Abstract
Vaccinations, especially DNA vaccines that promote host immunity, are the most effective interventions for tuberculosis (TB) control. However, the vaccine delivery system exhibits a significant impact on the protective effects of the vaccine. Recently, effective nanomaterial-based delivery systems (including nanoparticles, nanogold, nanoliposomes, virus-like particles, and virus carriers) have been developed for DNA vaccines to control TB. This review highlights the historical development of various nanomaterial-based delivery systems for TB DNA vaccines, along with the emerging technologies. Nanomaterial-based vaccine delivery systems could enhance the efficacy of TB vaccination; therefore, this summary could guide nanomaterial selection for optimal and safe vaccine delivery, facilitating the design and development of highly effective TB vaccines.
1. Introduction
Tuberculosis (TB), causing 1.5 million deaths in 2020 (Ghebreyesus, Citation2022, Zaman, Citation2010), is a significant global threat to human health. In this year, an estimated 10 million people worldwide have been infected with TB (Ghebreyesus, Citation2022), with 1 million new patients (Ghebreyesus) reported in 2021 (according to the World Health Organization), and 842,000 (World Health Organization, 2020) new cases in China in 2020. On an average, 49% TB patients spend more than 20% (in the range of 19–83%) of their annual household income on TB treatment (Viney et al., Citation2021),which is a challenging process due to numerous reasons (). It involves the circumvention of phagocytic fusion and its destruction (I), a neutralization of the acidic environment (II), an inhibition of envelope formation in apoptosis (III), the suppression of plasma-membrane repair and immune-cell activation (IV–V), and the restriction of pro-inflammatory responses (VI) (Sampath et al., Citation2021). Although a combination of first-line (isoniazid, rifampicin, ethambutol, and streptomycin) and second-line (amikacin, kanamycin, and capreomycin) drugs is useful for TB treatment, their utility is limited by the prevalence of multidrug-resistant TB (MDR-TB) (Marks et al., Citation2014), extensively drug-resistant TB (XDR-TB) (Mullerpattan et al., Citation2019), and HIV superinfection.
Figure 1. TB pathogenic mechanism.
Note: M. tuberculosis escapes the influence of host immune defenses by the (I) circumvention of phagocytic fusion and phagocytic destruction, (II) neutralization of the acidic environment, (III) inhibition of apoptotic-envelope formation, and (IV) suppression of plasma-membrane repair, causing the spread of infection after macrophage necrosis, (V) suppressing the activation of the immune cells and (VI) restricting the pro-inflammatory responses.
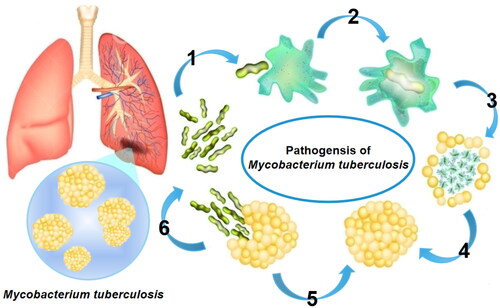
Vaccination that promotes host immunity is the most effective intervention for TB treatment (Sia & Rengarajan, Citation2019); the Bacillus Calmette–Guerin (BCG) vaccine is approved worldwide for the prevention of TB meningitis (Puvacic et al., Citation2004) and miliary TB (Trunz et al., 2006). Currently, more than 90% TB vaccines are manufactured using five variants, namely, the 1173P2 (Pasteur), 1331 (Danish), 1077 (Glaxo), 172 (Japanese), and D2PB302 (China) strains (Li et al., Citation2020). Although TB vaccination is effective for infants (Schrager et al., Citation2020), its protective efficacy for adolescents and adults is low (Lowrie et al., Citation1994). Additionally, the effectiveness of vaccination varies greatly in different countries owing to significant differences in immune capacities (Abubakar et al., Citation2013). However, administering the BCG vaccine at an age of 3–5 years provides protection against active TB for the next 20 years (Mangtani et al., Citation2018). Furthermore, the BCG vaccine exhibits significant protective efficacy against persistent new M. tuberculosis infections, as indicated by recent studies (Nemes et al. 2018). According to data from the United States National Library of Medicine (http://clinicaltrials.gov), 149 studies on TB have been conducted in different phases of clinical trials (105 have been completed and 15 are in recruitment). However, these vaccines exhibit a poor ability to generate humoral and cell-mediated immunity (Ojha et al., 2020), necessitating the development of novel vaccines and delivery systems for TB.
DNA vaccines, with facile preservation, transport, manufacture, good stability, and efficient immune responses, are a useful strategy for the treatment and prevention of MDR-TB (Kutzler & Weiner, Citation2008). Currently, prophylactic and therapeutic DNA-vaccine vectors for delivery include hemagglutinating virus of Japan (HVJ)-liposomes and their envelopes, adenoviral or lentiviral vectors, and adeno-associated viral vectors (Okada et al. 2007, Yoshida et al., Citation2006); the DNA-vaccine antigens are HSP65 (Goncalves et al., Citation2007), MPT64 (Kamath et al., Citation1999), Ag85A (Tanghe et al., Citation2001), ESAT6 (Wang et al., Citation2004), Mtb10, and Mtb41(Skeiky et al., Citation2000). DNA vaccines recombine the foreign gene encoding a specific antigen protein into a eukaryotic expression element to form recombinant plasmid DNA (Liang et al., Citation2008). Subsequently, this plasmid is directly transformed into living cells in vivo, its dominant gene is released and enters mRNA in the nucleus via the nuclear pore complex, which is translated into a protein via ribosomes and transfer RNA (tRNA). After being delivered by the APC to drainage lymph nodes, it induces cellular and humoral immune responses (Tejeda-Mansir et al., Citation2019). The greatest drawback of DNA vaccines is their lack of immunogenicity in large mammals because of poor delivery efficiency (Simmons et al., Citation2018). Therefore, to enhance the immune responses elicited by TB DNA vaccines, it is vital to improve vaccine delivery to the nucleus and optimize the antigen combinations (). This stimulates the host immune system to express internalized plasmid DNA via somatic cells, which is subsequently presented to the CD8+ T cells via class I MHC complexes (Sefidi-Heris et al., Citation2020). Hence, the design and development of safe and efficient delivery systems has attracted immense attention in the field of DNA-vaccine development.
Figure 2. TB DNA-vaccine action mechanisms.
Note: TB DNA vaccines induce immunogenicity and immune response in the host by improving the delivery process of the vaccine to the nucleus and optimizing the precise combinations of antigens.
