Abstract
Osteoarthritis (OA) is a progressive chronic inflammation that leads to cartilage degeneration. OA Patients are commonly given pharmacological treatment, but the available treatments are not sufficiently effective. The development of sustained-release drug delivery systems (DDSs) for OA may be an attractive strategy to prevent rapid drug clearance and improve the half-life of a drug at the joint cavity. Such delivery systems will improve the therapeutic effects of anti-inflammatory effects in the joint cavity. Whereas, for disease-modifying OA drugs (DMOADs) which target chondrocytes or act on mesenchymal stem cells (MSCs), the cartilage-permeable DDSs are required to maximize their efficacy. This review provides an overview of joint structure in healthy and pathological conditions, introduces the advances of the sustained-release DDSs and the permeable DDSs, and discusses the rational design of the permeable DDSs for OA treatment. We hope that the ideas generated in this review will promote the development of effective OA drugs in the future.
1. Introduction
Osteoarthritis (OA) is the most prevalent progressive chronic disease globally. It is characterized by an inflammatory environment and articular cartilage damage, leading to more severe synovial edema, osteophytes, and subchondral bone sclerosis (Zhong et al., Citation2019; Zeng et al., Citation2021). Further, the cause of OA is still unclear. It is not caused by a single factor but a result of the combination of multiple factors, among which old age and overweight are the main pathogenic factors (Krishnamurthy et al., Citation2021). The work capacity of OA patients is greatly impaired, which affects their quality of life and causes an economic burden on individuals and society (Losina et al., Citation2015).
According to the International Cartilage Repair Society (ICRS) Cartilage Lesion Classification System, OA is divided into four levels as follows. ICRS grade I: cartilage has superficial, blunt gaps, and superficial cracks; ICRS grade II: cartilage damage depth <50% of cartilage depth; ICRS grade III: cartilage damage depth >50% of cartilage depth, but not reaching the subchondral bone; ICRS grade IV: total cartilage tear with exposure of subchondral bone (van der Meijden et al., Citation2012). It has been noted that OA can be treated with drugs when the degree of cartilage damage is ICRS grade I-III. However, surgical treatment is needed when the disease progresses to ICRS grade IV.
Currently, the common drugs used for treating OA include selective COX-2 enzyme inhibitors, non-steroidal anti-inflammatory drugs (NSAIDs), glucocorticoids, viscosupplements, and other traditional drugs. However, injecting the drugs directly into the joint cavity can only relieve inflammatory symptoms and delay the progress of the disease but cannot reverse the course of the disease and cure OA. Furthermore, the drugs also have the disadvantages of being easily eliminated and the risk of causing adverse reactions (Lin et al., Citation2004). To increase the retention capacity of the drugs and reduce adverse reactions, many researchers are committed to the development of formulations or drug delivery systems (DDS) with a slow-release effect (Zhang et al., Citation2018; Kou et al., Citation2019; Xue et al., Citation2021). Micro/nano DDS is the most common DDS for intra-articular injection because of its good safety, sustained-release function, and easy modification feature. There are three kinds of micro/nano-drug carriers: suspension, binding, and permeation () (Bajpayee & Grodzinsky, Citation2017).
Figure 1. Three types of micro/nano drug carriers: suspension drug carriers, binding drug carriers, permeable drug carriers, and their characteristics.
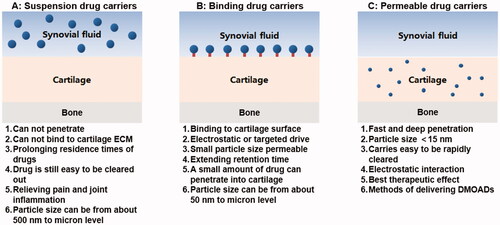
Suspension type and binding type drug carriers can solve the problem of rapid clearance and short half-life of drugs in the joint cavity. They also greatly improve the therapeutic effect of the drugs that work in the joint cavity. However, the extracellular matrix (ECM) is lost only at ICRS grade III-IV. And at that time, the suspension and binding drug carriers can give full play to the efficacy of the drug that promotes proliferation and differentiation of mesenchymal stem cells (MSCs) or acts on chondrocytes, such as Kartogenin (KGN) (Zeng et al., Citation2021). When OA is at ICRS grade I-II and the ECM is relatively intact, very few parts of the drugs released from suspension or binding drug carriers can permeate into the cartilage, and the penetration depth is insufficient. Therefore, the drug cannot reach the therapeutic concentration at the target site, thereby compromising therapeutic efficacy.
It has been found that it is more likely to interrupt or even reverse the OA process for earlier treatment. This is because OA is a chronic progressive disease followed by increasing inflammation and the continuous excessive catabolism of cartilage (Krych et al., Citation2020). The intervention and treatment are critical for OA patients at ICRS grade I-II. Moreover, with the continuous exploration of OA disease-modifying drugs (DMOADs), the possibility of reversing the course and curing OA is also increasing. Therefore, it is necessary for the investigation and development of DDS with penetration potential that can deliver the drugs to the target cell in deep ECM for maximum efficacy. The present review introduced joint structure in healthy and pathological conditions, summarized the advances of the sustained DDSs and permeable DDSs for OA treatment in recent years, and discussed the advantages and innovations of these DDS. We hope it can provide new ideas for researchers in related areas.
2. Joint structure in healthy and pathological conditions
Understanding the knee joint structure in healthy conditions and its pathological changes in patients with OA plays an important role in understanding the DDS in the articular cavity. The basic composition of the knee joint includes articular cartilage, capsule, cavity, and subchondral bone. The present study introduced each part of the knee joint for a deep understanding of the microenvironment of the OA joint ().
Figure 2. Structural changes of joints before and after OA and schematic diagram of aggrecan. Structural comparison of healthy joints and osteoarthritis (OA) joints. OA involves synovitis, cartilage degeneration, osteophyte formation and joint pain. Small molecular GAG is like a mane on a test tube brush. It binds to the core protein by a covalent bond and radiates outward with the core protein in the center, and then binds to the hyaluronic acid trunk to form an aggrecan. (Created with BioRender.com).
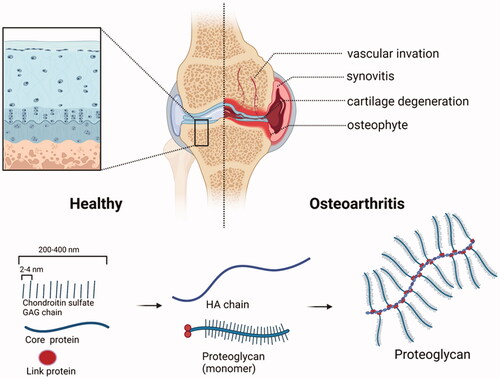
2.1. Articular cartilage
Articular cartilage is a thin layer of hyaline cartilage covering the articular surface at the end of the bone. It has a certain elasticity and smooth surface, which is enhanced for joint movement. Articular cartilage can be divided into chondrocytes and ECM. Chondrocytes play an important role in the stability of the knee joint. They secrete catabolic and anabolic enzymes to maintain the catabolism and anabolism balance of the cartilage tissue. The ECM is composed of matrix and collagen fibers. Type II collagen (Coll-II) accounts for about 90% of the collagen content in the extracellular matrix of cartilage, whereas proteoglycan is the main component of the matrix, containing at least one glycosaminoglycan (GAG) side chain.
Glycosaminoglycan (GAG) includes chondroitin sulfate (CHS), dermatan sulfate (DS), heparan sulfate (HS), keratin sulfate (KS), and hyaluronic acid (HA). It confers the overall negative charge and dense microporous structure (). Proteoglycan is important for the metabolism of chondrocytes (Bottini et al., Citation2016). When OA occurs, GAG is stimulated by pro-inflammatory factors such as interleukin-1β (IL-1-β), tumor necrosis factor-α (TNF-α), interleukin-6 (IL-6), interleukin-15 (IL-15), interleukin-17 (IL-17), and interleukin-18 (IL-18) (Mathiessen & Conaghan, Citation2017).
Expression levels of type II collagen, proteoglycan genes, and related proteins will significantly decrease. In contrast, there will be a significant increase in MMP and proteoglycan enzyme expression levels so that the original balance between synthetic metabolic enzymes and catabolic enzymes in ECM is destroyed. Therefore, this causes degradation and loss of ECM, leading to the death of chondrocytes, further aggravating inflammation as well as accelerating the progress of OA (Pelletier et al., Citation1993).
2.2. Subchondral bone
The epiphysis of long bones is beneath the calcified layer of articular cartilage. The epiphysis can be divided into a dense cortical bone plate on the surface and a looser, more metabolically active cancellous bone distally. The structure mentioned above is called the subchondral bone, which, together with the articular cartilage, serves as the load cell of the knee joint. On the other hand, the subchondral bone mass and density caused by bone remodeling will cause uneven stress on the articular cartilage, resulting in a shear force, causing damage to the articular cartilage. Burr, D.B. et al. reviewed the vital role of bone remodeling in the occurrence and development of OA (Burr & Gallant, Citation2012). In early osteoarthritis, the cortical bone plate of the subchondral bone becomes thinner and the cancellous bone is lost as the rate of bone remodeling increases. When osteoarthritis progresses to an advanced stage, the balance of bone remodeling is tilted toward the bone formation, resulting in subchondral sclerosis, but the newly formed bone remains less rigid due to incomplete mineralization. In addition to force conduction, there are many molecular interactions between subchondral bone and cartilage in the development of osteoarthritis (Lories & Luyten, Citation2011). In the disease state, the layer of calcified cartilage is destroyed, and there are many tiny fissures in the articular cartilage that extend into the subchondral bone. Subchondral bone has blood vessels that reach the deep cartilage. Cartilage decomposing substances such as MMP-13 secreted by subchondral osteoblasts follow the channels such as microcracks and blood vessels between bone and cartilage, further causing cartilage loss.
2.3. Articular capsule
The articular capsule is a cystic structure composed of fibrous connective tissue that closes the articular cavity. It is divided into inner and outer layers, whereby the outer layer is collagen fibril dense connective tissue, which is continuous with the periosteum for maintenance of the joint stability. On the other hand, the inner layer, which is also called synovium, is loose and has a smooth surface. It protrudes to the joint cavity to form synovial folds or villi and has lymphatic vessels inside it. There are two types of synovial cells covering the synovial. One is the macrophage-like cell, which contains more lysosomes and has phagocytosis ability and the other is the fibroblast, which contains rougher endoplasmic reticulum and secretes hyaluronic acid as well as mucopolysaccharide, which lubricates the joints.
When Osteoarthritis disease occurs, cartilage releases the products of proteoglycan degradation and damage-associated molecular patterns (DAMPs) into the joint space. These molecules activate macrophages in the synovium and secrete pro-inflammatory cytokines (Brown et al., Citation2019). Subsequently, the function of synovial cells is affected. Further, the secretion of hyaluronic acid and mucopolysaccharide is significantly reduced, the network structure of the synovium is destroyed, and pathological changes such as hyperemia, edema, and hyperplasia occur (Zheng et al., Citation2021).
2.4. Articular cavity
The articular cavity is a closed space enclosed by the synovial layer of the articular capsule and articular cartilage, which contains a small amount of transparent viscous fluid called synovial fluid. The main ingredient of synovial fluid in the articular cavity is including water, hyaluronic acid, mucopolysaccharide, and electrolytes. When OA occurs, the synovial fluid is filled with pro-inflammatory factors (Mathiessen & Conaghan, Citation2017). The infiltration of inflammatory factors promotes catabolism of chondrocytes and degradation of cartilage. Deterioration of OA affects the entire joint, including subchondral bone, ligaments, synovium, and peri-articular muscles. Further, more severe symptoms appear around the articular cavity: fibrocartilage degeneration, chondro-osteophytes, protrusions, subsynovial inflammatory, and joint effusion (Pereira et al., Citation2015). Due to the damage of the joint function, it may also cause the atrophy of thigh and calf muscles, whereby the first atrophied part is the skeletal muscle in the inner thigh. Subsequently, it causes an imbalance of force on the limbs, increases the burden on the lumbar spine, and causes some diseases related to the spine.
3. Drug delivery system (DDS) for OA therapy
The knee joint is a closed structure, and the articular cartilage lacks blood vessels, making it difficult for drugs to accumulate in the joint through the bloodstream. This can lead to limited efficacy and systemic side effects. On the other hand, IA injection delivery has fewer systemic side effects, rapid therapeutic concentration, and fast pain relief, among other advantages. Therefore, IA injection has become a widely used method of drug delivery in clinic practice. In this section, the ordinary injection, sustained-release DDSs, and permeable DDSs used for intra-articular injection are introduced.
3.1. Ordinary injection
Currently, the main drugs commonly used for IA injection in the knee joint are anti-inflammatory drugs (represented by corticosteroids) and intrinsic components of the articular cartilage matrix represented by HA and aminoglycan. This is according to the 2019 American College of Rheumatology Arthritis Foundation guidelines for the treatment of OA (Kolasinski et al., Citation2020).
However, there is still a lack of sufficient credible data to demonstrate the effectiveness of the above-mentioned therapies. According to Maheu et al., there is only low-grade evidence that corticosteroids have a greater benefit on pain and function than controls, thus are likely to be analgesic only in a short term (<4 weeks) (Maheu et al., Citation2019), whereas HA is ineffective in severe OA (Zhang et al., Citation2018). Further, the latest Bayesian network meta-analysis results of a 3-, 6-, and 12-month follow-up, showed that there is no significant difference between Corticosteroids, HA, and placebo in the metrics of ability to minimize pain (VAS score) and greatest overall outcome (WOMAC) (Migliorini et al., Citation2021).
Besides their uncertain efficacy are also non-negligible adverse effects: including accelerated osteoarticular progression, subchondral bone fractures, and bone loss. In a follow-up of 152 patients with IA knee injections of corticosteroid, OA rapidly worsened in 6% of patients (Kompel et al., Citation2019). The role of glucocorticoid injections in reducing the rate of cartilage loss has also been questioned. A recent clinical study revealed more significant cartilage loss in the triamcinolone acetonide-injected group compared with the saline-injected group (–0.21 vs −0.10 mm; between-group difference, −0.11 mm; 95% CI, −0.20 to −0.03 mm) (McAlindon et al., Citation2017). Glucocorticoids may cause cartilage damage by inhibiting chondrocyte proliferation, promoting chondrocyte apoptosis (Nakazawa et al., Citation2002), and affecting cartilage anabolism (Behrens et al., Citation1975). Due to repeated IA injections, HA treatment with fewer side effects may also result in transient hyperglycemia, skin redness, and fever as well as popular-like changes, local infections, skin atrophy, and periarticular calcification (McGarry & Daruwalla, Citation2011). Therefore, improved systems of drug delivery may be the key to addressing the limitations of the common injectable dosage forms that require repeated dosage, lack of long-term effects, and severe side effects. Many researchers are thus focusing on developing extended-release drug delivery systems for OA.
