latitudes in our study. In contrast, UV-induced inhibition of Fv/Fm was not significantly different among populations of G. lingulatum, perhaps because G. lingulatum grows in the intertidal zone where it is commonly exposed to intensive solar radiation (Montalva & Santelices, Citation1981; Véliz et al., Citation2019). Also, high physiological plasticity has been described for G. lingulatum populations in common culture conditions (Véliz et al., Citation2019, Citation2020). The production of photoprotective metabolites (MAAs) (Huovinen et al., Citation2005; Véliz et al., Citation2019, Citation2020) suggests that G. lingulatum has a high potential to adapt to changes in solar radiation.
Impact of UV radiation on seaweed epibiotic bacteria
Previous studies demonstrated that seaweeds are colonized by diverse microorganisms that can protect them from UV radiation, pathogens and biofouling (Wahl et al., Citation2012; Goecke et al., Citation2013; Campbell et al., Citation2015; Raddatz et al., Citation2017; de Mesquita et al., Citation2019). While the effect of UV radiation on bacteria has been investigated previously (reviewed in Goosen & Moolenaar, Citation2008), including marine bacteria from plankton and neuston (Agogué et al., Citation2005; Santos et al., Citation2011, Citation2012; Manrique et al., Citation2012), there is no information about the impact of UV on bacteria associated with seaweed surfaces.
Scanning electron microscopy (SEM) showed that all examined samples of G. lingulatum thalli had high densities of epibiotic bacteria with no other epibiotic microorganisms. The densities of bacteria observed in this study are in the range of those reported for other species of seaweeds such as Caulerpa racemosa (Dobretsov et al., Citation2006), Fucus vesiculosus (Wahl et al., Citation2010), Asparagopsis armata (Paul et al., Citation2006) and Agarophyton vermiculophyllum (Saha et al., Citation2020). While the total density of bacteria did not differ significantly between treatments at the end of the experiment, the proportions of coccus and bacillus cells were influenced by UV radiation. Similarly, next generation sequencing demonstrated that UV radiation affected the composition of bacteria on the surface of G. lingulatum as shown by cluster analysis and Shannon diversity. One potential explanation for observed differences in bacterial community structure between treatments may be the varied tolerance of bacterial lineages to UV radiation, as previously observed among taxonomic groups from bacterioplankton and bacterioneuston isolates (Santos et al., Citation2011, Citation2012; Manrique et al., Citation2012). Some bacteria have evolved different repair mechanisms to counter the damage induced by UV radiation (Goosen & Moolenaar, Citation2008). The NGS data suggested that the genus Alteromonas sp. was more dominant on G. lingulatum exposed to UV than on non-exposed ones. In a previous study, Alteromonas bacteria had been identified as highly resistant to solar radiation (Agogué et al., Citation2005). In contrast, the genera Methylophaga and Colwellia were dominant on G. lingulatum before experimental exposure to UV radiation. There is not much information about UV resistance of these genera and the role of these bacteria in bacteria–seaweed interactions. Colwellia is a genus of psychrophilic bacteria (Hünken et al., Citation2008) that has been isolated from the brown seaweed Ascophyllum nodosum (Martin et al., Citation2015). Colwellia sp. Arc7-D isolated from Arctic Ocean sediment is a H2O2 resistant species and has 23 genes related to antioxidant activity, which could help to protect this bacterium from UV radiation (Zhang et al., Citation2019).
Epibacterial communities on G. lingulatum
Previous studies have found that Gammaproteobacteria, Alphaproteobacteria and the Cytophaga-Flavobacteria-Bacteroidetes (CFB) group were dominant on the surface of seaweeds (Longford et al., Citation2007; Tujula et al., Citation2010; Hollants et al., Citation2013; Michelou et al., Citation2013; Brodie et al., Citation2016; Selvarajan et al., Citation2019; Saha et al., Citation2020). Actinobacteria were found on brown and red seaweeds, such as Fucus vesiculosus, Delesseria sanguinea and Cystoseira spp. (Goecke et al., Citation2013; Mancuso et al., Citation2016). A study of epibiotic bacteria associated with the Chilean seaweeds Scytosiphon lomentaria, Lessonia nigrescens and Ulva spp. revealed dominance of Alphaproteobacteria (Hengst et al., Citation2010) and the CFB group. While bacteria associated with seaweeds provide associational defence (Wiese et al., Citation2009) and protection against pathogenic bacteria (Saha & Weinberger, Citation2019), as well as affecting the morphology of the thalli (Singh & Reddy, Citation2014) and synthesis of specific metabolites (Selvarajan et al., Citation2019), other functional traits of epibiotic bacteria on seaweeds remain unknown.