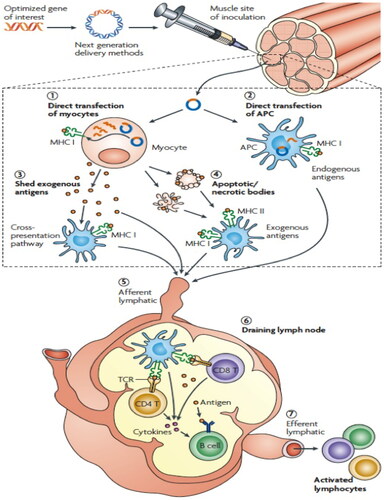
Nanomaterials, with good biocompatibility and biodegradability, easy modification and processing, and surface properties with a controllable mucosal absorption rate promote the entry of functional molecules into cells and protect against degradation, facilitating efficient drug delivery (Jiao et al., Citation2018). Various nanomaterials, including nanoparticles, liposomes, virus-like particles, and self-assembled proteins, have been developed for TB DNA-vaccine delivery () (Ho et al., Citation2021); recent reports indicate that nanomaterial-based delivery systems cause high immune responses (Wang et al., Citation2015). Thus, a systematic review of the progress in nanomaterial use for TB DNA-vaccine delivery could facilitate the development of safe and effective TB vaccines.
2. Nanomaterials in TB DNA-vaccine delivery
2.1. PLGA polymer nanoparticles in vaccine delivery
Polymer nanoparticles can be divided into two categories, namely, synthetic and natural (such as, poly (d, l-lactic-co-glycolic acid) (PLGA)) (Chan et al., Citation2010); these materials are widely used as they are easy to obtain and exhibit low production costs, good stability, and high physicochemical versatility. Additionally, PLGA exhibits immunological characteristics, including a receptor-recognition ability via cytokine stimulation and APCs, that protect vaccines from enzyme attack and provide more immune-safety than live bacterial carriers (Saxena et al., Citation2013, Thomas et al., Citation2019). Furthermore, PLGA can be used to independently design nanostructures with adjustable sizes and different surface properties (Franck et al., Citation2021). Thus, PLGA polymer nanoparticles, exhibiting good biocompatibility, favorable cellular interactions, and biodegradability, are widely used in the design and development of TB DNA vaccines (Mikusova & Mikus, Citation2021). Plasmid DNA and other molecules (such as, trehalose dimycolate and dimethyl dioctyldecyl ammonium bromide) have been incorporated into PLGA microspheres with up to 60% encapsulation efficiency (Khademi et al., Citation2018). As an efficient DNA-vaccine delivery system, PLGA nanoparticles prevent DNA degradation, facilitate pathogen stimulation, and enhance the internalization of APCs (Newman et al., Citation2002, Jiang et al., Citation2005). By encapsulating, capturing, and delivering plasmid DNA, PLGA nanoparticles deliver the vaccine materials to cells and maintain antigen release at a slow rate due to gradual biodegradation, stimulating the host to produce dual and long-acting cellular and humoral immune responses (Dykman & Khlebtsov, Citation2017) (). Furthermore, PLGA exhibits potential to be used as a plasmid-DNA transfection mediator targeting phagocytes, such as macrophages, and prevents nuclease biodegradation (Wang et al., Citation1999). Numerous vaccines, such as, the 2020-Mtb72F-TB10.4/CpG-PLGA vaccine (Dalirfardouei et al., Citation2020) and 2006-hsp65-DNA-TDM-PLGA vaccine (Coelho et al., Citation2006), utilize PLGA as the delivery system. In the development of TB DNA vaccines, the aforementioned immunostimulants are combined with plasmids loaded with TB-dominant antigen genes and subsequently encapsulated in the biopolymer material PLGA. Novel TB vaccines encapsulating plasmid DNA and immunostimulants using PLGA nanoparticles induce higher levels of interferon gamma (IFN)-γ and immunoglobulin (Ig) G2a subtype antibodies in mice via injection compared to naked plasmid-DNA vaccines (Lima et al., Citation2003), indicating a strong Th1 polarization-directed response (Wedlock et al., Citation2002). Additionally, after a challenge with M. tuberculosis H37Rv, a single dose of TB DNA nano-vaccine significantly decreased the bacterial colony-forming units in the lungs of mice and improved the granulomatous reactions of the lungs. Thus, M. tuberculosis is efficiently eradicated, without inhibiting its growth, on reducing the plasmid DNA by 10-fold. Additionally (Khademi et al., Citation2018), polymer nanoparticles with PLGA enhance the mucosal immune response to TB DNA vaccines and are suitable for the administration of non-parenteral and parenteral TB DNA vaccines. Therefore, non-biodegradable synthetic polymer materials and natural polymers cause significantly higher humoral and cellular immune responses than naked TB plasmid DNA (Rancan et al., Citation2014).
2.2. Chitosan (CS) and its derivative nanoparticles in vaccine delivery
The core scaffold of the biopolymer of chitins and its derivative nanoparticles can be modified with functional molecular adjuvants or copolymers, such as, dimycolates (TDM) and N, N, N-trimethyl chitosan (TMC), to enhance the plasmid transfection and antigen expression of TB DNA vaccines. TDM, located in the outer layer of the M. tuberculosis cell wall, acts as an immunomodulator with adjuvant effects; in combination with recombinant human hepatitis B virus vaccines, it produces cellular immune responses against the antigen and increased antibody levels (Koike et al., Citation1998). TMC is a cationic CS derivative that can load plasmid DNA via electrostatic interactions (Thanou et al., Citation1999; Amidi et al., Citation2006). CS nanoparticles have been used to formulate the existing BCG vaccine and other multi-epitope (T-cell epitopes) and pPES (multi-gene family protein) DNA vaccines. Several unique characteristics, such as, good bio-adhesion, effective antigen transport via instantaneous opening of tight junctions in cells, good biocompatibility, and low toxicity, make this nanoparticle delivery system particularly important for nasal DNA-vaccine delivery (Csaba et al., Citation2009).
2.3. Polyhydroxy biopolyester nanoparticles in vaccine delivery
Polyhydroxy biopolyester nanoparticles include poly (3-hydroxybutyrate) (PHB) and polyhydroxyalkanoates (PHA); PHB is commonly extracted and purified from E. coli and Lactococcus lactis. Antigens carried on specific nanoparticles are preferentially recognized and presented, enhancing the ability of cells to respond to immunogens (Khader et al., Citation2007). TB DNA vaccines containing PHB nanoparticles with ESAT-6 or Ag85A (the dominant antigens of M. tuberculosis) on the surface of biological beads induce high levels of cytokines interleukin (IL)-2, IFN-γ, tumor necrosis factor (TNF)-α, IL-17A, and IL-6 on intramuscular injection. According to Parlane et al., these bovine-TB vaccines cause a high T-cell immune response, with both CD4+ and CD8+ involved in the induction of IFN-γ release (Parlane et al., Citation2014). In TB DNA vaccines, PHA nanoparticles have been produced using bacteria (via bioengineering) as intracellular contents when carbon sources are abundant (Grage et al., Citation2009).