3.2. Sustained-release DDS
IA injection is the most suitable mode of administration for the treatment of osteoarthritis since the osteoarthritic condition is limited to the joint site. However, small-molecule drugs are easily removed by blood vessels and lymphatic vessels after entering the joint cavity (). Excessive clearance rate causes failure of the drug in the joint cavity to reach a therapeutic dose. This challenge can only be solved by frequent and multiple administrations, which may cause inflammation and worsen the condition. Therefore, sustained-release DDSs are needed to extend the residence time and increase the solubility of these drugs in the joint cavity. Although some achievements have been made, this is still a hot realm for joint DDS. It has been found that autologous liquid platelet-rich fibrin is a novel DDS that can release large biomolecules such as growth factors and antigenic factors, among others and small biomolecules such as antibodies, polypeptides, and gene therapy drugs among others) continually and controllable. Furthermore, it can reduce the clearance rate of biomolecules, prevent the immune response as well as effectively promote the repair of cartilage damage (Fu et al., Citation2012). Therefore, the present study introduced some sustained-releases DDSs based on different biomaterials as summarized in .
Figure 3. Schematic diagram of DDSs retention and clearance characteristics of different intra-articulate osteoarthritis (OA) drug delivery systems. Free drugs, hydrogels, nanoparticles, and microparticles could retain in the joint cavity for hours, weeks, and months, respectively. Macromolecules (>10 kDa) were eliminated through the lymphatics, while small molecules (<10 kDa) were eliminated through blood vessels. (Created with BioRender.com).
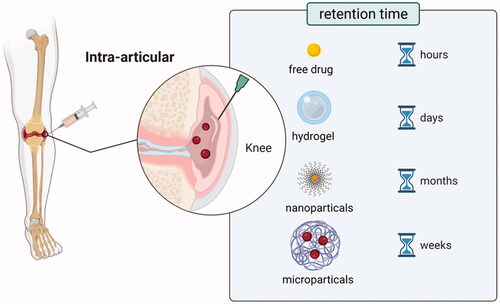
Table 1. Drug delivery systems with sustained release profile for OA therapy.
3.2.1. Chitosan (CS)
Chitosan (CS) is the product of natural polysaccharide chitin which removes part of the acetyl group. It has good biodegradation, biocompatibility, nontoxicity, and other biological characteristics. It is widely used in many fields, such as medical fiber, medical dressing, drug slow-release material, etc (Muxika et al., Citation2017). Chitosan nanoparticles (CNs) have been established as a useful drug delivery tool because they are easy to prepare, can load different macromolecules and drugs, and can act on different tissues or organs in different ways.
As drug carriers, CNs not only prolong the drug retention time but also relieve OA (Zhou et al., Citation2015). Therefore, CNs are very attractive biopolymers for sustained-releases DDS to OA. Kang et al. designed a dual DDS which encapsulated Kartogenin (KGN) and diclofenac (DCF) based on CN conjugated pluronic F127 grafting carboxyl group to treat OA (Kang et al., Citation2016). DCF has anti-inflammatory and analgesic effects. And KGN is an inducer for the differentiation of human mesenchymal stem cells into chondrocytes. It can bind to fibrinogen A, destroys its interaction with transcription factor core binding factor β subunit (CBFβ), and induces cartilage formation by regulating the CBFβ-RUNX1 transcription procedure (Cai et al., Citation2019). The designed dual DDS could provide synchronous and independent dual drug delivery in response to temperature changes. In addition, cold stimulation played an important role in drug release and efficacy. It loosened the structure of nanoparticles, which made it easier for drugs to show different release forms due to enhanced hydrolysis. On the other hand, cold stimulation and nanoparticles have a synergistic effect on the treatment of OA.
Furthermore, the combination of CS and HA can also help the DDS stay in the articular cavity and improve the ability of sustained drug release. In a previous study, Curcumin-loaded HA/CS nanoparticles were found to have a good sustained-release property. With the help of chitosan nanoparticles, a stable fusion of curcumin and HA was achieved, which synergistically acted on the NF-κB pathway to inhibit inflammation and inhibit cartilage apoptosis (Wang et al., Citation2018). In addition, Previous studies have shown that the combined use of salmon calcitonin and HA can reduce the expression level of mRNA for NR4A1-3, which is a key regulator of OA. A study conducted by Ryan et al. connected salmon calcitonin and HA using CS and showed a good anti-inflammatory effect (Ryan et al., Citation2013). Coincidentally, Sladek et al. have also developed polyelectrolyte nanoparticles composed of salmon calcitonin, CS, and HA, which have been shown to have anti-inflammatory potential in mouse osteoarthritis model and safe in a horse arthritis model.
Chitosan microparticles (CMs) can also be used to deliver some polypeptide drugs (Sladek et al., Citation2018). According to Chen et al. dextran sulfate-CMs were prepared for efficient delivery of recombinant human bone morphogenetic protein (rhBMP-2). It was found that the particle size of the CMs was about 250 nm, and it could sustained-release rhBMP-2 for about 20 days (Xia et al., Citation2018). Further, it has been reported that hyaluronic acid (HAMA) hydrogel is used as a carrier to promote the treatment of CS (Masters et al., Citation2005). Hyaluronic acid can be synthesized under different methacrylic acid levels as compared with common hydrogels. It produces hydrogels with adjustable physical properties, including degradation, stiffness, and pore structure, as well as showing the prospect of tissue engineering application.
It has been found that chitosan microparticles (CMs) loaded with cordycepin and delivered by HAMA could promote autophagy in the treatment of osteoarthritis (Shamekhi et al., Citation2019). The experimental results showed that the drug could be released continuously for up to three days. Further, HAMA hydrogel delayed the progression of OA induced by surgery at 4 and 8 weeks as compared with the OA mice in the control group (Xia et al., Citation2017). The sustained release properties of CS have great application prospects in cartilage engineering with the help of hydrogel. In conclusion, CS can be widely used in the construction of joint sustained-release DDS.
3.2.2. Poly (lactic-co-glycolic) acid (PLGA)
Poly (lactic-co-glycolic) acid (PLGA) is a kind of degradable and functional polymer organic compound that has good biocompatibility, good performance of encapsulation and film-forming capacity. It is widely used in pharmaceutical, medical engineering materials, and the modern chemical industry. It is an ideal drug carrier due to its injectable, degradable, and sustained-release properties (Ding & Zhu, Citation2018). Currently, there have been many studies using PLGA to carry small molecule drugs to extend their residence time in the joint cavity. For instance, a research team reported the effective use of PLGA with fluvastatin to treat OA (Goto et al., Citation2017). In vitro release, experiments have shown that it could continuously release the drug within four weeks, and its therapeutic effect has been confirmed in the rabbit OA model. Moreover, Diacerein (DIA) has been noted as an important inhibitor of IL-1 for OA.
DIA-loaded poly (d-lactide-lactide-glycolide) are nanoparticles (DIA/PLGA nanoparticles), which are nearly spherical with a particle size of between 200 nm to 320 nm (Jung et al., Citation2020). It was found that diacerein could release from the nanoparticles for up to 9 weeks to achieve the aim of resisting inflammatory reactions and preventing cartilage degeneration. Notably, it was evident that the increase of IL-1β and TNF-α caused by the nanoparticles in the early stage after articular injection was precisely offset by the initial explosive release of DIA.
Synovitis is a common symptom of OA, and sustained-release DDS is also ideal for the treatment of synovitis. N. Bodick et al. compared the efficacy of FX006, a triamcinolone acetonide (TCA) in PLGA microsphere and single IA injection of TCA for synovitis treatment (Kumar et al., Citation2015). The researchers found that IA injections of 0.28 mg FX006 resulted in near-normal gait scores on days 1, 2, and 4 after the first reactivation, indicating good analgesia. The second inflammatory reactivation on days 15–18 and the third inflammatory reactivation on days 29–32 were both effective and had no side effects. 0.06 mg dose of TCA showed significant improvement in gait scores only at the first reactivation, although it produced initial plasma concentrations approximately 10 times higher than those at the 0.28 mg dose of FX006 and comparable overall systemic TCA exposures. Since FX006 has the advantages of retention and sustained release, a single IA injection of FX006 can prolong the residence time of TCA in the joint. Significantly exert the analgesic effect of TCA, while effectively inhibiting synovitis, cartilage damage and osteophyte formation. Furthermore, it has been found that Rapamycin can activate autophagy to cope with the aging and apoptosis of chondrocytes under oxidation stress conditions. However, its use is limited by repeated joint injection and systemic toxicity, and this hence necessitated the synthesis of PLGA carrier for Rapamycin (Dhanabalan et al., Citation2020). The size of the PLGA carrier (900 nm-1 μm) allows Rapamycin to remain in the articular cavity for 30 days, effectively induces autophagy of human chondrocytes, and prevents cell aging. In addition, PLGA not only has a good sustained-release function but also can prolong the residence time of cationic polymer nanoparticles (cationic NPs) in the joint cavity, which are composed of PLGA as the matrix polymer.
Several research has indicated that due to the interaction of electrostatic cation NPs with endogenous hyaluronic acid (HA), a filamentous structure may form in the synovial fluid. The residence time in the knee joint can increase to 7 days as compared with the solution form. However, there is still a need for a longer retention time to reduce the frequency of IA injections and reduce discomfort in the patient. In a separate study, Kim et al. composed cationic PLGA/Eudragit RL nanoparticles, which were 170.1 nm in particle size and 21.3 mV in zeta potential. Notably, it was evident that the nanoparticles could remain in joints for 28 days after IA injection (Kang et al., Citation2015). Therefore, the study demonstrated that the cationic nanoparticle based on PLGA was a promising sustained release agent in joints.
Most PLGA formulations are spherical, but cube-shaped sustained-release DDS is also available. Decuzzi et al. established 20 × 10 μm shape-defined poly (D, L-lactide-coglycolide) acid microplates (μPLs) and sustained-release DEX in the joints. μPLs are fabricated by a strategy of sacrificing templates (Di Francesco et al., Citation2021). First, a silicon master template was created by the direct laser writing (DLW) method. Second, replicated with a polydimethylsiloxane (PDMS) template. Third, a polyvinyl alcohol (PVA) template was prepared by replicating the PDMS template. The template is filled with a drug-loaded polymer paste (PLGA). Finally, the μPLs are released into aqueous solutions by the stepwise dissolution of the sacrificial PVA template. Calculated as the ratio of the number of μPLs collected after PVA dissolution to the theoretical number of pores in the template, an approximate preparation yield of 40% was obtained. In vitro drug release kinetics were measured, in 500 μL of release medium simulating physiological conditions, the release of DEX was only about 4% within 8 h, and about 20% after 30 days. The results of in vivo retention experiments showed that Cy5-μPLs could remain in the cartilage surface, infrapatellar fat pad/synovium, and joint capsule of the in vivo OA mice model for more than 15 days. DEX-μPLs effectively protected articular cartilage and joint structure in the PTOA model. Not only that, μPLs could also carry short interfering RNA (siRNA) loaded nanoparticles (siNPs) of MMP13 () (Bedingfield et al., Citation2021). The findings showed that it could remain in the PTOA model for more than 10 days and maintained down-regulation of the MMP13 gene and reduced levels of MMP13 protein in the joints throughout the 28-day study. In conclusion, PLGA is a promising biomaterial that can be used in sustained-release DDS for OA disease.
Figure 4. Characterization of siNP-μPLs. (A) Particle size distribution of Cy5-siNPs before and after acetonitrile exposure. (B) MicroPlate size distribution. (C) μPLs loaded with Cy5-siNPs SEM image. (D) PVA template containing Cy5-siNPs (red) dispersed within PLGA paste (yellow-green) confocal microscopy image. (E) Harvested Cy5-siNP loaded CURC-μPLs confocal microscopy image. (F) Cy5-siNP-μPLs without CURC (red) confocal microscopy image. Reprinted with permission from ACS Publications (Bedingfield et al., Citation2021).
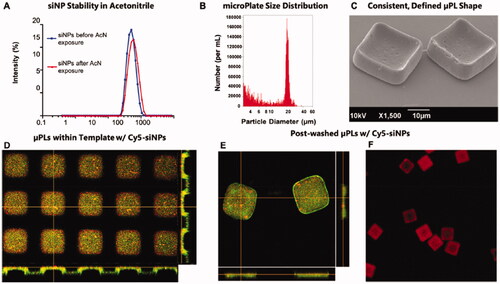
3.2.3. Hyaluronic acid (HA)
Hyaluronic acid is a disaccharide unit composed of D-glucuronic acid and N-acetylglucosamine, commonly used to construct drug delivery systems (Huang et al., Citation2021). It has good biocompatibility, degradability, non-inflammatory, nontoxic, and non-immune characteristics. There is natural HA in the synovial fluid, which plays an independent role in lubricating synovial joints. In addition, the interaction between HA and chondrocyte CD44 is also believed to act in the physiological and pathological processes of articular cartilage. Currently, IA injection of HA has become an important treatment method for OA (Jones et al., Citation2019). Therefore, HA, with the dual characteristics of treatment and drug loading, is a promising sustained-released DDS material.
However, exogenous HA is easily degraded in the joint cavity and has a high clearance rate. Therefore, researchers usually modify or protect HA to extend the retention time of HA-based DDS in the joints. For example, it is a feasible strategy to modify HA with heat-sensitive poly (N-isopropylacrylamide) (pNiPAM) polymer, which can make HA spontaneously form nanoparticles at the body temperature. According to another research, it was evident that HA modified by pNiPAM had better resistance to the degradation of hyaluronidase and could extend the residence time at the injection site (Maudens et al., Citation2018). During the 21-day monitoring period, it was found that the heat-sensitive particles stayed in the joint cavity. On the contrary, the free HA began to diffuse in 1 h after injection. Therefore, the nanoparticle effectively protected the cartilage, reduced pro-inflammatory cytokines, and maintained the thickness of the epiphysis. In addition, modification of HA with sulfuric acid groups can not only resist the decomposition of hyaluronidase but also make HA negatively charged, which is of great significance for binding the positively charged residues of the protein and thus improving ability of the modified HA to carry protein drugs (Li et al., Citation2012).