Our data support previous assertions that microbial communities associated with seaweeds were different from those found in the environment (Staufenberger et al., Citation2008; Burke et al., Citation2011; Lemay et al., Citation2018; Weigel & Pfister, Citation2019). This suggests that, first, particular bacteria are enriched on the surface of seaweeds, creating unique microbial communities, ‘seaweed holobiomes’, on the surface of different seaweeds (Egan et al., Citation2013; Miranda et al., Citation2013; Aires et al., Citation2015). Second, this finding indicates that observed differences in our experiment are not due to possible contamination of bacteria from seawater.
Changes in epibacterial communities during cultivation
Acclimation of G. lingulatum from La Pampilla prior to the experiment led to significant changes in the associated epibacterial communities. For example, the class Sphingobacteriia (mainly Lewinella sp.) dominated (25%) in un-acclimated samples, while on acclimated individuals this class was rare (1%). Marine bacteria belonging to the genus Lewinella are rod-shaped, non-motile and Gram-negative (Jung et al., Citation2016; Kang et al., Citation2017). This genus is also abundant on thalli of the red seaweed Porphyra umbilicalis with no known function (Miranda et al., Citation2013). Observed changes in epibacterial communities of acclimated and un-acclimated seaweeds were probably due to changes in seawater temperature, circulation, nutrients and solar radiation, similar to what has been suggested for the epibacterial community from the giant kelp Macrocystis pyrifera (Florez et al., Citation2019).
Changes in microbial communities during cultivation of seaweeds have been reported before. Microbial communities in seawater of an aquaculture farm changed significantly during cultivation of Undaria pinnatifida (Zhao et al., Citation2018). Moreover, environmental factors, such as temperature, oxygen and nitrogen concentrations, also shaped the structure of microbial communities (Zhao et al., Citation2018). Salinity and duration of the experiment affected richness of epibacterial communities of the red seaweed Agarophyton vermiculophyllum (Saha et al., Citation2020). Similarly, microbial communities on the red seaweed Porphyra umbilicalis differed between winter and autumn seasons (Miranda et al., Citation2013). Seasonal differences were also observed in the epibacterial community of the kelp M. pyrifera from northern Chile (Florez et al., Citation2019). Future studies should consider this season- or condition-dependent variability and examine the role and ecological function of microbial communities associated with G. lingulatum.
Our study suggested that UV radiation can affect both photosynthetic performance of G. lingulatum and associated microbial communities. UV exposure caused photoinhibition, but effective recovery is reached in all studied seaweed populations. There were no differences in UV response and total abundances of associated bacteria between different populations of G. lingulatum. Changes in microbial communities associated with G. lingulatum due to UV exposure were less prominent between long-acclimated and field-collected populations. Microbes associated with G. lingulatum may play an important ecological and functional role in seaweed–microbial interactions, which should be investigated in the future. Differences in microbial communities between field and acclimated G. lingulatum also highlight the importance of studying spatial and temporal variability of seaweed holobiomes in natural habitats.
Author contributions
S. Dobrestov: experimental design, data analysis, drafting and editing the manuscript; K. Véliz: experimental design and lab work, data analysis, drafting and editing the manuscript; M. Soledad Romero: data analysis and editing the manuscript; F. Tala: drafting and editing the manuscript; M. Thiel: experimental design, and editing the manuscript.
TEJP-2019-0111-File004.docx
Download MS Word (257.3 KB)Acknowledgements
We are grateful to David Yañez for his collaboration in laboratory activities and access to the facilities of the Botany Lab. We acknowledge David Jofré Madariaga, Oscar Pino and Felipe Sáez for their help in the collection of field samples.
Disclosure statement
No potential conflict of interest was reported by the authors.