2.4. Fe3O4-Glu-polyethylenimine nanoparticles in vaccine delivery
Fe3O4-Glu-polyethylenimine nanoparticles encapsulated in a DNA vaccine (Ag85A-ESAT-6-IL-21) have been used as prophylactic vaccines in mouse models of M. tuberculosis infection (Yu et al., Citation2012). These nanoparticle vaccines induce a stronger immune response than naked DNA, overcome the inability of plasmid DNA to easily cross the cell membrane, stimulate IFN-γ production, and significantly reduce the bacterial burden after challenge in vaccinated mice (Pereira et al., Citation2014, Zhao et al., Citation2017). This vaccine exhibits a strong protective efficacy against M. tuberculosis, possibly due to the encapsulation of plasmid DNA in the Fe3O4-Glu-polyethylenimin nanoparticles. After intramuscular injection, a reservoir is formed at the injection site, initiating an immune response and gradually releasing the antigen, thereby establishing a long-term response. Both materials show strong protection against M. tuberculosis and should be studied in depth (Khan et al., Citation2007, Zaman et al., Citation2011).
2.5. CS/DNA-complex nanoparticles in vaccine delivery
A CS/DNA-complex nanoparticle system containing three ESAT-6 T cell epitopes (ESAT-6/3e) and FMS-like tyrosine kinase 3 ligand (FL) genes (termed ESAT-6/3e-FL) enveloped with CS nanoparticles has been reported to improve mucosal delivery and immunogenicity (Feng et al., Citation2013). This CS/DNA vaccine significantly improves the secretory IgA mucosal response level (Wu et al., Citation2017). Additionally, the CS/DNA nanoparticles exhibit a high cellular uptake and can be used to deliver TB DNA vaccines. Compared with traditional systems, the new design enables extracellular-matrix nucleic acids to be transferred more efficiently and remain longer on the nasal mucosal surface. Thus, the nanomaterial induces significantly higher levels of secretory IgA and a longer duration of mucosal immunity than naked DNA vaccines in animal studies; additionally, the vaccine exhibits minimal cytotoxicity (Wu et al., Citation2017). According to recent research, CS/DNA complex nanoparticles are safe and effective DNA-vaccine delivery systems (Fries et al., Citation2021). These nanoparticles have been developed as a potential platform for DNA packaging, to facilitate the crossing of DNA through different physiological barriers to elicit effective mucosal immunity.
2.6. Gold nanoparticles in vaccine delivery
The low transfection rate and necessity for repeated vaccinations are the main disadvantages of plasmid-DNA vaccines. According to observations made in 1992, the delivery of plasmid DNA coated with gold nanoparticles, administered in the epidermis using a gene gun loaded with pressurized helium, enhances the expression of foreign proteins and produces antibodies. Subsequently, it has been confirmed that the bombardment of the epidermis with gene-gun-assisted DNA delivered via gold nanoparticles preferentially triggers Th2-type cellular immune responses (IgG1 and IgE isotope antibodies) and produces Th2-characteristic cytokines (such as IL-4) (Feltquate et al., Citation1997, Tighe et al., Citation1998). However, the failure of vaccination programs indicates that the expression and transformation of exogenous genes in the host cells requires improvement. The 2007-Ag85A-EP + GM-CSF (Zhang et al., Citation2007), 2015-Ag85B-IL-33-EP (Villarreal et al., Citation2015), and 2018-EP vaccines (Tang et al., Citation2018) confirm the efficacy of AuNPs for DNA vaccination by injection via T cell activation and protection. Future studies should focus on the delivery of different-sized AuNPs loaded with plasmid DNA using various types of materials to develop new TB-protection strategies.
2.7. Liposome nanomaterials in vaccine delivery
Liposomes, small vesicles formed by hydrophobic and hydrophilic interactions, have been utilized for the delivery of TB vaccines (Pinheiro et al., Citation2011). Liposome-nanomaterial-based delivery effectively prevents DNA degradation by endonucleases outside the cell via interactions with the cell membrane, improving the efficiency of plasmid-DNA transfection presentation compared to naked plasmid-DNA vaccines (Schwendener et al., Citation2010). The surface charge and particle size of liposomes significantly influence their vaccine delivery (Song et al., Citation2014), possibly due to their physicochemical (size and charge) and immunogenic (combined with adjuvants and targeted ligands) abilities to attract, interact with, and activate APCs (such as, dendritic cells (DCs), macrophages, and B cells). The positively charged surfaces of cationic liposomes efficiently interact with the negatively charged surfaces of DCs, promoting the transmission and uptake of antigens. DCs are of great importance because they are one of the main inducers of T cell-mediated immune response and mediate antigen presentation to T cell receptors via the MHC. Modified liposomes with appropriate targeting ligands stimulate and activate cells through a pattern-recognition receptor, causing the maturation of APCs, and the processing and presentation of antigens (Chatzikleanthous et al., Citation2021) (). DNA contains phosphate groups; thus, it firmly binds to plasmid DNA through electrostatic interactions, and is more widely used than the other two forms of liposome encapsulation and transfection. A liposome vaccine composed of a newly developed liposome of dimethyl dioctadecyl ammonium (DDA) and pattern recognition receptor agonists (mono-phosphoryl lipid A [MPLA] and trehalose 6,6′-dibenzoate [TDB]) has been reported. This vaccine significantly reduces the ζ potential compared to naked plasmid-DNA delivery and exhibits a higher storage stability with slower and more sustained antigen release. As a Toll 4-like receptor agonist, MPLA elicits a strong humoral immune response and reduces the surface charge of DMT liposomes through electrostatic interactions, increasing the stability of the DNA vaccine. Furthermore, DDA/MPLA liposomes induce a stronger IFN-γ and IL-17 response than DDA alone (Agger et al., Citation2008). DDA-MPLA-TDB liposomes have been used for the design of TB DNA vaccines and the construction of a plasmid pCMFO that secretes the fusion of four multistage antigens (Rv0577, Rv3044, Rv2875, and Rv2073c). This vaccine elicits a more significant response level of IL-2, induces a Th1-biased response, and provides enhanced and more durable protection against M. tuberculosis infection than pCMFO or pCMFO/DDA. Additionally, liposomes enable the simultaneous slow release and deposition of plasmid DNA and the two receptor agonists TDB and MPLA at the injection site after immunization, to form a reservoir (Tian et al., Citation2018). DDA-based liposomes are moderately or strongly responsive chemical molecule adjuvants that elicit a humoral response and powerful cell-mediated immunity against different types of antigens in the laboratory and large animals. These liposomes, in combination with immunomodulatory compounds, enhance vaccine immunogenicity (Hilgers & Snippe, Citation1992), induce DC maturation, and enhance plasmid-DNA uptake (Thanou et al., Citation2002; Slutter et al., Citation2009). Moreover, liposomes can be combined with viral vectors to form novel DNA delivery vectors (such as HVJ). HVJ-liposomes can be used as gene transfer vectors, mainly via the HVJ viral-cell fusion mechanism, to directly and effectively introduce DNA into host cells. Their complete DNA gene transfection efficiency is 30–100 times greater than that of naked DNA (Yoshida et al., Citation2006) and more than three times greater than that of cationic liposome delivery (Nakamura et al., Citation2001). Some studies have compared intramuscular injection of the HVJ-liposome HSP65 DNA vaccine with that of the IgHSP65 DNA vaccine using a gene gun. The IgHSP65 + mIL-12/HVJ vaccine significantly induces T-cell activation, produces cytotoxic CD8+ T cells and T cell helper responses, increases IFN-γ and cytokine concentrations in the supernatant, and reduces granulomas and pathological conditions in the lungs of challenged mice (Hobson, Citation2003, Huckriede et al., Citation2005). HVJ-liposomes could be developed as new anti-TB DNA-vaccine delivery systems in the future owing to their high antigen expression ability, low cytotoxicity and inflammatory response, and repeated injections that do not affect transfection efficiency (Pinheiro et al., Citation2011).