Apart from modifying HA, using HA to form a special three-dimensional structure is also another possible solution. Recently, gene silencing has been a popular direction to inhibit the development of OA. Hydrogels constructed by HA are used to replace viral or non-viral vectors for delivery of small iRNAs and hence no potential toxicity with a sustained-release profile can be achieved. According to Garcia et al. fibrin, hyaluronic acid (HA) hydrogels were used to deliver stem cells and ADAMTS5-targeted ASO, which could continuously release Gapmers to treat OA (Garcia et al., Citation2019). Coincidentally, in a separate study, Cai et al. used chitosan and HA to produce hydrogels, which also achieved the described effects. In particular, Cai et al. also considered the binding of HA to CD44 in chondrocytes to recruit chondrocytes with high CD44 expression (Cai et al., Citation2017).
Therefore, it is evident that HA-based drug delivery systems usually have excellent characteristics, which shows the bright prospect of HA in the field of drug delivery, such as easy modification, sustained release, active targeting, and low side effects.
3.2.4. Polyester amide (PEA)
Polyamide ester (PEA) is a new type of polymer based on amino acids, aliphatic dicarboxylic acids and aliphatic α-ω diols. PEA is biodegradable and amphiphilic and is very stable in PBS. Multiple studies have shown it to be a durable and safe topical drug delivery platform (Andres-Guerrero et al., Citation2015; Peters et al., Citation2017; Tellegen et al., Citation2021). Besides, it is sensitive to serine proteases, which are rich in inflammatory environments, so PEA is suitable for DDS in the treatment of OA (Guo & Chu, Citation2009).
Thies et al. investigated the potential of celecoxib-loaded PEA microspheres as a DDS for the treatment of OA (Janssen et al., Citation2016). Interestingly, it had an automatic adjustment function: the degradation of PEA microspheres was more obvious in OA joints, and its degradation could be significantly inhibited by loading celecoxib. Because inflammatory cells could maintain the enzymatic degradation of PEA microspheres and celecoxib inhibited this process. Celecoxib-loaded PEA microspheres had a particle size of about 10–100 μm, which made it have excellent sustained release and retention characteristics. The study showed it had an initial burst release on the first day, about 15% of the total drug load was released. Subsequently, celecoxib could be sustained and slowly released for up to 80 days. Moreover, celecoxib-loaded PEA microspheres have good biocompatibility and extended retention in the joint cavity, which are beneficial for OA treatment.
In another study, the research team prepared PEA microspheres loaded with triamcinolone acetonide (TAA) (Rudnik-Jansen et al., Citation2017). Microspheres in PBS released TAA sustainably for more than 60 days and could effectively inhibit inflammation for at least 28 days. A near-infrared marker was used to track the in vivo fate of microspheres after administration into healthy rat joints or mildly collagenase-induced OA joints. It was found that the retention time could be up to 70 days. The results demonstrated that the microspheres provided a safe drug DDS that did not induce foreign body reactions and reduced TAA plasma levels. Synovial inflammation was significantly reduced after treatment.
All in all, PEA microspheres have good biocompatibility, allowing long-term retention in the joint cavity. In addition, responsiveness to inflammation makes it an autoregulatory drug delivery system. PEA microspheres are ideal materials for the construction of OA sustained-release DDS.
3.2.5. Other biomaterials
At present, several biomaterials have been used to develop sustained-release DDS of OA drugs because of their good biocompatibility and stability. Apart from the above-mentioned biomaterials, peptides, cyclodextrin, heparin, and phospholipids may become ideal materials for synthesizing sustained-release DDS of different kinds of drugs. Some of these materials and their features were introduced in the present study.
First, there is a polypeptide which is a biologically active substance with low toxicity and good biocompatibility. It can be made into various carrier materials to control drug release. A previous study reported that Kimmerling et al. designed the cross-linked elastin-like polypeptide (xELP) for sustain-releasing inhibitors of IL-1 and TNF-α (Kimmerling et al., Citation2015). ELPs are derived from the pentapeptide sequence of native human elastin (VPGXG, where X is any amino acid), and their biocompatibility and non-immunogenicity have been demonstrated for medical applications. ELP has temperature-sensitive properties, and the temperature exceeds the phase transition temperature to form self-assembled particles to encapsulate drugs. On the one hand, this characteristic prolonged the release time of the drug. On the other hand, it protected the conformation of the drug from being destroyed, making it possible to encapsulate macromolecular proteins. Results of the study indicated that the polypeptide has a good anti-inflammatory effect, can be used in the treatment of post-traumatic arthritis and can be released continuously within seven days. Besides, Florine et al. used self-assembled peptide hydrogel to load Heparin-binding insulin-like growth factor 1 (HB-IGF-1) and released it to contain chondrocyte explants. It could be seen from the results of their study that self-assembling peptide hydrogel had the potential for sustained release, hence it was a feasible method to release drugs into the cartilage injury site (Florine et al., Citation2015).
Second, Heparin is a linear polysaccharide with an average molecular weight of 15KDa and strong acidity. It is a natural anticoagulant substance in animals that contains some positively charged proteins, such as tumor necrosis factor alpha activated gene-6 (TSG-6) (Liang & Kiick, Citation2014). According to Tellier et al. heparin microspheres can be used to deliver TSG-6 (Tellier et al., Citation2018). Results of the phase image analysis indicated that microparticles were 80 ± 60 μm in diameter. In vitro release experiment showed that it had a sustained-release function, whereas the in vivo experiment showed that it could reduce cartilage damage in OA rats.
Third, cyclodextrin is composed of glucose and it has the characteristics of nontoxic, has no side effects, and can be absorbed by the human body (Bonnet et al., Citation2015). Further, the structure of cyclodextrin is similar to that of HA, which is made up of a sugar backbone. The sugar skeleton has a circular structure and the potential to contain some small molecular drugs. A previous study reported that a high concentration of cyclodextrin polymer could make the corticosteroid release curve up to one week (Rivera-Delgado et al., Citation2018). This system relies on affinity interactions for sustained release and is suitable for any small molecule. Therefore, cyclodextrin polymer can contain anti-inflammatory drugs such as hydrocortisone, triamcinolone acetonide, and dexamethasone, which could be released slowly in the articular cavity for a week to treat OA.
Fourth, the hydrogel has good biodegradability, superior biocompatibility, and low immunogenicity. Injectable materials such as hydrogel or hydrogel microspheres have been widely studied for the application of IA injection in the treatment of OA (He et al., Citation2017). In the present study, it was evident that hydrogel based on glycerol monooleate can carry hydrophilic or hydrophobic anti-inflammatory drugs and maintain drug release within a few weeks. In addition, in vitro experiments showed that the hydrogel has antioxidant and anti-inflammatory properties and has no toxicity to cells. Previous studies on OA rabbit models indicated that the hydrogel carrier or drug is well tolerated, does not have harmful effects on cartilage, and does not aggravate inflammation (Réeff et al., Citation2015). Moreover, it has been reported that thermosensitive hydrogel is also a good drug delivery vehicle. Furthermore, it can be transformed into fixed hydrogel states at body temperature and act as drug repositories in the articular cavity. Therefore, flurbiprofen thermosensitive hydrogel based on PCLA-PEG-PCLA triblock copolymer was developed, from which flurbiprofen can be released slowly, and the release time could be adjusted with the change of concentration in the copolymer. In the OA-induced animal model, the hydrogel showed good analgesia and relieved local inflammation in both short- and long-term (Li et al., Citation2020).
Fifth, Polylactic acid (PLA) is mainly produced by industrial polycondensation of lactic acid or ring-opening polymerization of lactide. It is a new type of biodegradable material, which is made from renewable plant resources such as corn starch (Castro-Aguirre et al., Citation2016). PLA is an ideal material for sustained release preparation. Allémann et al. compared the function of PLA microspheres and PLGA microspheres to load p38α/β MAPK inhibitor PH-797804 (PH) (Maudens et al., Citation2018). In in vitro drug release experiments, PH-loaded PLGA microspheres (PH-MPs) released about 80% of the drug within 10–15 days. On the contrary, PH-loaded PLA microspheres (PH-NPPs) released only about 50% of the drug within 90 days. The researchers pointed out that PLA microspheres had long-term retention, good biocompatibility, high drug loading, weak burst release and sustained drug release for several months. Therefore, it has a more obvious curative effect on OA than PH-MPs.
Sustained-release DDS can suspend in the articular cavity, slowly release the drug into the articular fluid and then treat the entire joint. Or can be retained in synovial tissue, which directly releases the drug into the articular capsule and exerts a curative effect on immune cells or fibroblasts. Whereas, for the drugs acting on articular chondrocytes or MSCs, a longer residence time can provide certain conditions for the drug to enter the cartilage. However, the dense, negatively charged cartilage matrix is still an insurmountable obstacle. There is a need for a drug delivery system to make the drug penetrate ECM. Therefore, in the next section of the present study, the permeable drug delivery system was discussed.
3.3. Permeable DDS
The use of sustained-release DDS can extend the retention time and improve the efficacy of drugs in the articular cavity. Besides, it can reduce adverse reactions caused by large doses or frequent injections. However, drugs acting in the articular cavity or synovial membrane can only alleviate the symptoms of OA and delay the process of OA, they can’t reverse or even cure OA. Chondrocytes are the only cell type in cartilage tissue. Although they only constitute a small part of cartilage tissue, they play an essential role in expressing and secreting extracellular matrix and maintaining cartilage stability. The development of OA involves many changes in the phenotype of chondrocytes and metabolic disorders (Goldring & Goldring, Citation2016). Bone marrow-derived MSCs are located in the subchondral bone deep in the cartilage and can migrate to the injured site and differentiate into chondrocytes when injured (Zhang et al., Citation2016). Therefore, therapy targeting MSCs or chondrocytes is the key to reversing OA and even curing osteoarthritis. There is a need to use DMOADs that can promote the differentiation of MSCs and stimulate the proliferation of chondrocytes. Further, there are some DMOADs, like growth factors, cytokine receptor antagonists, and inhibitors of metabolisms enzymes, which have a good therapeutic effect on OA in preclinical studies but fail in clinical studies (Karsdal et al., Citation2016). The main reason for the failures is that the drugs cannot be delivered to chondrocytes or MSCs, which are their target cells. Therefore, DDS should not only continuously release the drugs but also have permeability so that it can quickly and deeply penetrate the articular cartilage and hence successfully deliver drugs to chondrocytes or MSCs. The present study also introduced some research on permeable DDS and some permeable DDSs as summarized in .
Table 2. Drug delivery systems with enhanced penetration profile for OA therapy.
3.3.1. Avidin
Avidin is an alkaline protein with a positive charge on its surface, and the particles are 8 nm in size. Avidin has weak and reversible electrostatic interaction with cartilage, and its small volume makes it suitable for OA drug delivery. The extracellular matrix is a kind of avascular tissue, which consists of GAG with a high negative charge. Therefore, avidin with a positive charge can effectively penetrate ECM. A previous study has confirmed that avidin could penetrate the whole layer of articular cartilage within 6 hours and remain in the joint for 7 days, with a half-life of 29 hours. Besides, avidin can also penetrate the full thickness of bovine cartilage in one day, while neutravidin can penetrate only half of the sample thickness in four days (Bajpayee et al., Citation2014).
It is feasible to use it to deliver drugs into deep cartilage because avidin has excellent penetration ability. Research has shown that avidin can combine with dexamethasone (Dex) and gradually release it for 3 weeks (Bajpayee et al., Citation2017). In addition to the characteristics of sustained release, avidin can also infiltrate cartilage to deliver Dex by using the effect of electric charge. The avidin binding Dex had a stronger ability to inhibit catabolism and more significantly reduced the loss of GAG as compared with the free Dex. Similarly, another study showed that avidin could penetrate the whole layer of rabbit cartilage to deliver Dex, keep it for at least 3 weeks, reduce IL-1α induced cell apoptosis, and relieve inflammation (Bajpayee et al., Citation2016).
However, the drug loading potential of avidin is limited because of its small particle size. If the concentration of the drug is required to reach a therapeutic concentration in the target cells, the dose of avidin needs to be increased. However, when the dose of avidin is more than 20 mg, it may also accelerate cartilage damage. Therefore, to avoid cartilage damage, He et al. designed an avidin-based drug delivery nanostructure which is called multi-arm avidin and combined it with Dex (mAv-Dex) () (He et al., Citation2020). It has 28 covalent binding sites and can transfer OA drugs of small molecules to the chondrocytes with high efficiency, hence enhancing the retention time of the drugs in healthy or OA cartilage.
Figure 5. Multi-arm avidin drug delivery system. (A) The nano-constructed multi-arm avidin and the small molecular drug dexamethasone (mAV-Dex) was assembled through the hydrolyzable ester conjugates succinic acid, glutaric acid and phthalic anhydride. The particles penetrate through the cartilage quickly through electrostatic action and reversibly bind to articular cartilage to transform it into a drug repository, (B) Drug release profiles of Dex from different formulations. (C) Histological and immunohistochemical analysis of cartilage. After 16 days of culture, the cartilage explants were stained with lycopene-O and solid green (GAG) or type II collagen immunostaining to determine the protective effect of mAV-Dex on cartilage matrix. Reprinted with permission from Elsevier (He et al., Citation2020).
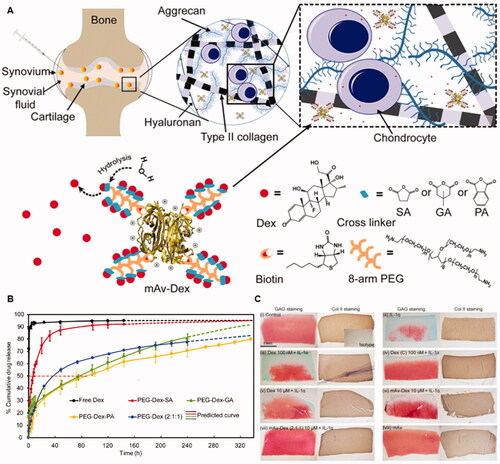
In the bovine OA cartilage model, the experimental results showed that a low dose of mAv-Dex was more effective than unmodified Dex in inhibiting the cartilage loss, chondrocyte apoptosis, and inflammatory response, which was induced by cytokines. Furthermore, it was found that only less than 1 μM avidin was needed for the synthesis of mAv-Dex, and the dose would not lead to cartilage damage or chondrocyte cytotoxicity. Therefore, mAv-Dex can not only penetrate cartilage-like avidin but also avoid side effects caused by a high dose of avidin. Avidin has a suitable size and positive charge, which helps it to penetrate through the full thickness of cartilage and deliver drugs to chondrocytes or MSCs for the treatment of OA disease. However, they are still easy to be quickly removed by the synovial lymph system due to their small particle size, thus resulting in reduced drug utilization, insufficient therapeutic effect, and may have some systemic toxicity effects. Therefore, It’s need to find a way that can extend the residence time of avidin and maintain its small particle size.