Supplementary materials
The following supplementary material is accessible via the Supplementary Content tab on the article’s online page at: https://doi.org/10.1080/09670262.2020.1775309
Supplemental figure 1. Daily variation in maximal quantum yield (Fv/Fm) among populations of Gelidium lingulatum maintained under control condition (only PAR). Initial values of Fv/Fm where measured at Day 0. Values are mean (n = 3) ± SD. Different letters above the bars indicated significant differences according to the Tukey post hoc test at p < 0.05.
Additional information
Funding
References
- Agogué, H., Joux, F., Obernosterer, I. & Lebaron, P. (2005). Resistance of marine bacterioneuston to solar radiation. Applied and Environmental Microbiology, 71: 5282–5289.
- Aires, T., Moalic, Y., Serrao, E.A. & Arnaud-Haond, S. (2015). Hologenome theory supported by cooccurrence networks of species-specific bacterial communities in siphonous algae (Caulerpa). FEMS Microbiology Ecology, 91: fiv067.
- Bischof, K., Hanelt, D. & Wiencke, C. (2000). Effects of ultraviolet radiation on photosynthesis and related enzyme reactions of marine macroalgae. Planta, 211: 555–562.
- Brodie, J., Williamson, C., Barker, G.L., Walker, R.H., Briscoe, A. & Yallop, M. (2016). Characterising the microbiome of Corallina officinalis, a dominant calcified intertidal red alga. FEMS Microbiology Ecology, 92: fiw110.
- Burke, C., Thomas, T., Lewis, M., Steinberg, P. & Kjelleberg, S. (2011). Composition, uniqueness and variability of the epiphytic bacterial community of the green alga Ulva australis. ISME Journal, 5: 590–600.
- Buschmann, A.H., Correa, J.A., Westermeier, R., Hernández-González, M. del C. & Norambuena, R. (2001). Red algal farming in Chile: a review. Aquaculture, 194: 203–220.
- Campbell, A.H., Marzinelli, E.M., Gelber, J. & Steinberg, P.D. (2015). Spatial variability of microbial assemblages associated with a dominant habitat-forming seaweed. Frontiers in Microbiology, 6: 230.
- Chen, M.Y. & Parfrey, L.W. (2018). Incubation with macroalgae induces large shifts in water column microbiota, but minor changes to the epibiota of co-occurring macroalgae. Molecular Ecology, 27: 1966–1979.
- Clarke, A. (2003). Costs and consequences of evolutionary temperature adaptation. Trends in Ecology and Evolution, 18: 573–581.
- de Mesquita, M.M.F., Crapez, M.A.C., Teixeira, V.L. & Cavalcanti, D.N. (2019). Potential interactions bacteria-brown algae. Journal of Applied Phycology, 31: 867–883.
- de Oliveira, L.S., Gregoracci, G.B., Silva, G.G.Z., Salgado, L.T., Filho, G.A., Alves-Ferreira, M., Pereira, R.C. & Thompson, F.L. (2012). Transcriptomic analysis of the red seaweed Laurencia dendroidea (Florideophyceae, Rhodophyta) and its microbiome. BMC Genomics, 13: 487.
- Dobretsov, S., Dahms, H.-U., Harder, T. & Qian, P.-Y. (2006). Allelochemical defense against epibiosis in the macroalga Caulerpa racemosa var. turbinata. Marine Ecology Progress Series, 318: 165–175.
- Dobretsov, S.V. & Qian, P.-Y. (2002). Effect of bacteria associated with the green alga Ulva reticulata on marine micro- and macrofouling. Biofouling 18: 217–228.
- Dowd, S.E., Wolcott, R.D., Sun, Y., McKeehan, T., Smith, E. & Rhoads, D. (2008). Polymicrobial nature of chronic diabetic foot ulcer biofilm infections determined using bacterial tag encoded FLX amplicon pyrosequencing (bTEFAP). PLoS ONE, 3: e3326.
- Egan, S., Harder, T., Burke, C., Steinberg, P., Kjelleberg, S. & Thomas, T. (2013). The seaweed holobiont: understanding seaweed–bacteria interactions. FEMS Microbiology Review, 37: 462–476.
- Egan, S., Thomas, T. & Kjelleberg, S. (2008). Unlocking the diversity and biotechnological potential of marine surface associated microbial communities. Current Opinion of Microbiology, 11: 219–225.