Figure 5. Liposome nanomaterials in vaccine delivery.
Note: Liposome vaccines with targeting ligands are captured by DC and activate cells through pattern recognition receptors, producing T cell-mediated immune responses, and mediating antigen presentation to T cell receptors, causing APC maturation with antigen processing and presentation.
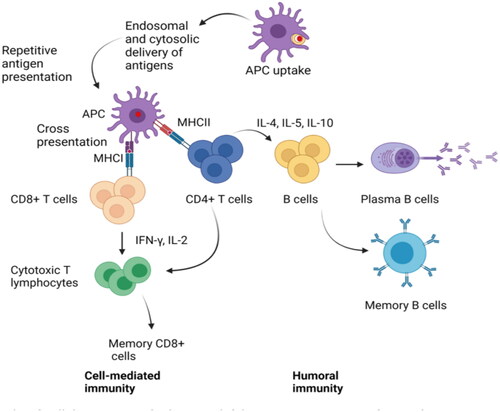
2.8. Virus-like nanoparticles and virosomes in vaccine delivery
Virus-like nanoparticles (VLP) and virosomes, containing viral envelope proteins, encapsulate plasmid DNA; the viral envelope protein makes VLP more immunogenic than polymer nanoparticles and liposomes during DNA-vaccine delivery against M. tuberculosis (Shakouri et al., Citation2016). The mechanism of action of VLP vaccines has been described in detail in previous publications (); VLP mimics a virus but lacks a genome, and therefore cannot replicate. VLP and virosome vaccines bind to targeted/non-targeted cells; subsequently, the targeted virus exhibits anti-TB protection by reduced infectious doses compared with non-targeted cellular pathogens. VLP and virosomes can replicate or non-replicate and contain the genomic information of the necessary antigens in their genome. Their replication mechanism remains intact on use, and replicates the viral vector vaccine to produce more vaccine viruses and infect other APCs. The antigen is displayed on the cell surface and stimulates CD4+ and CD8+ T cells (Chung et al., Citation2020). Recently, a novel Ag85A-ESAT6 fusion antigen vaccine containing (LV-AEG/SVGmu), a new DC-targeted recombinant lentivirus targeting the M. tuberculosis lentiviral vector, has been constructed; it is highly inclined to transfect DC-SIGN-expressing cells and expresses its fusion antigen in vitro and in vivo. This vaccine induces an antigen-specific lymphoid proliferation response and increases the secretion of Th1 cytokines (including IL-2 and IFN-γ) (Shakouri et al., Citation2016, Safar et al., Citation2020). Furthermore, a new DNA vaccine virus vector, pUMVC6, containing an IE promoter expression vector for cytomegalovirus and a kanamycin marker at the 5′ end (Safar et al., Citation2020, Hanif et al., Citation2010) has recently been constructed. Its delivery virus vector contains human IL-2 peptides as immunostimulants, allowing the antigen gene to secrete cytoplasmic proteins in the cell, triggering a strong immune response in healthy volunteers (Wu et al., Citation2016). As viral vectors enhance the ability of the BCG vaccine to combat TB compared with non-targeted lentiviral vectors, targeted lentiviral vectors should be designed for enhanced delivery using heterologous proteins in the future.
Figure 6. Virus-like nanoparticles in vaccine delivery.
Note: VLP or virosome vaccines bind to targeted/non-targeted cells, and the targeted virus causes anti-TB protection with infectious doses that are significantly lower than those involving non-targeted cellular pathogens. The replication mechanism remains intact, and is used to replicate the viral vector vaccine, produce more viruses, and infect other APCs. The antigen is displayed on the cell surface and stimulates CD4+ and CD8+ T cells.
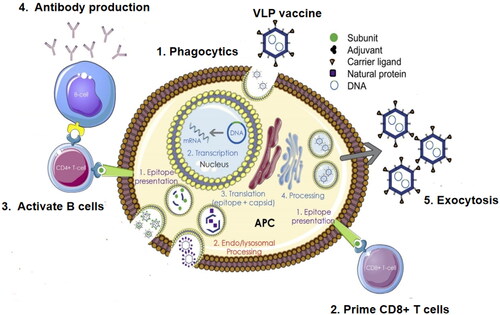
2.8. Self-assembled peptides and proteins in vaccine delivery
Self-assembled peptides and protein nanomaterials are injectable, biodegradable, and biocompatible. Through self-assembly, peptide nanomaterials can be applied in numerous fields, such as, drug delivery (small molecules and large molecules), regenerative medicine, and nano-biotechnology (Yu et al., Citation2016). The multi-epitope DNA vaccine HSP65 scaffold is a novel transplantation strategy that uses a protein-engineering backbone design. Five T-cell epitope antigens derived from the PE19, PPE25, MTB10.4, ESAT-6, and Ag85B proteins of the H37Rv M. tuberculosis strain of the pPES epitope–HSP65 scaffold, have been inserted into the scaffold protein to enhance epitope processing expression and immune response. Enzyme-linked Immunospot results indicate that the five T-cell epitope proteins expressed by this scaffold-construct induce IFN-γ+ and polyfunctional CD4+ T-cell responses, significantly enhancing the immune response of CTL in B cells and IL-2 levels (CD8+ T-cell responses) compared to other DNA constructs (naked DNA, epitope, or tandem HSP65 protein).