3.3.2. Dendrimer
Dendrimers are polymeric nanoparticles with branched structures that have a wide range of applications in the field of biomedicine, such as drug delivery, gene transfection, imaging, or use as antimicrobials (Fox et al., Citation2018). PAMAM dendrimers, the commonly used dendrimers in the design of drug delivery systems, are composed of layered dendrites which radiate from a central initiator core. Each branch layer is called a generation (G) (Tomalia, Citation2012). Although its size will increase with the growth of G, it is still very small (<10 nm) and has dense surface cationic primary amines (64–256 for G4–G6) for functionalization. Studies have shown that poly (ethylene glycol) (PEG) can be used to modify amino groups on the surface of dendrimers to control their charge and improve biocompatibility (Kim et al., Citation2008; Kono et al., Citation2008). PAMAM dendrimers can be divided into three main groups based on the mechanism of drug loading: (i) central void nucleus (via molecular capture); (ii) branch layer (via hydrogen bonding); (iii) surface groups (via charge interaction) (Chauhan, Citation2018; Kim et al., Citation2018). PAMAM dendrimers are ideal for DDS in the treatment of OA due to their small size, adjustable positive charge, and their excellent drug-carrying ability.
PAMAMs can be used to deliver various growth factors, e.g. insulin-like growth factor 1 (IGF-1), fibroblast growth factor 18 (FGF-18) and bone morphogenetic protein 7, and insulin-like growth factor 1 (IGF-1), which have been shown to treat OA by inhibiting chondrocyte apoptosis and promoting ECM synthesis. Geiger et al. designed a PEGylated PAMAM dendrimer nanocarrier with a particle size of about 10 nm. The amines on the surface of PAMAM can be modified with polyethylene glycol (PEG) oligomer to strictly control the charge and improve biocompatibility () (Geiger et al., Citation2018). PEGylated PAMAM dendrimers combined with IGF-1 can penetrate deep into the human thick bovine cartilage tissue in two days and maintain the growth factor levels in cartilage above the effective therapeutic concentration over 30 days. In addition, the injection of PEGylated PAMAM dendrimer-IGF-1 can effectively protect the cartilage of rats and reduce the medial tibial cartilage thickness and the size of osteophytes, demonstrating a more attenuated cartilage degradation condition as compared with free IGF-1 injection in the OA rat model.
Figure 6. PEGylated dendrimer-IGF-1 conjugates were designed for penetrating cartilage for chondrocyte drug delivery. (A) Schematic illustration for the fates of particles with different sizes and surface potential after intra-articular injection. (B) Chemical structure of G4 PAMAM dendrimer. (C) IVIS images of rat knee joints after intra-articular administration of fluorescent IGF-1 for 28 days. (D) Quantitative analysis of IVIS images over time. (E) Time at therapeutic concentration for each delivery method. (F) 3 D reconstruction of multi photon microscopy images of cartilage at day 6 post injection. Reprinted with permission from AAAS (Geiger et al., Citation2018).
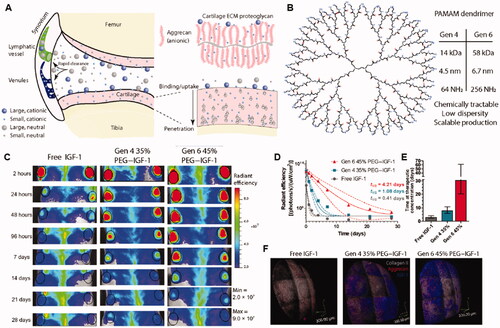
PAMAM can also be used to deliver small-molecule drugs, such as Kartogenin (KGN), which promotes the differentiation of MSCs into chondrocytes (Johnson et al., Citation2012). Hu et al. developed an intra-articulate injection PEG-PAMAM nanocarrier for delivering KGN effectively (Hu et al., Citation2017). They showed that free Cy7 was almost eliminated within 24 h, while Cy7-PEG-PAMAM still produced a light signal after 21 days. Their study demonstrated that PEG-PAMAM greatly increased the residence time of KGN in the articular cavity, thereby improving its curative effect on OA. However, the study did not discuss the ability of PEG-PAMAM to penetrate cartilage. Apart from PAMAM dendrimers, there are other dendrimers with similar properties that are suitable for use as permeable DDS.
For example, Schneider et al. developed dendritic polyglycerol sulfate (dPGS) that could permeate up to about 50 μm into cartilage chips and was rapidly absorbed by chondrocytes (Schneider et al., Citation2015). Dendritic polyglycerol sulfate (dPGS) itself can interfere with the process of leukocyte adhesion by binding to selectins and has complement inhibitory properties. As a suitable nanocarrier polymer, dPGS takes glycerol unit as the backbone, and different numbers of sulfuric acid groups replace the hydroxyl groups on the branched chain, so it has good ‘modifiability’ and biocompatibility, even at a high dose (10-3 mol/L), and did not affect the activity of chondrocytes. Although dPGS failed to inhibit the synthesis of pro-inflammatory cytokines, TNF-α and IL-6, it produced anti-inflammatory effects by upregulating the expression of anti-inflammatory factor IL-10. Another study also demonstrated that dPGS inhibited the progression of OA by protecting the cartilage and inducing anti-inflammatory effects in knee joint osteoarthritis (Schneider et al., Citation2015). Dendrimer nanotechnology has the potential to be the permeable DDS for the treatment of OA due to its small particle size, easy-to-modify surface groups, and multiple drug loading methods. However, there are currently no suitable tools for predicting the encapsulation potential of any drug. Therefore, to successfully develop a DDS based on dendrimers, different kinds of dendrimers need to be screened to find the right combination. Taking the PAMAM dendrimer as an example, there is a need to identify a suitable surface modification method for its generation (Chauhan, Citation2018).
3.3.3. DDS with targeting peptides
DDS modified with targeting peptides can improve the targeting ability of the systems and promote drug penetration into cartilage. At present, targeting peptides mainly include affinity ECM peptides and affinity chondrocyte peptides. Although PEG-modified PANAM dendrimers can penetrate cartilages, a study showed that PEG-PAMAM-RB (PP-RB) without a chondrocyte affinity peptide (CAP) could only penetrate the cartilage surface within 48 hours, while CAP modification (CAP4-PP-RB) increased the penetration to a greater extent (Hu et al., Citation2018). These findings suggested that the permeability properties of PAMAM dendrimers are not sufficient and can be improved using targeting peptides. Apart from PAMAM dendrimers, other nanoparticles can also be linked to targeting peptides to improve penetration. After five rounds of biological elimination, Rothenfluh et al. identified a WYRGRL ligand that can bind to collagen IIα1 and then connected it with poly (propylene sulphide) nanoparticles to construct PPS-WYRGRL nanoparticles (Rothenfluh et al., Citation2008). The results of in vivo experiments indicated that compared with non-targeted nanoparticles, the accumulation of targeted nanoparticles in the ECM increased by 44.8 times after 24 hours and by an average of 71.7 times after 48 hours; the accumulation of targeted nanoparticles in chondrocytes increased by 3.7 times after 24 hours, 5.0 times after 48 hours, and 4.2 times after 96 hours. Moreover, they also compared the penetration of targeted nanoparticles in the cartilage between average particle size of 38 nm and 96 nm and found that nanoparticles with a smaller particle size were more likely to penetrate the ECM. Hu et al. generated permeable DDS by combining AcWYRGRL with DOTAM (1,4,7,10-tetraazacyclododecane-1,4,7,10-tetraacetic acid amide) (Hu et al., Citation2015). They then compared the permeability of AcNH-DOTAM-Cy5.5 (non-targeting control), AcWYRGRL-DOTAM-Cy5.5 (1 collagen II targeting peptide), AcYRLGYW-DOTAM-Cy5.5 (1 scrambled peptide), AcWYRGRL-DOTAM-Cy5.5 (3 collagen II targeting peptide) and AcYRLGYW-DOTAM-Cy5.5 (3 scrambled peptide) in pig cartilage explants. They found that increase in the number of linked affinity peptides increased the permeability of the compounds. The accumulation of the DDS with three targeted affinity peptides in pig cartilage was 3.1 times higher than that of the DDS with one targeted affinity peptide, and 36.6 times higher than that of the non-targeted DDS. Further, in vivo evaluation in mouse knee joint cartilage showed that the retention time of compounds with one and three target affinity peptides was longer than that of compounds with one and three non-target affinity peptides. These findings demonstrated that the penetration and retention time of a DOTAM-conjugated drug could be enhanced through various modifications. What’s more, Zhang et al. synthesized about 25 nm lipopolymer hybrid also functionalized with collagen-binding polypeptide WYRGRLC (ctLP-NPs) for targeted cartilage (Ai et al., Citation2021). They labeled ctLP-NPs and non-targeted nanoparticle LP-NPs with DiD and verified their permeability to cartilage. The results showed that the fluorescence signal of LP-NPs was lower than 10% when the cartilage depth increased to 40 μm, while the fluorescence signal of ctLP-NPs was still stronger than 10% when the cartilage depth increased to 100 μm (). The permeability enabled ctLP-NPs to stay in the joint cavity for a longer time and improve the therapeutic effect of OA. Thus ctLP-NPs encapsulated with MK-8722, an activator of AMPK (ctLP-NP(MK)), showed better efficacy than LP-NP (MK) in a mouse model of OA. In addition to the collagen affinity peptide WYRGRL, there were also studies that reported different targeting peptides. Cartilage affinity peptide (CAP, DWRVIIPPRPSA) is one of them.
Figure 7. Permeation and retention characteristics of ctLP-NPs in the cartilage. (a) Representative fluorescence images of DiD-labeled LP-NPs (top) or ctLP-NPs (bottom) in femoral head sections. (b) Relative fluorescence intensity of cartilage incubated with LP-NPs or ctLP-NPs for 24 h. (c) Quantitative analysis of penetration depth of nanoparticles. (d) Fluorescence images of ctLP-NPs and LP-NPs at different time points. (e) Fluorescence intensity of the nanoparticles in the knee joints. Reprinted with permission from Wiley (Ai et al., Citation2021).
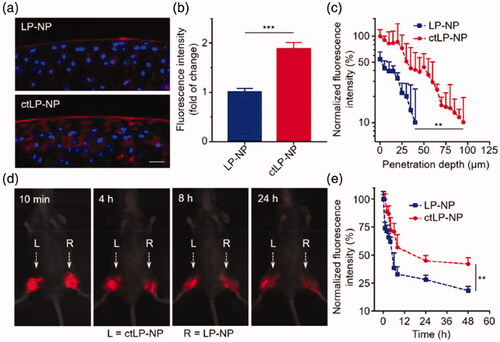
Pi et al. identified CAP using phage display technology and used it to covalently modify Polyethylenimine (PEI) to generate a non-viral vector for cartilage-targeted therapy (Pi et al., Citation2011). They found that the amount of CAP-linked vectors was more than that of interference peptide-linked vectors in cartilages of a rabbit knee joint model. Confocal microscopy showed that most of the CAP-linked vectors penetrated the deep cartilage and were absorbed by chondrocytes 48 hours after injection, but the interference peptide-linked vectors could not penetrate through ECM into cartilage. In another research, Liu et al. synthesized a novel magnetic resonance contrast agent nanoparticle modified with CAP and carried hesperetin (Hes) (Hes-Gd2(CO3)3@PDA-PEG-DWpeptide, HGdPDW) (Ouyang et al., Citation2019). It is about 110 nm in different solutions and about +6 mV zeta potential. The MRI/IVIS dual-mode imaging showed that HGdPDW bound to cartilage in knee joints of OA mice was stronger than that of HGdP 12 h after IA injection. Furthermore, the researchers evaluated the biodistribution of nanoparticles. The results indicated that Gd3+ was almost accumulated in cartilage in the GdPDW group. However, a large amount of Gd3+ in GdP group was distributed in the liver, suggesting that GdPDW have specific cartilage targeting effects in vivo. Due to this ability, GdPDW inhibits apoptosis by inhibiting the expression of TLR-2 in chondrocytes, thereby reducing the progressive damage and degeneration of OA articular cartilage in vivo. It is a pity that the permeability of nanoparticles was not verified in this article.
During OA progression, cartilage damage exposes collagen antibody binding sites, therefore type II collagen antibody is also an excellent agent for targeting cartilage. Duvall et al. attached a Col2 monoclonal antibody (mAbCII) to the surface of polymeric siRNA nanoparticle complexes (siNPs) (Bedingfield et al., Citation2021). They build a nanoparticle that could target and penetrate cartilage with approximately 100 nm and approximately zeta potential. Researchers confirmed the ability of mAbCII-siNPs to penetrate cartilage and deliver drugs to chondrocytes. We found that 12 h after IA injection, mAbCII-siNPs were visible on the surface of articular cartilage and in single chondrocytes, and there was also a signal of non-targeted nanoparticle mAbCtrl-siNPs on the surface of articular cartilage, but no signal in chondrocytes. The results indicated mAbCII-siNPs have better penetration ability. Besides, mAbCII-siNPs achieved up to 90% silencing of target genes in vivo, and in a 6-week study, gene silencing remained effective 1 week after final treatment, reduced 80% MMP13 expression. Ultimately protected cartilage integrity, improved total joint histopathology, reduced ectopic mineralization and osteophyte formation, and reduced subchondral bone destruction in the PTOA model.
In summary, the use of targeting affinity peptides or targeting antibodies is a promising strategy to improve the permeability of DDS.