- Enríquez, S. & Borowitzka, M. (2010). The use of the fluorescence signal in studies of sea grasses and macroalgae. In Chlorophyll a Fluorescence in Aquatic Sciences: Methods and Applications ( Suggett, D.J., Borowitzka, M.A. & Prášil, O., editors), 187–208. Springer, Dordrecht.
- Falkowski, P.G. & LaRoche, J. (1991). Acclimation to spectral irradiance in algae. Journal of Phycology, 27: 8–14.
- Florez, J.Z., Camus, C., Hengst, M.B. & Buschmann, A.H. (2017). A functional perspective analysis of macroalgae and epiphytic bacterial community interaction. Frontiers in Microbiology, 8: 2561.
- Florez, J.Z., Camus, C., Hengst, M.B., Marchant, F. & Buschmann, A.H. (2019). Structure of the epiphytic bacterial communities of Macrocystis pyrifera in localities with contrasting nitrogen concentrations and temperature. Algal Research, 44: 101706.
- Goecke, F., Labes, A., Wiese, J. & Imhoff, J.F. (2013). Phylogenetic analysis and antibiotic activity of bacteria isolated from the surface of two co-occurring macroalgae from the Baltic Sea. European Journal of Phycology, 48: 47–60.
- Goosen, N. & Moolenaar, G.F. (2008). Repair of UV damage in bacteria. DNA Repair, 7: 353–379.
- Harder, T. (2009). Marine epibiosis: concepts, ecological consequences and host defence. In Marine and Industrial Biofouling (Flemming, H.-C., Murthy, P.S., Venkatesan, R. & Cooksey, K., editors), 219–231. Springer, Dordrecht.
- Hengst, M.B., Andrade, S., González, B. & Correa, J.A. (2010). Changes in epiphytic bacterial communities of intertidal seaweeds modulated by host, temporality, and copper enrichment. Microbial Ecology, 60: 282–290.
- Hepburn, C.D. & Hurd, C.L. (2005). Conditional mutualism between the giant kelp Macrocystis pyrifera and colonial epifauna. Marine Ecology Progress Series, 302: 37–48.
- Hernández, K., Yannicelli, B., Montecinos, A., Ramos, M., González, H.E. & Daneri, G. (2012). Temporal variability of incidental solar radiation and modulating factors in a coastal upwelling area (36°S). Progress in Oceanography, 92–95: 18–32.
- Hollants, J., Leliaert, F., De Clerck, O. & Willems, A. (2013). What we can learn from sushi: a review on seaweed-bacterial associations. FEMS Microbial Ecology, 83: 1–16.
- Hudson, J., Kumar, V. & Egan, S. (2019). Comparative genome analysis provides novel insight into the interaction of Aquimarina sp. AD1, BL5 and AD10 with their macroalgal host. Marine Genomics, 46: 8–15.
- Hünken, M., Harder, J. & Kirst, G.O. (2008). Epiphytic bacteria on the Antarctic ice diatom Amphiprora kufferathii Manguin cleave hydrogen peroxide produced during algal photosynthesis. Plant Biology, 10: 519–526.
- Huovinen, P., Gómez, I., Figueroa, F.L., Ulloa, N., Morales, V. & Lovengreen, C. (2005). Ultraviolet-absorbing mycosporine-like amino acids in red macroalgae from Chile. Botanica Marina, 47: 21–29.
- Hurd, C.L., Harrison, P.J., Bischof, K. & Lobban, C.S. (2014). Seaweed Ecology and Physiology. 2nd ed. Cambridge University Press, Cambridge.
- Jung, Y.T., Lee, J.S. & Yoon, J.H. (2016). Lewinella aquimaris sp. nov., isolated from seawater. International Journal of Systematic and Evolutionary Microbiology, 16: 3989–3994. doi:10.1099/ijsem.0.001299
- Kang, H., Kim, H., Joung, Y. & Joh, K. (2017). Lewinella maritima sp. nov., and Lewinella lacunae sp. nov., novel bacteria from marine environments. International Journal of Systematic and Evolutionary Microbiology, 67: 3603–3609. doi:10.1099/ijsem.0.002176
- Lachnit, T., Meske, D., Wahl, M., Harder, T. & Schmitz, R. (2011). Epibacterial community patterns on marine macroalgae are host-specific but temporally variable. Environmental Microbiology, 13: 655–665.