The delivery of protein expressed as an adjuvant molecule with plasmid DNA causes enhanced protective efficacy against a challenge infection with M. tuberculosis H37Rv, emphasizing the significant role of CD4+ helper T cells (Th1-type) in protection (Tanghe et al., Citation2001, Yang et al., Citation2011). shows self-assembled protein nanoparticles in vaccine design. B- and T-cell stimulation and activation, and the subsequent secretion of antigen-specific antibodies by plasma cells depend on the effective cross-linking between B-cell surface immunoglobulins (B-cell receptors, BCRs) and recognition patterns presented by the pathogen. The high-density and structurally ordered antigenic array presented by nanoparticle vaccines facilitate multiple binding events to occur simultaneously between the self-assembling protein nanoparticles and host-cell BCRs (Lopez-Sagaseta et al., Citation2016). Several paradigms have been reported for the design of TB DNA-vaccine delivery vectors using protein-backbone engineering. The synthetic antimicrobial peptide KLKL5KLK exhibits effective immunostimulant properties that enhance and prolong immune responses against M. tuberculosis in combination with DNA vaccines (Li et al., Citation2008). Therefore, these self-assembled peptide and protein nanomaterials represent novel TB DNA-vaccine delivery systems with immense application prospects.
Figure 7. Self-assembled protein nanoparticles in vaccine delivery.
Note: Nanoparticle vaccines with self-assembled proteins facilitate potent generation and long-lived immuno-protection in germinal centers. These nanoparticles, loaded with the desired antigen, are designed to present multiple copies of the pathogen epitope in a highly ordered manner on the surface of the self-assembled nanoparticle.
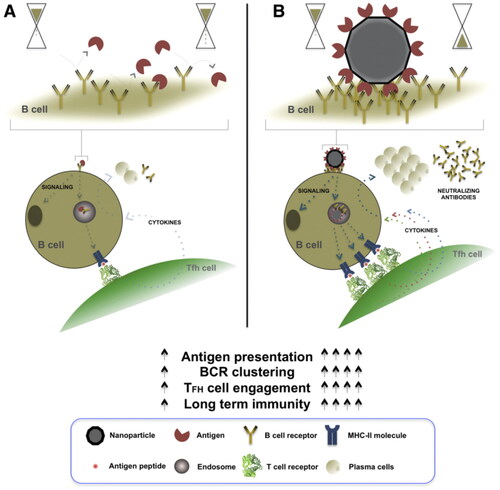
3. General review of nanomaterial delivery
DNA vaccines represent a third generation of new and improved vaccines for the prevention and treatment of TB. The acquisition of plasmid DNA is simple and does not require protein purification; the principle of TB DNA vaccination is also relatively simple (Huygen, Citation1998). For vaccine preparation, the dominant antigen gene fragment of TB is synthesized and amplified using primers, followed by an insertion of the DNA into the expression plasmid, its purification and amplification using transformed bacteria, combination with novel nanomaterial vectors, and finally immunization of the host, followed by screening and evaluation (Hobernik & Bros, Citation2018). Nanomaterials are used as antigens or adjuvants in DNA vaccines, which affect T cells or DCs and activate CTL to clear TB infection () (Yan et al., Citation2019). DCs are the most effective cross-presenting type of APC to initiate CD8+ T cell immunity in vivo, which is conducive to antigen presentation and uptake (Jorritsma et al., Citation2016). Novel nanomaterial-based mucosal administration enables barrier crossing via perioral, intranasal, and intratracheal routes, inducing local or systemic immunity at the mucosal site (Jin et al., Citation2019). Additionally, the use of novel nanomaterials, electroporation (Covello et al., Citation2014), micro-needling (Noh et al., Citation2022), and needle-free delivery systems (Mooij et al., Citation2019), such as gene guns or biological syringes which enhance skin-based delivery (), significantly enhance the transfection efficiency.
Figure 8. Nanomaterial vaccine delivery route.
Note: Nanomaterial delivery in the antigen or adjuvant of DNA-TB vaccines delivers vaccines into DCs, causing their maturation, and activates CTL to clear TB infection. These vaccines are also activated by TCR and effectively cross-present via APC cells, initiating CD8+ T-cell immunity in vivo.
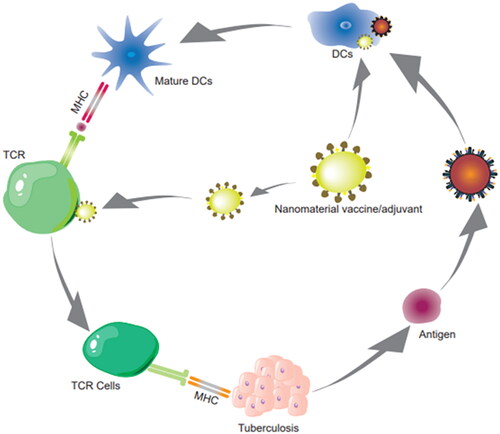
Table 1. Nanomaterials used in TB DNA vaccines.
4. Future research
Despite immense scientific progress in recent years, TB remains a significant threat to public health; nanomaterials could facilitate the development of better TB DNA vaccines and alleviate this global threat (Xu et al., Citation2018). Various nanocarriers have been investigated for use in TB vaccines, such as, lipid, polymeric, carbohydrate, and amino acid nanoparticles and viral nanoparticulate vectors. Other than immense applications in vaccine delivery, polymeric nanoparticles are promising adjuvants that enhance the protection of TB vaccines in vivo. Despite promising in-vitro and in-vivo results, further investigation is required before the clinical use of nanomaterial-based therapeutics and vaccines. From molecular mechanisms and detection tools to therapeutic strategies and vaccine development, nanomaterial research has focused on the generation, transmission, and role of ‘microscopic’ molecules. The emergence of fusion proteins has facilitated the development of TB vaccines. Non-viral vector nanomaterials for gene therapy load nontoxic short- or large-sized genetic materials that are effective in dividing and non-dividing cells. This material overcomes the difficulty of delivering traditional plasmid DNA to the nucleoplasm via the nuclear membrane and exhibits a low transfection rate and a high intracellular and nuclear uptake, gene delivery, and transfection efficiency (Mullick Chowdhury et al., Citation2016). Physical methods, such as electromechanical destruction, and the utilization of nanomaterials of cell membranes to deliver DNA molecules to the nucleus and cytoplasm with high throughput, enable the rapid expression of DNA molecules (Ding et al., Citation2017). Further preclinical (small and large animal studies) and clinical validation of toxicity and efficacy is required before the ‘bench to bedside’ transition of nanomaterial-based therapeutic vaccines. Nanomaterials could significantly reduce TB treatment duration, reduce the adverse effects of medications, and mitigate the development of multidrug resistance, facilitating a ‘TB-free world’ (Xu et al., Citation2018). Summarizing, novel nanomaterial-delivery vaccines against M. tuberculosis will play a crucial role in TB research and the development of future applications to control TB infection.