3.3.4. Carbon-Based DDS
Carbon is one of the basic elements that constitute organic life and has been widely used in biology and commerce, including the field of nanomaterials. Currently, the widely used carbon-based nanomaterials can be divided into three types: zero-dimensional (fullerenes, carbon dots, nanodiamonds), one-dimensional (carbon nanotubes), and two-dimensional (graphene) nanomaterials. They all have strong plasticity, and studies have shown that they could be used in vivo and in vitro to promote the regeneration of bone, cartilage and osteochondrocytes. According to existing literature, carbon nanomaterials are rarely used in cartilage regeneration medicine, and most of them are dedicated to the 3 D reconstruction of cartilage in tissue engineering research (Yudoh et al., Citation2007; Shen et al., Citation2018; Liu et al., Citation2019; Meng et al., Citation2020). However, tissue engineering still faces many problems. For example, the remodeling process is sometimes accompanied by chronic inflammation, which may hinder cartilage regeneration; lack of cell sources; abnormal differentiation of cultured cells in vitro; low survival rate after in vivo transplantation; and restricted exogenous chondrocyte transplantation (Liu et al., Citation2021). Therefore, we believe that it is better to promote cartilage repair by using permeable carbon-based DDS to promote the synthesis of ECM by chondrocytes and promote the differentiation of MSCs into chondrocytes in OA patients.
Mao et al. prepared single-walled carbon nanotubes (SWCNTs) modified with collagen (collagen-SWCNTs) of about 235.42 nm in length and with good stability in water (Mao et al., Citation2013). They then evaluated the cellular uptake of collagen-SWCNTs by bovine chondrocytes during cell culture. The chondrocytes were first cultured in a medium containing collagen-SWCNTs for three days and thereafter maintained in a medium without collagen-SWCNTs. They found that the cellular uptake of SWCNTs increased even after the culture medium was changed to a culture medium without collagen-SWCNTs. To explain this phenomenon, the team quantitatively studied SWCNTs in the ECM and discovered that the absorption of SWCNTs by the cells increased, while the number of SWCNTs in ECM decreased when the culture medium was changed to medium without SWCNTs. These results proved that SWCNTs could penetrate ECM and be taken up by chondrocytes. Although this study proved for the first time that SWCNTs could penetrate ECM, the findings were not validated in vivo experiments.
However, this gap was later filled by Sacchetti et al. who developed polyethylene glycol (PEG) chain-modified single-wall carbon nanotubes (PEG-SWCNTs) as DDS for delivery of gene inhibitors to chondrocytes () (Sacchetti et al., Citation2014). The PEG-SWCNTs had diameters of less than 10 nm and lengths ranging from a few tens to several hundreds of nanometers. In vivo experiments confirmed that PEG-SWCNTs could easily enter the dense cartilage ECM, transfer into chondrocytes, and deliver gene inhibitors without affecting cartilage homeostasis or causing systemic side effects. Carbon nanotubes have unique shapes compared with other spherical DDS, which may explain their ability to penetrate ECM. In conclusion, carbon-based DDS, particularly carbon nanotubes, can be used to generate permeable DDS.
Figure 8. Single-walled carbon nanotubes with PEG modification (PEG-SWCNT) were prepared for antisense oligonucleotide delivery into chondrocytes. (A) schematic introduction for the fate of PEG-SWCNT after intra-articular injection. (B) Joint sections stained with Safranin-O and counterstained with Fast green and Hematoxylin. (C) IL-1 and TNF-α expressions were examined by immunohistochemical assay. Reprinted with permission from ACS publications (Sacchetti et al., Citation2014).
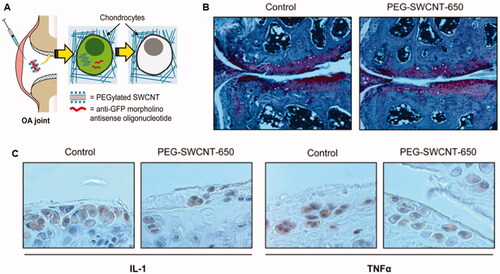
3.3.5. Lipid nanoparticle (LNP)
Lipid nanoparticles (LNP) simulate cell membrane forming cells with a diameter of about 100 nm, and the components include neutral lipids, cationic lipids, cholesterol, and PEG-lipids. LNP is one of the most widely used delivery systems for RNA drug research. Compared with other types of nucleic acid drug delivery systems, LNP has many advantages, such as high nucleic acid encapsulation efficiency and effective transfection of cells, strong tissue permeability, low cytotoxicity and immunogenicity, and more conducive to the delivery of drugs. These advantages make LNP an excellent nucleic acid delivery system (Cullis & Hope, Citation2017). Some researchers found that LNP has the function of penetrating cartilage.
Wei et al. synthesized a positively charged LNP and carried Indian Hedgehog (Ihh) small interfering RNA (LNP-Ihh siRNA) (Wang et al., Citation2018). Its particle size is about 67 nm. Ihh is a gene closely related to the development of human OA, and several studies have shown that blocking its expression is a way to treat OA (Lin et al., Citation2009; Zhou et al., Citation2014). LNP-siRNA had extremely high transfection efficiency in vitro and could transfect almost 100% chondrocytes. To evaluate the permeability of LNP-siRNA, scientists incubated the whole pig cartilage with it for 48 h. Then frozen sections were detected under a confocal microscope. The results showed that red fluorescence signals could be detected in the shallow and deep layers of porcine cartilage treated with LNP-beacon. However, no red fluorescence signal was detected in free beacon-treated porcine cartilage. It was proved that LNPs had good penetration ability in porcine cartilage and could transfer probe into chondrocytes in vitro. They further evaluated the drug delivery effect of LNPs in normal mice and rats. They injected LNP-beacon and free beacon in the right knee of mice and rats, respectively. 72 hours later, the specimens were observed by a confocal microscope. The results showed that there was no red fluorescence signal in the articular cartilage injected with the free beacon. On the contrary, red fluorescence signal could be detected in the articular cartilage injected with LNP-beacon, indicating that LNP also had excellent permeability in vivo. Furthermore, the researchers proved that LNP successfully transfected siRNA into chondrocytes in vivo. The strong permeability enables LNP-Ihh siRNA to effectively treat OA rats. The strong permeability and transfection efficiency enable LNP-Ihh siRNA to effectively treat OA rats. It significantly inhibited MMP-13, Runx2, and type X collagen levels in rat cartilage and significantly increased type II collagen and aggrecan levels. In summary, LNP is an effective and promising platform for RNA drug delivery, and its good permeability to cartilage makes it an ideal choice for the treatment of OA.
In the next section, we focus on factors that affect DDS permeability and further discuss how to modify the physicochemical properties of DDS to enhance penetration.
4. Rational design of OA permeable DDSs
Articular cartilage has a complex structure and dense ECM that poses a challenge in drug delivery as it prevents drugs of nanometer-scale from entering its deep sit. The permeability of DDS is influenced by factors such as particle size, size distribution, surface charge, targeting and biocompatibility. In the following sections, we summarize the penetration DDS listed above and suggest a reasonable strategy for designing and constructing permeable DDS by discussing these factors.
4.1. Particle size
Particle size is one of the most important factors that affect DDS permeability. To improve the permeability of DSS, the particle size of the drug needs to be adjusted to a suitable range. A review by DiDomenico et al. discussed the hierarchical pore system in cartilage-from the gap of 4 ∼ 6 nm between GAG chains to the gap of 5 0 ∼ 100 nm between collagen fibers and how the diffusion rate and radius are inversely proportional in the particle radius range of 0.1–16 nm (DiDomenico et al., Citation2018). In addition, some studies have shown that although the volume diffusivity and partition coefficient are highly dependent on the size of solutes, not all solutes (especially large solutes) show uniform diffusivity in the whole cartilage. The smallest (1.75 nm) and largest (15.3 nm) dextrans had the highest diffusivities on the surface of the cartilage and statistically similar diffusivities elsewhere. Interestingly, the 5.7 nm and 7.4 nm dextrans showed the opposite trend, displaying the lowest diffusivities on the surface and the highest diffusivities in the middle and deep zones (Leddy & Guilak, Citation2003). These results were similar to the results of another study. In the range of 150–350 μm of the cartilage surface, the solute with a radius of 2.35 nm, 3.25 nm, 5.45 nm and 7.59 nm had the highest local diffusion rate (DiDomenico et al., Citation2017). The solute diffusion rate is also affected by the diffusion direction, and large particles are more susceptible to changes in collagen composition and orientation than small particles (Leddy et al., Citation2006). Bajpayee et al. showed that solutes with hydrodynamic diameters of less than 10 nm could penetrate the whole layer of bovine cartilage explant, while particles with diameters of 15 nm were trapped on the surface due to space hindrance. A study demonstrated that a 7 nm affinity peptide successfully penetrated ECM and could be used as a drug carrier (Bajpayee et al., Citation2014). Another study showed that 4.3 nm dextran could penetrate the whole layer of normal bovine cartilage in 24 hours, while 10 nm dextran only penetrated half of the tissue thickness in 4 days (Bajpayee et al., Citation2014). The effective pore size of ECM may be enlarged during OA due to corrosion of cartilage, allowing the entry of larger particle sizes into cartilage tissue. As a result, the effective pore size varies with the severity of OA. It has been reported that NPs with sizes between 100 nm to 300 nm selectively enter osteoarthritic cartilage tissue but not healthy cartilage (Jiang et al., Citation2018). Bajpayee et al. further explored the difference in penetration depth of positively charged QD and amino-modified neutral QD in normal cartilage and OA cartilage. The results of in vitro experiments showed that 15 nm QDs were trapped in the superficial area of healthy cartilage within 24 h, but they could penetrate deep into OA cartilage. In this study, trypsin-treated bovine cartilage was used as an in vitro OA cartilage model, and about 40% GAG was retained in the cartilage. It was proved that under pathological conditions, the loss of GAG enabled large particles that could not enter cartilage to enter () (Bajpayee et al., Citation2014).
Figure 9. (A) Confocal images of non-functionalized Cd-Se QDs after 24 h absorption in normal cartilage explants. (B) Confocal images of non-functionalized Cd-Se QDs after 24 h desorption in normal cartilage explants. (C) Confocal images of amine-functionalized QDs after 24 h absorption in normal cartilage explants. (D) Confocal images of amine-functionalized QDs after 24 h desorption in normal cartilage explants. (E) Confocal images of non-functionalized Cd-Se QDs after 24 h absorption in trypsin treated cartilage explants. (F) Confocal images of non-functionalized Cd-Se QDs after 24 h desorption in trypsin treated cartilage explants. (G) Confocal images of amine-functionalized QDs after 24 h absorption in trypsin treated cartilage explants. (H) Confocal images of amine-functionalized QDs after 24 h desorption in trypsin treated cartilage explants. (I) Quantitative analysis of cadmium in the bath of Cd-Se red and green QDs that were absorbed into normal and trypsin treated bovine cartilage explants in 24 h. (J) Quantitative analysis of cadmium absorbed in 24 h that was retained inside the cartilage explants after 24 h desorption into 1X PBS for red and green QDs and into 10X PBS for green QDs only. Reprinted with permission from Elsevier (Bajpayee et al., Citation2014).
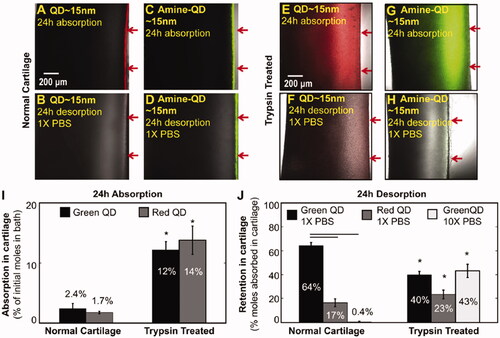
In summary, the permeation of drugs into articular cartilage can be enhanced by reducing the particle size. The depth of particle penetration into ECM is affected by its particle size, penetration direction and cartilage composition. Since OA induces varying degrees of cartilage damage, DDS is more likely to penetrate ECM during OA. Therefore, particles of a broad range of sizes can be used to penetrate the cartilage of patients with OA compared with healthy cartilage. In the future, there is a need to select DDS with particle sizes that correspond to the stage of OA progression to not only ensure the sustained release of drugs but also to improve the permeability of drugs.
4.2. Size distribution
While the particle size affects the penetration depth, size distribution affects osmotic dose. Enough drug particles are required to reach the drug target to get satisfactory curative effects. If particle size distribution is too wide, the number of particles with the appropriate size reduces, and fewer particles reach the drug target. An increase in dosage may solve this problem but is likely to be associated with adverse reactions. Consequently, improving the homogeneity of drug particles is the best way to ensure adequate drug concentration and reduce the probability of adverse reactions. Therefore, there is a need to develop technology for preparing homogeneous particles. Ansboro et al. were able to prepare homogeneous nanoparticles using a sulfonated polystyrene template, thus enhancing curative effects (Ansboro et al., Citation2014).
In conclusion, uniformity of particle size can increase the efficacy of drugs and reduce adverse reactions.
4.3. Surface charge
The permeation of drug carriers is also affected by the surface charges since ECM has a high negative charge due to the polymerization of collagen and aggregation proteoglycan by glycosaminoglycan. Therefore, drug carriers with positive surface charge are able to reach target cartilage cells. Bajpayee et al. evaluated the permeability of about 7 nm avidin and neutral avidin in isolated bovine cartilage and found that avidin could penetrate the full thickness of cartilage within 24 h, while neutral avidin could only penetrate the surface of cartilage (Bajpayee et al., Citation2014). This proved that positive surface charge is a critical factor in determining the permeability of drug carriers. Furthermore, they explored the effect of electrostatic interaction on avidin and its transport and binding properties. Also with avidin and its neutrality counterpart, neutral avidin comparison. They incubated cartilage with 1 × PBS, and about half of the neutral avidin absorbed was released within a day. After incubation with 1 × PBS for 15 days, however, only about 4% of the absorbed avidin was released. If the PBS concentration was increased to 10 ×, about 69% of the absorbed avidin would be released within a day, indicating that they incubated cartilage with 1 × PBS, and about half of the neutral avidin absorbed was released within a day. After incubation with 1 × PBS for 15 days, however, only about 4% of the absorbed avidin was released. If the PBS concentration was increased to 10 ×, about 69% of the absorbed avidin would be released within a day, indicating that the charge interaction enhanced the retention ability of avidin in cartilage. Reference Section 4.1, they compared the permeability of QD and amino-QD in healthy cartilage and OA cartilage (). The results showed that QD and Amine-QD could only remain in the shallow layer of normal cartilage within 24 h, but could penetrate OA cartilage, and QD had deeper penetration depth than Amine-QD. In addition, after incubation in 1 × PBS for 24 h, QD was still absorbed in normal cartilage but Amine-QD was not. The results were consistent in OA cartilage. Therefore, it is proved that both in healthy and OA cartilage, absorption and retention are inseparable from the interaction of charges, positive charged particles have stronger permeability and longer retention time.