- Lemay, M.A., Martone, P.T., Hind, K.R., Lindstrom, S.C. & Parfrey, L.W. (2018). Alternate life history phases of a common seaweed have distinct microbial surface communities. Molecular Ecology, 27: 3555–3568.
- Longford, S.R., Campbell, A.H., Nielsen, S., Case, R.J., Kjelleberg, S. & Steinberg, P.D. (2019). Interactions within the microbiome alter microbial interactions with host chemical defences and affect disease in a marine holobiont. Scientific Reports, 9: 1363.
- Longford, S.R., Tujula, N.A., Crocetti, G.R., Holmes, A.J., Holmström, C., Kjelleberg, S., Steinberg, P.D. & Taylor, M.W. (2007). Comparisons of diversity of bacterial communities associated with three sessile marine eukaryotes. Aquatic Microbial Ecology, 48: 217–229.
- Loos, van der, L.M., Eriksson, B.K. & Falcão Salles, J. (2019). The macroalgal holobiont in a changing sea. Trends in Microbiology, 27: 635–650.
- López, B.A., Tellier, F., Retamal-Alarcón, J.C., Pérez-Araneda, K., Fierro, A.O., Macaya, E.C., Tala, F. & Thiel, M. (2017). Phylogeography of two intertidal seaweeds, Gelidium lingulatum and G. rex (Rhodophyta: Gelidiales), along the South East Pacific: patterns explained by rafting dispersal? Marine Biology, 164: 188.
- Macaya, E.C., López, B., Tala, F., Tellier, F. & Thiel, M. (2016). Float and raft: role of buoyant seaweeds in the phylogeography and genetic structure of non-buoyant associated flora. In Seaweed Phylogeography: Adaptation and Evolution of Seaweeds under Environmental Change (Hu, Z.-M. & Fraser, C., editors), 97–130. Springer, Dordrecht.
- Mancuso, F.P., D’Hondt, S., Willems, A., Airoldi, L. & De Clerck, O. (2016). Diversity and temporal dynamics of the epiphytic bacterial communities associated with the canopy-forming seaweed Cystoseira compressa (Esper) Gerloff and Nizamuddin. Frontiers in Microbiology, 7: 476.
- Manrique, J.M., Calvo, A.Y., Halac, S.R., Villafañe, V.E., Jones, L.R. & Helbling, H.E. (2012). Effects of UV radiation on the taxonomic composition of natural bacterioplankton communities from Bahía Engaño (Patagonia, Argentina). Journal of Photochemistry and Photobiology B, 117: 171–178.
- Martin, M., Barbeyron, T., Martin, R., Portetelle, D., Michel, G. & Vandenbol, M. (2015). The cultivable surface microbiota of the brown alga Ascophyllum nodosum is enriched in macroalgal-polysaccharide-degrading bacteria. Frontiers in Microbiology, 6: 1487.
- Matsuo, Y., Imagawa, H., Nishizawa, M. & Shizuri, Y. (2005). Isolation of an algal morphogenesis inducer from a marine bacterium. Science, 307: 1598.
- Michelou, V.K., Caporaso, J.G., Knight, R. & Palumbi, S.R. (2013). The ecology of microbial communities associated with Macrocystis pyrifera. PLoS ONE, 8: e67480.
- Miranda, L.N., Hutchison, K., Grossman, A.R. & Brawley, S.H. (2013). Diversity and abundance of the bacterial community of the red macroalga Porphyra umbilicalis: did bacterial farmers produce macroalgae? PLoS ONE, 8: e58269.
- Montalva, S. & Santelices, B. (1981). Interspecific interference among species of Gelidium from Central Chile. Journal of Experimental Marine Biology and Ecology, 53: 77–88.
- Paix, B., Carriot, N., Barry-Martinet, R., Greff, S., Misson, B., Briand, J.-F. & Culioli, G. (2020). A multi-omics analysis suggests links between the differentiated surface metabolome and epiphytic microbiota along the thallus of a Mediterranean seaweed holobiont. Frontiers in Microbiology, 11: 494.
- Paix, B., Othmani, A., Debroas, D., Culioli, G. & Briand, J.-F. (2019). Temporal covariation of epibacterial community and surface metabolome in the Mediterranean seaweed holobiont Taonia atomaria. Environmental Microbiology. https://doi.org/10.1111/1462-2920.14617.