Disclosure statement
No potential conflict of interest was reported by the authors.
Additional information
Funding
References
- Abubakar I, Pimpin L, Ariti C, et al. (2013). Systematic review and meta-analysis of the current evidence on the duration of protection by bacillus Calmette-Guerin vaccination against tuberculosis. Health Technol Assess 17:1–372.
- Afrough S, Rhodes S, Evans T, et al. (2020). Immunologic dose-response to adenovirus-vectored vaccines in animals and humans: a systematic review of dose-response studies of replication incompetent adenoviral vaccine vectors when given via an intramuscular or subcutaneous route. Vaccines (Basel) 8:131:1–12.
- Agger EM, Cassidy JP, Brady J, et al. (2008). Adjuvant modulation of the cytokine balance in Mycobacterium tuberculosis subunit vaccines; immunity, pathology and protection. Immunology 124:175–85.
- Amidi M, Romeijn SG, Borchard G, et al. (2006). Preparation and characterization of protein-loaded N-trimethyl chitosan nanoparticles as nasal delivery system. J Control Release 111:107–16.
- Bettencourt P, Muller J, Nicastri A, et al. (2020). Identification of antigens presented by MHC for vaccines against tuberculosis. NPJ Vaccines 5:2:1–14.
- Cai H, Hu XD, Yu DH, et al. (2005). Combined DNA vaccine encapsulated in microspheres enhanced protection efficacy against Mycobacterium tuberculosis infection of mice. Vaccine 23:4167–74.
- Cai H, Tian X, Hu XD, et al. (2004). Combined DNA vaccines formulated in DDA enhance protective immunity against tuberculosis. DNA Cell Biol 23:450–6.
- Chan JM, Valencia PM, Zhang L, et al. (2010). Polymeric nanoparticles for drug delivery. Methods Mol Biol 624:163–75.
- Chatzikleanthous D, O’Hagan DT, Adamo R. (2021). Lipid-based nanoparticles for delivery of vaccine adjuvants and antigens: toward multicomponent vaccines. Mol Pharm 18:2867–88.
- Chung YH, Cai H, Steinmetz NF. (2020). Viral nanoparticles for drug delivery, imaging, immunotherapy, and theranostic applications. Adv Drug Deliv Rev 156:214–35.
- Coelho EA, Tavares CA, Lima Kde M, et al. (2006). Mycobacterium hsp65 DNA entrapped into TDM-loaded PLGA microspheres induces protection in mice against Leishmania (Leishmania) major infection. Parasitol Res 98:568–75.
- Covello G, Siva K, Adami V, Denti MA. (2014). An electroporation protocol for efficient DNA transfection in PC12 cells. Cytotechnology 66:543–53.
- Csaba N, Garcia-Fuentes M, Alonso MJ. (2009). Nanoparticles for nasal vaccination. Adv Drug Deliv Rev 61:140–57.
- Dalirfardouei R, Tafaghodi M, Meshkat Z, et al. (2020). A novel formulation of Mtb72F DNA vaccine for immunization against tuberculosis. Iran J Basic Med Sci 23:826–32.
- Ding X, Stewart M, Sharei A, et al. (2017). High-throughput nuclear delivery and rapid expression of DNA via mechanical and electrical cell-membrane disruption. Nat Biomed Eng 1:1–15.
- Dykman LA, Khlebtsov NG. (2017). Immunological properties of gold nanoparticles. Chem Sci 8:1719–35.
- Feltquate DM, Heaney S, Webster RG, Robinson HL. (1997). Different T helper cell types and antibody isotypes generated by saline and gene gun DNA immunization. J Immunol 158: 2278–84.
- Feng G, Jiang Q, Xia M, et al. (2013). Enhanced immune response and protective effects of nano-chitosan-based DNA vaccine encoding T cell epitopes of Esat-6 and FL against Mycobacterium tuberculosis infection. PLoS One 8(4):1–10.
- Franck CO, Fanslau L, Bistrovic Popov A, et al. (2021). Biopolymer-based Carriers for DNA Vaccine Design. Angew Chem 133:13333–51.
- Fries CN, Curvino EJ, Chen JL, et al. (2021). Advances in nanomaterial vaccine strategies to address infectious diseases impacting global health. Nat Nanotechnol 16:1–14.
- Ghebreyesus, TA. 2022. Global tuberculosis report 2021. Available at: https://www.who.int/teams/global-tuberculosis-programme/tb-reports/global-tuberculosis-report-2021.
- Goncalves ED, Bonato VL, da Fonseca DM, et al. (2007). Improve protective efficacy of a TB DNA-HSP65 vaccine by BCG priming. Genet Vaccines Ther 5:7:1–14.
- Grage K, Jahns AC, Parlane N, et al. (2009). Bacterial polyhydroxyalkanoate granules: biogenesis, structure, and potential use as nano-/micro-beads in biotechnological and biomedical applications. Biomacromolecules 10:660–9.
- Hanif SN, Al-Attiyah R, Mustafa AS. (2010). DNA vaccine constructs expressing Mycobacterium tuberculosis-specific genes induce immune responses. Scand J Immunol 72:408–15.
- Hilgers LAT, Snippe H. (1992). DDA as an immunological adjuvant. Res Immunol 143(5):494–503.
- Ho W, Gao M, Li F, et al. (2021). Next-generation vaccines: nanoparticle-mediated DNA and mRNA delivery. Adv Healthc Mater 10(8):1–17.
- Hobernik D, Bros M. (2018). DNA vaccines-how far from clinical use? Int J Mol Sci 19(11):1–28.
- Hobson P. (2003). Mucosal immunization with DNA vaccines. Methods 31:217–24.
- Huckriede A, Bungener L, Stegmann T, et al. (2005). The virosome concept for influenza vaccines. Vaccine 23:S26–S38.
- Huygen K. (1998). DNA vaccines: application to tuberculosis. Int J Tuberc Lung Dis 2:971–8.
- Jiang W, Gupta RK, Deshpande MC, Schwendeman SP. (2005). Biodegradable poly(lactic-co-glycolic acid) microparticles for injectable delivery of vaccine antigens. Adv Drug Deliv Rev 57:391–410.
- Jiao H, Yang H, Zhao D, et al. (2018). Design and immune characterization of a novel Neisseria gonorrhoeae DNA vaccine using bacterial ghosts as vector and adjuvant. Vaccine 36:4532–9.