Additionally, the strength of surface charge can also influence the permeability of drug carriers. Although positive surface charge promotes the permeability of drug carriers, higher surface charges do not necessarily translate into better permeability. Too much charge may cause irreversible interaction between the drug carrier and the ECM, thus preventing deeper penetration. Vedadghavami et al. used cationic polypeptide modified nanoparticles to demonstrate the effect of surface charge on the permeability of macromolecules in articular cartilage (Vedadghavami et al., Citation2019). They found that a surface charge of +14 had the best penetration. A surface charge of more than +14 induced an irreversible interaction between the cationic polypeptide and the ECM, which weakened the permeability of the polypeptide. Short-range hydrogen bonds and hydrophobic interactions between the cationic peptide and cartilage components can further stabilize the electrostatic binding. Arginine-rich cationic peptides have been found to bind more strongly to 24 negatively charged aggrecan GAGs in cartilage than lysine-rich cationic peptides. The effect of synovial fluid on charge in the articular cavity cannot be ignored. Some studies have shown that cationic DMAB NPs undergo charge reversal in synovial fluid, which has a negative effect on the penetration of nanoparticles (Brown et al., Citation2019).
Therefore, weak and reversible charge interactions between the carrier and ECM enhance penetration of the carriers and allow them to reach the target area. In addition, cationic peptides that modify DDS can be designed to further stabilize the electrostatic binding through hydrogen bonding and hydrophobicity and effectively avoid the influence of synovial fluid, to improve the permeability and retention time.
4.4. Targeting
Targeting plays an important role in the permeability of drug carriers (Kou et al., Citation2018; Citation2020; Citation2020; Citation2020; Citation2020; Wang et al., Citation2020; Zhang et al., Citation2020). Effective targeting, such as targeting articular cartilage or synovium, ensures drugs are specifically delivered to their sites of action. It is only when the drug is delivered and released in its target region that full efficacy of the drug can be achieved. Targeting of DDS includes passive targeting and active targeting. Passive targeting can be achieved by changing the physical and chemical properties of DDS, while active targeting can be achieved by using special peptides or antibodies to modify the DDS. Generally, active targeting is better than passive targeting. The passive targeting of DDS to the articular cartilage can be improved by reducing the particle size or adjusting the positive charge, as discussed above. We have demonstrated the active targeting of DDS using targeting peptides in Section 3.3.3.
In conclusion, effective targeting of DDS enhances the delivery of the drug to the target site. Passive targeting can be achieved by reducing the particle size and adjusting the surface charge, while active targeting can be achieved by modifying DDS with cartilage affinity peptides and antibodies. Both strategies improve drug permeability and efficacy.
4.5. Biocompatibility
Biocompatibility is generally defined as the interaction of biological material with the body at a specific location. When designing DDS, the biocompatibility of materials is one of the factors that must be considered. When evaluating biocompatibility, it usually starts from the properties of the material itself and the body's response. Whether the material itself is cytotoxic, teratogenic, carcinogenic, or mutagenic, all require rigorous experimental demonstrations. On the other hand, the interaction with the organism. Under the premise of intra-articular injection of the knee, the first challenge of DDS is to resist the degradation of the lytic enzymes in the joint cavity. Good biocompatibility means that DDS can maintain a certain retention time under the action of decomposing enzymes in the joint cavity to provide a more extended window period for DDS to penetrate cartilage. Q et al. modified the surface of HA with sulfate groups, which improved the problem of easy degradation of HA, and proved in subsequent experiments that it did not affect the activity of stem cells (Feng et al., Citation2017). In another study, Das et al. combined HA modifiers and gelatin to generate hydrogel with sustained release of white protein and dexamethasone that supported tissue penetration (Das et al., Citation2019). In addition, a ‘protein corona’ is adsorbed on the surface of DDS, like the situation in plasma. The size, charge, and surface properties of the DDS affect the amount and composition of the encapsulated ‘protein corona’. The adsorbed proteins can change the intrinsic properties of DDS and cause a shift in the properties of DDS, which may be a factor leading to difficulties in clinical translation. At the same time, the adhered ‘protein crown’ itself has biological activity, and the possible immune response is also a pain point for clinical transformation (Lundqvist et al., Citation2008; Kopac, Citation2021). We mentioned above that Wei et al. delivered siRNA with LNP to OA and achieved ideal results. However, a recent preclinical experiment has pointed out that LNPs may cause inflammation and some side effects in mice, which may be related to its ionizable lipid components (Ndeupen et al., Citation2021). Thus, researchers need to further study the biocompatibility of LNP to improve the success rate of clinical transformation.
In conclusion, finding inert materials, or achieving high biocompatibility through surface modification to prepare DDS can reduce adverse reactions and ensure the safety of DDS in cartilage. Ultimately achieving clinical translation is still the goal of researchers' efforts.
5. Summary and outlook
New DDS have shown their advantages in halting or delaying OA progression and relieving associated symptoms. Compared with conventional formulations, nano-formulations, microsphere formulations, hydrogels, and liposomes are superior in terms of prolonging drug retention time, increasing efficacy and improving patient compliance. Currently, an important trend in the development of drug delivery systems for osteoarthritis is to improve the penetration capacity of drugs so that they can permeate the cartilage matrix and act on chondrocytes or MSCs, thereby reversing or even curing osteoarthritis. Here, we reviewed some of the characteristics of drug delivery systems with good cartilage matrix permeability. After intra-articular administration, a certain degree of targeting is required. Drugs targeting the cartilage matrix can improve the accumulation of drugs in the cartilage and provide the necessary preconditions for the next step of penetration. Furthermore, based on the negative charge and nano-sized pore of the cartilage matrix, many drug delivery systems have been ingeniously designed to have the smallest possible size, positively charged surfaces and specific geometric structures like tubular bodies. We also summarized the challenges that are likely to be encountered in the development of permeable drugs, as well as possible solutions, in the hope of providing some ideas for researchers.
First, we believe that a comprehensive understanding of the dynamic structure of articular cartilage is necessary. Articular cartilage is not a homogeneous structure, both on a ‘temporal and spatial’ scale. On the spatial scale, the collagen fiber content in the extracellular matrix, the angles of alignment, and the gaps vary greatly at different depths of the articular cartilage. On the temporal scale, the process of OA is often accompanied by degeneration of the articular cartilage, and therefore the permeability of drug delivery systems through the cartilage matrix at different stages of OA is different. Therefore, the design of penetrating drug delivery systems should consider the required penetration depth and the time course of osteoarthritis.
Secondly, we should consider the characteristics of DDS mentioned in Section 4 to design a high-quality permeable DDS. For instance, although reducing the particle size can increase the penetration of the drug into the cartilage tissue, it is associated with insufficient drug loading capacity. As a result, it is not advisable to blindly reduce particle size when improving permeability. Therefore, the future development of permeable DDS is faced with the challenges of balancing between the drug loading capacity and permeability and ensuring sufficient drug release at the target site. In addition, reducing the particle size may also make the DDS susceptible to elimination, which may result in insufficient permeation doses to achieve the desired therapeutic effect. One possible solution is to design a DDS with a combination of sustained-release and permeable delivery, like a combo. For example, Rothenfloh et al. proposed targeting type II collagen in the cartilage matrix and using the complex three-dimensional structure of the ECM to act as a reservoir for DMOADs, which offers great possibilities for the penetration of DMOADs into the ECM (Rothenfluh et al., Citation2008). In addition, dual-stage nano-DDSs can be developed in which smaller-sized nanoparticles are encapsulated within larger-sized nanoparticles, and they can both carry drugs. Nanoparticles with larger diameters have better retention and sustained release functions, and can carry anti-inflammatory drugs or analgesics, while nanoparticles with smaller diameters can penetrate the ECM to release drugs at the target chondrocytes.
Otherwise, the difference between the transport and retention of permeable DDS in normal and OA cartilage is not fully studied. In Sections 4.1 and 4.3, we referred to Ambika G. Bajpayee et al. exploring the differences in permeability of particles with different particle sizes and surface charges to normal cartilage and OA cartilage. However, only in vitro bovine cartilage samples for evaluation, the lack of in vivo experimental data. Nevertheless, their research is still of great significance. They confirmed that GAG is the main factor that prevents drugs from entering the cartilage. Due to the loss of GAG in OA, even larger electrically neutral particles can penetrate OA cartilage to some extent. In another study, Bottini et al. evaluated the permeability of carbon nanotubes in healthy and OA mice (Sacchetti et al., Citation2014). PEG-SWCNT-650 was stranded on the cartilage surface after 1 day of injection, entered the cytoplasm or nucleus after 3 days, and particles were still visible in the cells after 14 days of administration. They suggested that there was no significant difference in drug transport between healthy and OA mice. However, it could be observed from the figure in this paper that the positive signal was strong in normal mice. Moreover, in vivo imaging of intra-articular retention signal intensity could be reflected in healthy mice for longer retention. We speculated that the accelerated clearance of DDS in articular cavity is due to cartilage ECM damage, loss of GAG, inflammation, and angiogenesis with OA state. Therefore, we should further study the difference of DDS permeability in healthy and OA cartilage, ultimately design permeability DDS suitable for different stages of OA, to give full play to the role of DDS.
Finally, most studies on permeability have used animal models, which poses a challenge in the translation of the data in human clinical settings. This is mainly due to the differences in cartilage composition and cartilage thickness between humans and animals. The thickness of cartilages in mice, rats, rabbits, and other common laboratory animals is less than 700 μm, while the thickness of human cartilage is about 1.5–2.0 mm (Bajpayee & Grodzinsky, Citation2017). Therefore, some OA treatments that work in animal models have failed in human clinical trials. Bajpayee et al. observed differences between healthy 12-month-old female rabbits and rats in transport dynamics and retention in the knee joint tissues. This showed that differences in cartilage thickness and animal size should be considered when evaluating cartilage delivery techniques. Consequently, the permeability of DDSs targeting chondrocytes should be evaluated using thicker tissues (such as bovine cartilage or horse cartilage). Bajpayee et al. found that the half-life of avidin has little correlation with the concentration of GAGs in rabbit cartilage but has a strong correlation with the square of cartilage thickness () (Bajpayee et al., Citation2015). This significant relationship indicates that we may be able to establish a standardized cartilage and drug half-life model that will provide some reference for application to human beings. Therefore, there is a need to explore ways of minimizing the impact of differences in cartilage properties on the experimental results.
Figure 10. The relationship of avidin half-life with GAG concentration or density. (A) In different tissues, the relation of GAGs concentration with Avidin half-lives. (B) The correlation of Avidin half-lives with GAGs concentration* tissue thickness square for different tissue types. Reprinted with permission from Bajpayee et al. (Citation2015).
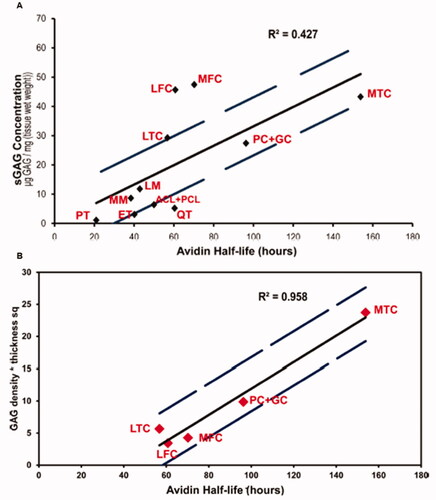
In conclusion, great progress has been made in the development of DDSs for the treatment of OA, with enhanced capacity for continuous drug delivery and to reduce osteoarthritis symptoms and delay disease progression. However, DDSs with effective penetrating capacity are required to develop chondrocyte-targeting drugs to treat the root cause of osteoarthritis. Consequently, as shown in , the application of articular cavity DDSs will improve the ability of DDSs to penetrate cartilage ECM, thereby delivering drugs through the sustained release of DDSs.
Disclosure statement
No potential conflict of interest was reported by the author(s).
Additional information
Funding
References
- Ai X, Duan Y, Zhang Q, et al. (2021). Cartilage-targeting ultrasmall lipid-polymer hybrid nanoparticles for the prevention of cartilage degradation. Bioeng Transl Med 6:e10187.
- Aini H, Itaka K, Fujisawa A, et al. (2016). Messenger RNA delivery of a cartilage-anabolic transcription factor as a disease-modifying strategy for osteoarthritis treatment. Sci Rep 6:18743.
- Andres-Guerrero V, Zong M, Ramsay E, et al. (2015). Novel biodegradable polyesteramide microspheres for controlled drug delivery in Ophthalmology. J Control Release 211:105–17.
- Ansboro S, Hayes JS, Barron V, et al. (2014). A chondromimetic microsphere for in situ spatially controlled chondrogenic differentiation of human mesenchymal stem cells. J Control Release 179:42–51.
- Aydin O, Korkusuz F, Korkusuz P, et al. (2015). In vitro and in vivo evaluation of doxycycline-chondroitin sulfate/PCLmicrospheres for intraarticular treatment of osteoarthritis. J Biomed Mater Res B Appl Biomater 103:1238–48.
- Bajpayee AG, De la Vega RE, Scheu M, et al. (2017). Sustained intra-cartilage delivery of low dose dexamethasone using a cationic carrier for treatment of post traumatic osteoarthritis. Eur Cell Mater 34:341–64.
- Bajpayee AG, Grodzinsky AJ. (2017). Cartilage-targeting drug delivery: can electrostatic interactions help? Nat Rev Rheumatol 13:183–93.
- Bajpayee AG, Quadir MA, Hammond PT, Grodzinsky AJ. (2016). Charge based intra-cartilage delivery of single dose dexamethasone using Avidin nano-carriers suppresses cytokine-induced catabolism long term. Osteoarthritis Cartilage 24:71–81.
- Bajpayee AG, Scheu M, Grodzinsky AJ, Porter RM. (2014). Electrostatic interactions enable rapid penetration, enhanced uptake and retention of intra-articular injected avidin in rat knee joints. J Orthop Res 32:1044–51.
- Bajpayee AG, Scheu M, Grodzinsky AJ, Porter RM. (2015). A rabbit model demonstrates the influence of cartilage thickness on intra-articular drug delivery and retention within cartilage. J Orthop Res 33:660–7.