- Paul, N.A., Nys, R. de & Steinberg, P.D. (2006). Chemical defence against bacteria in the red alga Asparagopsis armata: linking structure with function. Marine Ecology Progress Series, 306: 87–101.
- Raddatz, S., Guy‐Haim, T., Rilov, G. & Wahl, M. (2017). Future warming and acidification effects on anti-fouling and anti-herbivory traits of the brown alga Fucus vesiculosus (Phaeophyceae). Journal of Phycology, 53: 44–58.
- Ramírez, M. & Santelices, B. (1991). Catálogo de las algas marinas bentónicas de la costa temperada del Pacífico de Sudamérica. Ediciones Universidad Católica de Chile, Santiago de Chile.
- Ramos-Rodríguez, A., Lluch-Cota, D.B., Lluch-Cota, S.E. & Trasviña-Castro, A. (2012). Sea surface temperature anomalies, seasonal cycle and trend regimes in the Eastern Pacific coast. Ocean Science, 8: 81–90.
- Saha, M., Ferguson, R.M.W., Dove, S., Künzel, S., Meichssner, R., Neulinger, S.C., Petersen, F.O. & Weinberger F. (2020). Salinity and time can alter epibacterial communities of an invasive seaweed. Frontiers in Microbiology, 10: 2870. doi: 10.3389/fmicb.2019.02870.
- Saha, M. & Weinberger, F. (2019). Microbial “gardening” by a seaweed holobiont: surface metabolites attract protective and deter pathogenic epibacterial settlement. Journal of Ecology. https://doi.org/10.1111/1365-2745.13193.
- Santelices, B. & Varela, D. (1994). Abiotic control of reattachment in Gelidium chilense (Montagne) Santelices & Montalva (Gelidiales; Rhodophyta). Journal of Experimental Marine Biology and Ecology, 177: 145–155.
- Santos, A.L., Lopes, S., Baptista, I., Henriques, I., Gomes, N.C.M., Almeida, A., Correia, A. & Cunha, A. (2011). Diversity in UV sensitivity and recovery potential among bacterioneuston and bacterioplankton isolates. Letters in Applied Microbiology, 52: 360–366.
- Santos, A.L., Oliveira, V., Baptista, I., Henriques, I., Gomes, N.C., Almeida, A., Correia, A. & Cunha, A. (2012). Effects of UV-B radiation on the structural and physiological diversity of bacterioneuston and bacterioplankton. Applied and Environmental Microbiology, 78: 2066–2069.
- Selvarajan, R., Sibanda, T., Venkatachalam, S., Ogola, H.J.O., Obieze, C.C. & Msagati, T.A. (2019). Distribution, interaction and functional profiles of epiphytic bacterial communities from the rocky intertidal seaweeds, South Africa. Scientific Reports, 9: 19835.
- Singh, R.P., Mantri, V.A., Reddy, C.R.K. & Jha, B. (2011). Isolation of seaweed-associated bacteria and their morphogenesis-inducing capability in axenic cultures of the green alga Ulva fasciata. Aquatic Biology, 12: 13–21.
- Singh, R.P. & Reddy, C.R.K. (2014). Seaweed–microbial interactions: key functions of seaweed-associated bacteria. FEMS Microbiology Ecology, 88: 213–230.
- Staufenberger, T., Thiel, V., Wiese, J. & Imhoff, J.F. (2008). Phylogenetic analysis of bacteria associated with Laminaria saccharina. FEMS Microbiology Ecology, 64: 65–77.
- Stratil, S.B., Neulinger, S.C., Knecht, H., Friedrichs, A.K. & Wahl, M. (2013). Temperature-driven shifts in the epibiotic bacterial community composition of the brown macroalga Fucus vesiculosus. Microbiology Open, 2: 338–349.
- Tapia, F.J., Largier, J.L., Castillo, M., Wieters, E.A. & Navarrete, S.A. (2014). Latitudinal discontinuity in thermal conditions along the nearshore of Central-Northern Chile. PLoS ONE, 9: e110841.
- Tujula, N.A., Crocetti, G.R., Burke, C., Thomas, T., Holmström, C. & Kjelleberg, S. (2010). Variability and abundance of the epiphytic bacterial community associated with a green marine Ulvacean alga. ISME Journal, 4: 301–311.