- Jin Z, Gao S, Cui X, et al. (2019). Adjuvants and delivery systems based on polymeric nanoparticles for mucosal vaccines. Int J Pharm 572:1–58.
- Jorritsma SHT, Gowans EJ, Grubor-Bauk B, Wijesundara DK. (2016). Delivery methods to increase cellular uptake and immunogenicity of DNA vaccines. Vaccine 34:5488–94.
- Kamath AT, Feng CG, Macdonald M, et al. (1999). Differential protective efficacy of DNA vaccines expressing secreted proteins of Mycobacterium tuberculosis. Infect Immun 67:1702–7.
- Khademi F, Derakhshan M, Yousefi-Avarvand A, Tafaghodi M. (2018). Potential of polymeric particles as future vaccine delivery systems/adjuvants for parenteral and non-parenteral immunization against tuberculosis: a systematic review. Iran J Basic Med Sci 21:116–23.
- Khader SA, Bell GK, Pearl JE, et al. (2007). IL-23 and IL-17 in the establishment of protective pulmonary CD4+ T cell responses after vaccination and during Mycobacterium tuberculosis challenge. Nat Immunol 8:369–77.
- Khan N, Best D, Bruton R, et al. (2007). T cell recognition patterns of immunodominant cytomegalovirus antigens in primary and persistent infection. J Immunol 178:4455–65.
- Koike Y, Yoo YC, Mitobe M, et al. (1998). Enhancing activity of mycobacterial cell-derived adjuvants on immunogenicity of recombinant human hepatitis B virus vaccine. Vaccine 16:1982–9.
- Kutzler MA, Weiner DB. (2008). DNA vaccines: ready for prime time? Nat Rev Genet 9:776–88.
- Li J, Zhao A, Tang J, et al. (2020). Tuberculosis vaccine development: from classic to clinical candidates. Eur J Clin Microbiol Infect Dis 39:1405–25.
- Li M, Yu DH, Cai H. (2008). The synthetic antimicrobial peptide KLKL5KLK enhances the protection and efficacy of the combined DNA vaccine against Mycobacterium tuberculosis. DNA Cell Biol No 27: 405–13.
- Liang Y, Wu X, Zhang J, et al. (2008). The treatment of mice infected with multi-drug-resistant Mycobacterium tuberculosis using DNA vaccines or in combination with rifampin. Vaccine 26:4536–40.
- Lima KM, Santos SA, Lima VM, et al. (2003). Single dose of a vaccine based on DNA encoding mycobacterial hsp65 protein plus TDM-loaded PLGA microspheres protects mice against a virulent strain of Mycobacterium tuberculosis. Gene Ther 10:678–85.
- Lima VM, Bonato VL, Lima KM, et al. (2001). Role of trehalose dimycolate in recruitment of cells and modulation of production of cytokines and NO in tuberculosis. Infect Immun 69:5305–12.
- Lopez-Sagaseta J, Malito E, Rappuoli R, Bottomley MJ. (2016). Self-assembling protein nanoparticles in the design of vaccines. Comput Struct Biotechnol J 14:58–68.
- Lowrie DB, Tascon RE, Colston MJ, Silva CL. (1994). Towards a DNA vaccine against tuberculosis. Vaccine 12:1537–40.
- Mangtani P, Nguipdop-Djomo P, Keogh RH, et al. (2018). The duration of protection of school-aged BCG vaccination in England: a population-based case-control study. Int J Epidemiol 47:193–201.
- Marks SM, Flood J, Seaworth B, et al. (2014). Treatment practices, outcomes, and costs of multidrug-resistant and extensively drug-resistant tuberculosis, United States, 2005–2007. Emerg Infect Dis 20:812–21.
- Mikusova V, Mikus P. (2021). Advances in chitosan-based nanoparticles for drug delivery. Int J Mol Sci 22:1–185.
- Mooij P, Grodeland G, Koopman G, et al. (2019). Needle-free delivery of DNA: targeting of hemagglutinin to MHC class II molecules protects rhesus macaques against H1N1 influenza. Vaccine 37:817–26.
- Mullerpattan JB, Udwadia ZZ, Banka RA, et al. (2019). Catastrophic costs of treating drug resistant TB patients in a tertiary care hospital in India. Indian J Tubercul 66:87–91.
- Mullick Chowdhury S, Zafar S, Tellez V, Sitharaman B. (2016). Graphene nanoribbon-based platform for highly efficacious nuclear gene delivery. ACS Biomater Sci Eng 2:798–808.
- Nakamura N, Hart DA, Frank CB, et al. (2001). Efficient transfer of intact oligonucleotides into the nucleus of ligament scar fibroblasts by HVJ-cationic liposomes is correlated with effective antisense gene inhibition. J Biochem 129:755–9.
- Nemes E, Geldenhuys H, Rozot V, et al. (2018). Prevention of M. tuberculosis infection with H4:IC31 vaccine or BCG revaccination. N Engl J Med 379:138–49.
- Newman KD, Elamanchili P, Kwon GS, Samuel J. (2002). Uptake of poly(D,L-lactic-co-glycolic acid) microspheres by antigen-presenting cells in vivo. J Biomed Mater Res 60:480–6.
- Noh I, Lee K, Rhee YS. (2022). Microneedle systems for delivering nucleic acid drugs. J Pharm Investig 52:273–92.
- Ojha R, Kumar Pandey R, Prajapati VK. (2020). Vaccine delivery systems against tuberculosis. In: Kesharwani P, ed. Nanotechnology based approaches for tuberculosis treatment. India: Academic Press, 75–90.
- Okada M, Kita Y, Nakajima T, et al. (2007). Evaluation of a novel vaccine (HVJ-liposome/HSP65 DNA + IL-12 DNA) against tuberculosis using the cynomolgus monkey model of TB. Vaccine 25:2990–3.
- World Health Organization. 2020. Global tuberculosis report 2020: executive summary. Geneva.
- Parlane NA, Rehm BH, Wedlock DN, Buddle BM. (2014). Novel particulate vaccines utilizing polyester nanoparticles (bio-beads) for protection against Mycobacterium bovis infection - a review. Vet Immunol Immunopathol 158:8–13.
- Pereira VB, Zurita-Turk M, Saraiva TDL, et al. (2014). DNA vaccines approach: from concepts to applications. WJV 04:50–71.
- Pinheiro M, Lucio M, Lima JLFC, Reis S. (2011). Liposomes as drug delivery systems for the treatment of TB. Nanomedicine (Lond) 6:1413–28.
- Poecheim J, Barnier-Quer C, Collin N, Borchard G. (2016). Ag85A DNA vaccine delivery by nanoparticles: influence of the formulation characteristics on immune responses. Vaccines (Basel) 4(3):32:1-13.