- Bajpayee AG, Wong CR, Bawendi MG, et al. (2014). Avidin as a model for charge driven transport into cartilage and drug delivery for treating early stage post-traumatic osteoarthritis. Biomaterials 35:538–49.
- Bedingfield SK, Colazo JM, Di Francesco M, et al. (2021). Top-down fabricated microPlates for prolonged, intra-articular matrix metalloproteinase 13 siRNA nanocarrier delivery to reduce post-traumatic osteoarthritis. ACS Nano 15:14475–91.
- Bedingfield SK, Colazo JM, Yu F, et al. (2021). Amelioration of post-traumatic osteoarthritis via nanoparticle depots delivering small interfering RNA to damaged cartilage. Nat Biomed Eng 5:1069–83.
- Behrens F, Shepard N, Mitchell N. (1975). Alterations of rabbit articular cartilage by intra-articular injections of glucocorticoids. J Bone Joint Surg Am 57:70–6.
- Bonnet V, Gervaise C, Djedaini-Pilard F, et al. (2015). Cyclodextrin nanoassemblies: a promising tool for drug delivery. Drug Discov Today 20:1120–6.
- Bottini M, Bhattacharya K, Fadeel B, et al. (2016). Nanodrugs to target articular cartilage: an emerging platform for osteoarthritis therapy. Nanomedicine 12:255–68.
- Brown S, Kumar S, Sharma B. (2019). Intra-articular targeting of nanomaterials for the treatment of osteoarthritis. Acta Biomater 93:239–57.
- Brown S, Pistiner J, Adjei IM, Sharma B. (2019). Nanoparticle properties for delivery to cartilage: the implications of disease state, synovial fluid, and off-target uptake. Mol Pharm 16:469–79.
- Burr DB, Gallant MA. (2012). Bone remodelling in osteoarthritis. Nat Rev Rheumatol 8:665–73.
- Cai JY, Zhang L, Chen J, Chen SY. (2019). Kartogenin and its application in regenerative medicine. Curr Med Sci 39:16–20.
- Cai Y, Lopez-Ruiz E, Wengel J, et al. (2017). A hyaluronic acid-based hydrogel enabling CD44-mediated chondrocyte binding and gapmer oligonucleotide release for modulation of gene expression in osteoarthritis. J Control Release 253:153–9.
- Castro-Aguirre E, Iniguez-Franco F, Samsudin H, et al. (2016). Poly(lactic acid)-mass production, processing, industrial applications, and end of life. Adv Drug Deliv Rev 107:333–66.
- Chauhan AS. (2018). Dendrimers for drug delivery. Molecules 23:938.
- Chiang CS, Chen JY, Chiang MY, et al. (2018). Using the interplay of magnetic guidance and controlled TGF-β release from protein-based nanocapsules to stimulate chondrogenesis. Int J Nanomedicine 13:3177–88.
- Cullis PR, Hope MJ. (2017). Lipid nanoparticle systems for enabling gene therapies. Mol Ther 25:1467–75.
- Das D, Cho H, Kim N, et al. (2019). A terpolymeric hydrogel of hyaluronate-hydroxyethyl acrylate-gelatin methacryloyl with tunable properties as biomaterial. Carbohydr Polym 207:628–39.
- Dhanabalan KM, Gupta VK, Agarwal R. (2020). Rapamycin-PLGA microparticles prevent senescence, sustain cartilage matrix production under stress and exhibit prolonged retention in mouse joints. Biomater Sci 8:4308–21.
- Di Francesco M, Bedingfield SK, Di Francesco V, et al. (2021). Shape-defined microPlates for the sustained intra-articular release of dexamethasone in the management of overload-induced osteoarthritis. ACS Appl Mater Interfaces 13:31379–92.
- DiDomenico CD, Goodearl A, Yarilina A, Sun V, et al. (2017). The effect of antibody size and mechanical loading on solute diffusion through the articular surface of cartilage. J Biomech Eng 139:202.
- DiDomenico CD, Lintz M, Bonassar LJ. (2018). Molecular transport in articular cartilage – what have we learned from the past 50 years? Nat Rev Rheumatol 14:393–403.
- Ding D, Zhu Q. (2018). Recent advances of PLGA micro/nanoparticles for the delivery of biomacromolecular therapeutics. Mater Sci Eng C Mater Biol Appl 92:1041–60.
- Dwivedi P, Nayak V, Kowshik M. (2015). Role of gold nanoparticles as drug delivery vehicles for chondroitin sulfate in the treatment of osteoarthritis. Biotechnol Prog 31:1416–22.
- Fan W, Li J, Yuan L, et al. (2018). Intra-articular injection of kartogenin-conjugated polyurethane nanoparticles attenuates the progression of osteoarthritis. Drug Deliv 25:1004–12.
- Feng Q, Lin S, Zhang K, et al. (2017). Sulfated hyaluronic acid hydrogels with retarded degradation and enhanced growth factor retention promote hMSC chondrogenesis and articular cartilage integrity with reduced hypertrophy. Acta Biomater 53:329–42.
- Florine EM, Miller RE, Liebesny PH, et al. (2015). Delivering heparin-binding insulin-like growth factor 1 with self-assembling peptide hydrogels. Tissue Eng Part A 21:637–46.
- Fox LJ, Richardson RM, Briscoe WH. (2018). PAMAM dendrimer – cell membrane interactions. Adv Colloid Interface Sci 257:1–18.
- Fu Q, Kou L, Gong C, et al. (2012). Relationship between dissolution and bioavailability for nimodipine colloidal dispersions: the critical size in improving bioavailability. Int J Pharm 427:358–64.
- Garcia JP, Stein J, Cai Y, et al. (2019). Fibrin-hyaluronic acid hydrogel-based delivery of antisense oligonucleotides for ADAMTS5 inhibition in co-delivered and resident joint cells in osteoarthritis. J Control Release 294:247–58.
- Geiger B, Wang S, Padera R, et al. (2018). Cartilage-penetrating nanocarriers improve delivery and efficacy of growth factor treatment of osteoarthritis. Sci Transl Med 10:800.
- Goldring SR, Goldring MB. (2016). Changes in the osteochondral unit during osteoarthritis: structure, function and cartilage-bone crosstalk. Nat Rev Rheumatol 12:632–44.
- Goto N, Okazaki K, Akasaki Y, et al. (2017). Single intra-articular injection of fluvastatin-PLGA microspheres reduces cartilage degradation in rabbits with experimental osteoarthritis. J Orthop Res 35:2465–75.
- Guo K, Chu CC. (2009). Biodegradable and injectable paclitaxel-loaded poly(ester amide)s microspheres: fabrication and characterization. J Biomed Mater Res 89B:491–500.
- He T, Zhang C, Vedadghavami A, et al. (2020). Multi-arm Avidin nano-construct for intra-cartilage delivery of small molecule drugs. J Control Release 318:109–23.
- He Z, Wang B, Hu C, Zhao J. (2017). An overview of hydrogel-based intra-articular drug delivery for the treatment of osteoarthritis. Colloids Surf B Biointerfaces 154:33–9.
- Hu HY, Lim NH, Ding-Pfennigdorff D, et al. (2015). DOTAM derivatives as active cartilage-targeting drug carriers for the treatment of osteoarthritis. Bioconjug Chem 26:383–8.
- Hu Q, Chen Q, Yan X, et al. (2018). Chondrocyte affinity peptide modified PAMAM conjugate as a nanoplatform for targeting and retention in cartilage. Nanomedicine 13:749–67.
- Hu Q, Ding B, Yan X, et al. (2017). Polyethylene glycol modified PAMAM dendrimer delivery of kartogenin to induce chondrogenic differentiation of mesenchymal stem cells. Nanomedicine 13:2189–98.
- Huang ZW, Shi Y, Zhai YY, et al. (2021). Hyaluronic acid coated bilirubin nanoparticles attenuate ischemia reperfusion-induced acute kidney injury. J Control Release 334:275–89.
- Janssen M, Timur UT, Woike N, et al. (2016). Celecoxib-loaded PEA microspheres as an auto regulatory drug-delivery system after intra-articular injection. J Control Release 244:30–40.
- Jiang T, Kan HM, Rajpura K, et al. (2018). Development of targeted nanoscale drug delivery system for osteoarthritic cartilage tissue. J Nanosci Nanotechnol 18:2310–7.
- Johnson K, Zhu S, Tremblay MS, et al. (2012). A stem cell-based approach to cartilage repair. Science 336:717–21.
- Jones IA, Togashi R, Wilson ML, et al. (2019). Intra-articular treatment options for knee osteoarthritis. Nat Rev Rheumatol 15:77–90.
- Jung JH, Kim SE, Kim HJ, et al. (2020). A comparative pilot study of oral diacerein and locally treated diacerein-loaded nanoparticles in a model of osteoarthritis. Int J Pharm 581:119249.
- Kang MJ, Kim SR, Ho MJ, et al. (2015). Cationic PLGA/Eudragit RL nanoparticles for increasing retention time in synovial cavity after intra-articular injection in knee joint. Int J Nanomedicine 10:5263–71.
- Kang ML, Kim JE, Im GI. (2016). Thermoresponsive nanospheres with independent dual drug release profiles for the treatment of osteoarthritis. Acta Biomater 39:65–78.
- Kang ML, Ko JY, Kim JE, Im GI. (2014). Intra-articular delivery of kartogenin-conjugated chitosan nano/microparticles for cartilage regeneration. Biomaterials 35:9984–94.
- Karsdal MA, Michaelis M, Ladel C, et al. (2016). Disease-modifying treatments for osteoarthritis (DMOADs) of the knee and hip: lessons learned from failures and opportunities for the future. Osteoarthritis Cartilage 24:2013–21.
- Kim Y, Klutz AM, Jacobson KA. (2008). Systematic investigation of polyamidoamine dendrimers surface-modified with poly(ethylene glycol) for drug delivery applications: synthesis, characterization, and evaluation of cytotoxicity. Bioconjugate Chem 19:1660–72.
- Kim Y, Park EJ, Na DH. (2018). Recent progress in dendrimer-based nanomedicine development. Arch Pharm Res 41:571–82.
- Kimmerling KA, Furman BD, Mangiapani DS, Moverman MA, et al. (2015). Sustained intra-articular delivery of IL-1RA from a thermally-responsive elastin-like polypeptide as a therapy for post-traumatic arthritis. Eur Cell Mater 29:124–39.
- Kolasinski SL, Neogi T, Hochberg MC, et al. (2020). 2019 American College of rheumatology/arthritis foundation guideline for the management of osteoarthritis of the hand, hip, and knee. Arthritis Rheumatol 72:220–33.
- Kompel AJ, Roemer FW, Murakami AM, Diaz LE, et al. (2019). Intra-articular corticosteroid injections in the hip and knee: perhaps not as safe as we thought? Radiology 293:656–63.
- Kono K, Kojima C, Hayashi N, et al. (2008). Preparation and cytotoxic activity of poly(ethylene glycol)-modified poly(amidoamine) dendrimers bearing adriamycin. Biomaterials 29:1664–75.
- Kopac T. (2021). Protein corona, understanding the nanoparticle-protein interactions and future perspectives: a critical review. Int J Biol Macromol 169:290–301.
- Kou L, Bhutia YD, Yao Q, et al. (2018). Transporter-guided delivery of nanoparticles to improve drug permeation across cellular barriers and drug exposure to selective cell types. Front Pharmacol 9:27.
- Kou L, Jiang X, Huang H, et al. (2020). The role of transporters in cancer redox homeostasis and cross-talk with nanomedicines. Asian J Pharm Sci 15:145–57.
- Kou L, Sun R, Jiang X, et al. (2020). Tumor microenvironment-responsive, multistaged liposome induces apoptosis and ferroptosis by amplifying oxidative stress for enhanced cancer therapy. ACS Appl Mater Interfaces 12:30031–43.
- Kou L, Sun R, Xiao S, et al. (2020). OCTN2-targeted nanoparticles for oral delivery of paclitaxel: differential impact of the polyethylene glycol linker size on drug delivery in vitro, in situ, and in vivo. Drug Deliv 27:170–9.
- Kou L, Xiao S, Sun R, et al. (2019). Biomaterial-engineered intra-articular drug delivery systems for osteoarthritis therapy. Drug Deliv 26:870–85.
- Kou L, Yao Q, Zhang H, et al. (2020). Transporter-targeted nano-sized vehicles for enhanced and site-specific drug delivery. Cancers 12:2837.
- Krishnamurthy A, Lang AE, Pangarkar S, et al. (2021). Synopsis of the 2020 US Department of Veterans Affairs/US Department of Defense clinical practice guideline: the non-surgical management of hip and knee osteoarthritis. Mayo Clin Proc 96:2435–47.
- Krych AJ, Saris DBF, Stuart MJ, Hacken B. (2020). Cartilage injury in the knee: assessment and treatment options. J Am Acad Orthop Surg 28:914–22.
- Kumar A, Bendele AM, Blanks RC, Bodick N. (2015). Sustained efficacy of a single intra-articular dose of FX006 in a rat model of repeated localized knee arthritis. Osteoarthritis Cartilage 23:151–60.
- Leddy HA, Guilak F. (2003). Site-specific molecular diffusion in articular cartilage measured using fluorescence recovery after photobleaching. Ann Biomed Eng 31:753–60.
- Leddy HA, Haider MA, Guilak F. (2006). Diffusional anisotropy in collagenous tissues: fluorescence imaging of continuous point photobleaching. Biophys J 91:311–6.
- Li J, Gorski DJ, Anemaet W, et al. (2012). Hyaluronan injection in murine osteoarthritis prevents TGFbeta 1-induced synovial neovascularization and fibrosis and maintains articular cartilage integrity by a CD44-dependent mechanism. Arthritis Res Ther 14:R151.
- Li P, Li H, Shu X, et al. (2020). Intra-articular delivery of flurbiprofen sustained release thermogel: improved therapeutic outcome of collagenase II-induced rat knee osteoarthritis. Drug Deliv 27:1034–43.
- Liang Y, Kiick KL. (2014). Heparin-functionalized polymeric biomaterials in tissue engineering and drug delivery applications. Acta Biomater 10:1588–600.
- Lin AC, Seeto BL, Bartoszko JM, et al. (2009). Modulating hedgehog signaling can attenuate the severity of osteoarthritis. Nat Med 15:1421–5.