- Véliz, K., Chandía, N., Bischof, K. & Thiel, M. (2020). Geographic variation of UV stress tolerance in red seaweeds does not scale with latitude along the SE Pacific coast. Journal of Phycology. https://doi.org/10.1111/jpy.13009.
- Véliz, K., Chandía, N., Karsten, U., Lara, C. & Thiel, M. (2019). Geographic variation in biochemical and physiological traits of the red seaweeds Chondracanthus chamissoi and Gelidium lingulatum from the south east Pacific coast. Journal of Applied Phycology, 31: 665–682.
- Véliz, K., Edding, M., Tala, F. & Gómez, I. (2006). Effects of ultraviolet radiation on different life cycle stages of the south Pacific kelps, Lessonia nigrescens and Lessonia trabeculata (Laminariales, Phaeophyceae). Marine Biology, 149: 1015–1024.
- Vernet, M., Diaz, S.B., Fuenzalida, H.A., Camilion, C., Booth, C.R., Cabrera, S., Casiccia, C., Deferrari, G., Lovengreen, C., Paladini, A., Pedroni, J., Rosales, A. & Zagarese, H.E. (2009). Quality of UVR exposure for different biological systems along a latitudinal gradient. Photochememical & Photobiological Sciences, 8: 1329–1345.
- Wahl, M. (1989). Marine epibiosis. I. Fouling and antifouling: some basic aspects. Marine Ecology Progress Series, 58: 175–189.
- Wahl, M., Goecke, F., Labes, A., Dobretsov, S. & Weinberger, F. (2012). The second skin: ecological role of epibiotic biofilms on marine organisms. Frontiers in Microbiology, 3: 292.
- Wahl, M., Shahnaz, L., Dobretsov, S., Saha, M., Symanowski, F., David, K., Lachnit, T., Vasel, M. & Weinberger, F. (2010). Ecology of antifouling resistance in the bladder wrack Fucus vesiculosus: patterns of microfouling and antimicrobial protection. Marine Ecology Progress Series, 411: 33–48.
- Weigel, B.L. & Pfister, C.A. (2019). Successional dynamics and seascape-level patterns of microbial communities on the canopy-forming kelps Nereocystis luetkeana and Macrocystis pyrifera. Frontiers in Microbiology, 10: 346.
- Wichard, T. (2015). Exploring bacteria-induced growth and morphogenesis in the green macroalga order Ulvales (Chlorophyta). Frontiers in Plant Science, 6: 86.
- Wiencke, C., Lüder, U.H. & Roleda, M.Y. (2007). Impact of ultraviolet radiation on physiology and development of zoospores of the brown alga Alaria esculenta from Spitsbergen. Physiologia Plantarum, 130: 601–612.
- Wiese, J., Thiel, V., Nagel, K., Staufenberger, T. & Imhoff, J.F. (2009). Diversity of antibiotic active bacteria associated with the brown alga Laminaria saccharina from the Baltic Sea. Marine Biotechnology, 11: 287–300.
- Wieters, E.A., Medrano, A. & Quiroga, G. (2013). Spatial variation in photosynthetic recovery of intertidal turf algae from acute UVB and temperature stress associated with low tides along the central coast of Chile. Journal of Experimental Marine Biology and Ecology, 449: 340–348.
- Yokoya, N.S. & Handro, W. (2002). Effects of plant growth regulators and culture medium on morphogenesis of Solieria filiformis (Rhodophyta) cultured in vitro. Journal of Applied Phycology, 14: 97–102.
- Zar, J.H. (2010). Biostatistical Analysis. 5th ed. Prentice Hall, Pearson.
- Zhang, Z., Li, S., Li, J., Gu, X. & Lin, X. (2019). Complete genome sequences of a H2O2-resistant psychrophilic bacterium Colwellia sp. Arc7-D isolated from Arctic Ocean sediment. Marine Genomics, 43: 65–67.
- Zhao, Z., Pan, Y., Jiang, J., Gao, S., Sun, H., Dong, Y., Sun, P., Guan, X. & Zhou, Z. (2018). Unrevealing variation of microbial communities and correlation with environmental variables in a full culture-cycle of Undaria pinnatifida. Marine Environmental Research, 139: 46–56.