- Lin J, Zhang W, Jones A, Doherty M. (2004). Efficacy of topical non-steroidal anti-inflammatory drugs in the treatment of osteoarthritis: meta-analysis of randomised controlled trials. BMJ 329:324.
- Liu H, Chen J, Qiao S, Zhang W. (2021). Carbon-based nanomaterials for bone and cartilage regeneration: a review. ACS Biomater Sci Eng 7:4718–35.
- Liu J, Jiang T, Li C, et al. (2019). Bioconjugated carbon dots for delivery of siTnfα to enhance chondrogenesis of mesenchymal stem cells by suppression of inflammation. Stem Cells Transl Med 8:724–36.
- Lories RJ, Luyten FP. (2011). The bone-cartilage unit in osteoarthritis. Nat Rev Rheumatol 7:43–9.
- Lorscheider M, Tsapis N, Ur-Rehman M, et al. (2019). Dexamethasone palmitate nanoparticles: an efficient treatment for rheumatoid arthritis. J Control Release 296:179–89.
- Losina E, Paltiel AD, Weinstein AM, et al. (2015). Lifetime medical costs of knee osteoarthritis management in the United States: impact of extending indications for total knee arthroplasty. Arthritis Care Res 67:203–15.
- Lundqvist M, Stigler J, Elia G, et al. (2008). Nanoparticle size and surface properties determine the protein corona with possible implications for biological impacts. Proc Natl Acad Sci USA 105:14265–70.
- Maheu E, Bannuru RR, Herrero-Beaumont G, et al. (2019). Why we should definitely include intra-articular hyaluronic acid as a therapeutic option in the management of knee osteoarthritis: results of an extensive critical literature review. Semin Arthritis Rheum 48:563–72.
- Mao H, Kawazoe N, Chen G. (2013). Uptake and intracellular distribution of collagen-functionalized single-walled carbon nanotubes. Biomaterials 34:2472–9.
- Masters KS, Shah DN, Leinwand LA, Anseth KS. (2005). Crosslinked hyaluronan scaffolds as a biologically active carrier for valvular interstitial cells. Biomaterials 26:2517–25.
- Mathiessen A, Conaghan P. (2017). Synovitis in osteoarthritis: current understanding with therapeutic implications. Arthritis Res Ther 19:18.
- Matsuzaki T, Matsushita T, Tabata Y, et al. (2014). Intra-articular administration of gelatin hydrogels incorporating rapamycin-micelles reduces the development of experimental osteoarthritis in a murine model. Biomaterials 35:9904–11.
- Maudens P, Meyer S, Seemayer CA, et al. (2018). Self-assembled thermoresponsive nanostructures of hyaluronic acid conjugates for osteoarthritis therapy. Nanoscale 10:1845–54.
- Maudens P, Seemayer CA, Pfefferle F, et al. (2018). Nanocrystals of a potent p38 MAPK inhibitor embedded in microparticles: therapeutic effects in inflammatory and mechanistic murine models of osteoarthritis. J Control Release 276:102–12.
- McAlindon TE, LaValley MP, Harvey WF, et al. (2017). Effect of intra-articular triamcinolone vs saline on knee cartilage volume and pain in patients with knee osteoarthritis: a randomized clinical trial. JAMA 317:1967–75.
- McGarry JG, Daruwalla ZJ. (2011). The efficacy, accuracy and complications of corticosteroid injections of the knee joint. Knee Surg Sports Traumatol Arthrosc 19:1649–54.
- McMasters J, Poh S, Lin JB, Panitch A. (2017). Delivery of anti-inflammatory peptides from hollow PEGylated poly(NIPAM) nanoparticles reduces inflammation in an ex vivo osteoarthritis model. J Control Release 258:161–70.
- Meng W, Rey-Rico A, Claudel M, et al. (2020). rAAV-mediated overexpression of SOX9 and TGF-beta via carbon dot-guided vector delivery enhances the biological activities in human bone marrow-derived mesenchymal stromal cells. Nanomaterials 10:855.
- Migliorini F, Driessen A, Quack V, et al. (2021). Comparison between intra-articular infiltrations of placebo, steroids, hyaluronic and PRP for knee osteoarthritis: a Bayesian network meta-analysis. Arch Orthop Trauma Surg 141:1473–90.
- Mok SW, Fu SC, Cheuk YC, et al. (2020). Intra-articular delivery of quercetin using thermosensitive hydrogel attenuate cartilage degradation in an osteoarthritis rat model. Cartilage 11:490–9.
- Muxika A, Etxabide A, Uranga J, et al. (2017). Chitosan as a bioactive polymer: processing, properties and applications. Int J Biol Macromol 105:1358–68.
- Nakazawa F, Matsuno H, Yudoh K, et al. (2002). Corticosteroid treatment induces chondrocyte apoptosis in an experimental arthritis model and in chondrocyte cultures. Clin Exp Rheumatol 20:773–81.
- Ndeupen S, Qin Z, Jacobsen S, et al. (2021). The mRNA-LNP platform’s lipid nanoparticle component used in preclinical vaccine studies is highly inflammatory. iScience 24:103479.
- Niazvand F, Khorsandi L, Abbaspour M, et al. (2017). Curcumin-loaded poly lactic-co-glycolic acid nanoparticles effects on mono-iodoacetate -induced osteoarthritis in rats. Vet Res Forum 8:155–61.
- Ouyang Z, Tan T, Liu C, et al. (2019). Targeted delivery of hesperetin to cartilage attenuates osteoarthritis by bimodal imaging with Gd2(CO3)3@PDA nanoparticles via TLR-2/NF-κB/Akt signaling. Biomaterials 205:50–63.
- Pawar VA, Manjappa AS, Murumkar PR, et al. (2018). Drug-fortified liposomes as carriers for sustained release of NSAIDs: the concept and its validation in the animal model for the treatment of arthritis. Eur J Pharm Sci 125:11–22.
- Pelletier J, DiBattista J, Roughley P, et al. (1993). Cytokines and inflammation in cartilage degradation. Rheum Dis Clin North Am 19:545–68.
- Pereira D, Ramos E, Branco J. (2015). Osteoarthritis. Acta Med Port 28:99–106.
- Perni S, Prokopovich P. (2017). Poly-beta-amino-esters nano-vehicles based drug delivery system for cartilage. Nanomedicine 13:539–48.
- Peters T, Kim SW, Castro V, et al. (2017). Evaluation of polyesteramide (PEA) and polyester (PLGA) microspheres as intravitreal drug delivery systems in albino rats. Biomaterials 124:157–68.
- Petit A, Sandker M, Muller B, et al. (2014). Release behavior and intra-articular biocompatibility of celecoxib-loaded acetyl-capped PCLA-PEG-PCLA thermogels. Biomaterials 35:7919–28.
- Pi Y, Zhang X, Shi J, et al. (2011). Targeted delivery of non-viral vectors to cartilage in vivo using a chondrocyte-homing peptide identified by phage display. Biomaterials 32:6324–32.
- Réeff J, Oprenyeszk F, Franck T, et al. (2015). Development and evaluation in vitro and in vivo of injectable hydrolipidic gels with sustained-release properties for the management of articular pathologies such as osteoarthritis. Int J Pharm 490:74–84.
- Rivera-Delgado E, Djuhadi A, Danda C, et al. (2018). Injectable liquid polymers extend the delivery of corticosteroids for the treatment of osteoarthritis. J Control Release 284:112–21.
- Rothenfluh DA, Bermudez H, O’Neil CP, Hubbell JA. (2008). Biofunctional polymer nanoparticles for intra-articular targeting and retention in cartilage. Nat Mater 7:248–54.
- Rudnik-Jansen I, Colen S, Berard J, et al. (2017). Prolonged inhibition of inflammation in osteoarthritis by triamcinolone acetonide released from a polyester amide microsphere platform. J Control Release 253:64–72.
- Ryan SM, McMorrow J, Umerska A, et al. (2013). An intra-articular salmon calcitonin-based nanocomplex reduces experimental inflammatory arthritis. J Control Release 167:120–9.
- Sacchetti C, Liu-Bryan R, Magrini A, et al. (2014). Polyethylene-glycol-modified single-walled carbon nanotubes for intra-articular delivery to chondrocytes. ACS Nano 8:12280–91.
- Schneider T, Welker P, Haag R, et al. (2015). Effects of dendritic polyglycerol sulfate on articular chondrocytes. Inflamm Res 64:917–28.
- Schneider T, Welker P, Licha K, et al. (2015). Influence of dendritic polyglycerol sulfates on knee osteoarthritis: an experimental study in the rat osteoarthritis model. BMC Musculoskelet Disord 16:387.
- Shah NJ, Geiger BC, Quadir MA, et al. (2016). Synthetic nanoscale electrostatic particles as growth factor carriers for cartilage repair. Bioeng Transl Med 1:347–56.
- Shamekhi MA, Mirzadeh H, Mahdavi H, et al. (2019). Graphene oxide containing chitosan scaffolds for cartilage tissue engineering. Int J Biol Macromol 127:396–405.
- Shen H, Lin H, Sun AX, et al. (2018). Chondroinductive factor-free chondrogenic differentiation of human mesenchymal stem cells in graphene oxide-incorporated hydrogels. J Mater Chem B 6:908–17.
- Sladek S, Kearney C, Crean D, et al. (2018). Intra-articular delivery of a nanocomplex comprising salmon calcitonin, hyaluronic acid, and chitosan using an equine model of joint inflammation. Drug Deliv Transl Res 8:1421–35.
- Tellegen A, Beukers M, Rudnik-Jansen I, et al. (2021). Intra-articular slow-release triamcinolone acetonide from polyesteramide microspheres as a treatment for osteoarthritis. Pharmaceutics 13:372.
- Tellier LE, Trevino EA, Brimeyer AL, et al. (2018). Intra-articular TSG-6 delivery from heparin-based microparticles reduces cartilage damage in a rat model of osteoarthritis. Biomater Sci 6:1159–67.
- Tomalia DA. (2012). Interview: an architectural journey: from trees, dendrons/dendrimers to nanomedicine. Interview by Hannah Stanwix. Nanomedicine 7:953–6.
- van der Meijden OA, Gaskill TR, Millett PJ. (2012). Glenohumeral joint preservation: a review of management options for young, active patients with osteoarthritis. Adv Orthop 2012:160923.
- Vedadghavami A, Wagner EK, Mehta S, et al. (2019). Cartilage penetrating cationic peptide carriers for applications in drug delivery to avascular negatively charged tissues. Acta Biomater 93:258–69.
- Wang G, Zhao L, Jiang Q, et al. (2020). Intestinal OCTN2- and MCT1-targeted drug delivery to improve oral bioavailability. Asian J Pharm Sci 15:158–73.
- Wang J, Wang X, Cao Y, et al. (2018). Therapeutic potential of hyaluronic acid/chitosan nanoparticles for the delivery of curcuminoid in knee osteoarthritis and an in vitro evaluation in chondrocytes. Int J Mol Med 42:2604–14.
- Wang S, Wei X, Sun X, et al. (2018). A novel therapeutic strategy for cartilage diseases based on lipid nanoparticle-RNAi delivery system. Int J Nanomedicine 13:617–31.
- Xia C, Chen P, Mei S, et al. (2017). Photo-crosslinked HAMA hydrogel with cordycepin encapsulated chitosan microspheres for osteoarthritis treatment. Oncotarget 8:2835–49.
- Xia YJ, Xia H, Chen L, et al. (2018). Efficient delivery of recombinant human bone morphogenetic protein (rhBMP-2) with dextran sulfate-chitosan microspheres. Exp Ther Med 15:3265–72.
- Xue S, Zhou X, Sang W, et al. (2021). Cartilage-targeting peptide-modified dual-drug delivery nanoplatform with NIR laser response for osteoarthritis therapy. Bioact Mater 6:2372–89.
- Yudoh K, Shishido K, Murayama H, et al. (2007). Water-soluble C60 fullerene prevents degeneration of articular cartilage in osteoarthritis via down-regulation of chondrocyte catabolic activity and inhibition of cartilage degeneration during disease development. Arthritis Rheum 56:3307–18.
- Zeng WN, Zhang Y, Wang D, et al. (2021). Intra-articular injection of kartogenin-enhanced bone marrow-derived mesenchymal stem cells in the treatment of knee osteoarthritis in a rat model. Am J Sports Med 49:2795–809.
- Zhang HF, Wang CG, Li H, et al. (2018). Intra-articular platelet-rich plasma versus hyaluronic acid in the treatment of knee osteoarthritis: a meta-analysis. Drug Des Devel Ther 12:445–53.
- Zhang L, Sui C, Yang W, Luo Q. (2020). Amino acid transporters: emerging roles in drug delivery for tumor-targeting therapy. Asian J Pharm Sci 15:192–206.
- Zhang S, Bai C, Zheng D, et al. (2016). Identification and characterization of pig adipose-derived progenitor cells. Can J Vet Res 80:309–17.
- Zhang X, Shi Y, Zhang Z, et al. (2018). Intra-articular delivery of tetramethylpyrazine microspheres with enhanced articular cavity retention for treating osteoarthritis. Asian J Pharm Sci 13:229–38.
- Zheng L, Zhang Z, Sheng P, Mobasheri A. (2021). The role of metabolism in chondrocyte dysfunction and the progression of osteoarthritis. Ageing Res Rev 66:101249.
- Zhong G, Yang X, Jiang X, et al. (2019). Dopamine-melanin nanoparticles scavenge reactive oxygen and nitrogen species and activate autophagy for osteoarthritis therapy. Nanoscale 11:11605–16.
- Zhou J, Chen Q, Lanske B, et al. (2014). Disrupting the Indian hedgehog signaling pathway in vivo attenuates surgically induced osteoarthritis progression in Col2a1-CreERT2; Ihhfl/fl mice. Arthritis Res Ther 16:R11.
- Zhou Y, Liu SQ, Peng H, et al. (2015). In vivo anti-apoptosis activity of novel berberine-loaded chitosan nanoparticles effectively ameliorates osteoarthritis. Int Immunopharmacol 28:34–43.
- Zhu M, Zhu Y, Zhang L, Shi J. (2013). Preparation of chitosan/mesoporous silica nanoparticle composite hydrogels for sustained co-delivery of biomacromolecules and small chemical drugs. Sci Technol Adv Mater 14:045005.
- Zhu S, Lu P, Liu H, et al. (2015). Inhibition of Rac1 activity by controlled release of NSC23766 from chitosan microspheres effectively ameliorates osteoarthritis development in vivo. Ann Rheum Dis 74:285–93.