- Puvacic S, Dizdarevic J, Santic Z, Mulaomerovic M. (2004). Protective effect of neonatal BCG vaccines against tuberculous meningitis. Bosn J Basic Med Sci 4:46–9.
- Rancan F, Blume-Peytavi U, Vogt A. (2014). Utilization of biodegradable polymeric materials as delivery agents in dermatology. Clin Cosmet Investig Dermatol 7:23–34.
- Safar HA, Mustafa AS, Amoudy HA, El-Hashim A. (2020). The effect of adjuvants and delivery systems on Th1, Th2, Th17 and Treg cytokine responses in mice immunized with Mycobacterium tuberculosis-specific proteins. PLoS One 15:1–17.
- Sampath P, Periyasamy KM, Ranganathan UD, Bethunaickan R. (2021). Monocyte and macrophage miRNA: potent biomarker and target for host-directed therapy for tuberculosis. Front Immunol 12:1–11.
- Satti I, Meyer J, Harris SA, et al. (2014). Safety and immunogenicity of a candidate tuberculosis vaccine MVA85A delivered by aerosol in BCG-vaccinated healthy adults: a phase 1, double-blind, randomised controlled trial. Lancet Infect Dis 14:939–46.
- Saxena M, Van TTH, Baird FJ, et al. (2013). Pre-existing immunity against vaccine vectors–friend or foe? Microbiology (Reading) 159:1–11.
- Schrager LK, Vekemens J, Drager N, et al. (2020). The status of tuberculosis vaccine development. Lancet Infect Dis 20:e28–e37.
- Schwendener RA, Ludewig B, Cerny A, Engler O. (2010). Liposome-based vaccines. Methods Mol Biol 605:163–75.
- Sefidi-Heris Y, Jahangiri A, Mokhtarzadeh A, et al. (2020). Recent progress in the design of DNA vaccines against tuberculosis. Drug Discovery Today 25:1971–87.
- Shakouri M, Moazzeni SM, Ghanei M, et al. (2016). A novel dendritic cell-targeted lentiviral vector, encoding Ag85A-ESAT6 fusion gene of Mycobacterium tuberculosis, could elicit potent cell-mediated immune responses in mice. Mol Immunol 75:101–11.
- Sia JK, Rengarajan J. (2019). Immunology of Mycobacterium tuberculosis Infections. Microbiol Spectr 7:1–57.
- Simmons JD, Stein CM, Seshadri C, et al. (2018). Immunological mechanisms of human resistance to persistent Mycobacterium tuberculosis infection. Nat Rev Immunol 18:575–89.
- Skeiky YA, Ovendale PJ, Jen S, et al. (2000). T cell expression cloning of a Mycobacterium tuberculosis gene encoding a protective antigen associated with the early control of infection. J Immunol 165:7140–9.
- Song Y, Zhou Y, van Drunen Littel-van den Hurk S, Chen L. (2014). Cellulose-based polyelectrolyte complex nanoparticles for DNA vaccine delivery. Biomater Sci 2:1440–9.
- Slutter B, Plapied L, Fievez V, et al. (2009). Mechanistic study of the adjuvant effect of biodegradable nanoparticles in mucosal vaccination. J Control Release, 138(2):113–21.
- Stylianou E, Griffiths KL, Poyntz HC, et al. (2015). Improvement of BCG protective efficacy with a novel chimpanzee adenovirus and a modified vaccinia Ankara virus both expressing Ag85A. Vaccine 33:6800–8.
- Tang J, Cai Y, Liang J, et al. (2018). In vivo electroporation of a codon-optimized BER(opt) DNA vaccine protects mice from pathogenic Mycobacterium tuberculosis aerosol challenge. Tuberculosis (Edinb) 113:65–75.
- Tanghe A, D’Souza S, Rosseels V, et al. (2001). Improved immunogenicity and protective efficacy of a tuberculosis DNA vaccine encoding Ag85 by protein boosting. Infect Immun 69:3041–7.
- Tejeda-Mansir A, García-Rendón A, Guerrero-Germán P. (2019). Plasmid-DNA lipid and polymeric nanovaccines: a new strategic in vaccines development. Biotechnol Genet Eng Rev 35:46–68.
- Thanou MM, Coos Verhoef J, Romeijn SG, et al. (1999). Effects of N-trimethyl chitosan chloride, a novel absorption enhancer, on Caco-2 intestinal epithelia and the ciliary beat frequency of chicken embryo trachea. Int J Pharm 185:73–82.
- Thanou L, Florea BI, Geldof M, et al. (2002). Quaternized chitosan oligomers as novel gene delivery vectors in epithelial cell lines. Biomaterials 23(1):153–59.
- Thomas TJ, Tajmir-Riahi HA, Pillai CKS. (2019). Biodegradable polymers for gene delivery. Molecules 24:3744:1–14.
- Tian M, Zhou Z, Tan S, et al. (2018). Formulation in DDA-MPLA-TDB liposome enhances the immunogenicity and protective efficacy of a DNA vaccine against Mycobacterium tuberculosis infection. Front Immunol 9:310:1–13.
- Tighe H, Corr M, Roman M, Raz E. (1998). Gene vaccination: plasmid DNA is more than just a blueprint. Immunol Today 19:89–97.
- Trunz BB, Fine PEM, Dye C. (2006). Effect of BCG vaccination on childhood tuberculous meningitis and miliary tuberculosis worldwide: a meta-analysis and assessment of cost-effectiveness. Lancet 367:1173–80.
- Villarreal DO, Siefert RJ, Weiner DB. (2015). Alarmin IL-33 elicits potent TB-specific cell-mediated responses. Hum Vaccin Immunother 11:1954–60.
- Viney K, Itogo N, Yamanaka T, et al. (2021). Economic evaluation of patient costs associated with tuberculosis diagnosis and care in Solomon Islands. BMC Public Health 21:1928:1–14.
- Wang D, Robinson DR, Kwon GS, Samuel J. (1999). Encapsulation of plasmid DNA in biodegradable poly(d,l-lactic-co-glycolic acid) microspheres as a novel approach for immunogene delivery. J Control Release 57:9–18.
- Wang QM, Sun SH, Hu ZL, et al. (2004). Improved immunogenicity of a tuberculosis DNA vaccine encoding ESAT6 by DNA priming and protein boosting. Vaccine 22:3622–7.
- Wang S, Liu H, Zhang X, Qian F. (2015). Intranasal and oral vaccination with protein-based antigens: advantages, challenges and formulation strategies. Protein Cell 6:480–503.
- Wedlock DN, Keen DL, McCarthy AR, et al. (2002). Effect of different adjuvants on the immune responses of cattle vaccinated with Mycobacterium tuberculosis culture filtrate proteins. Veterinary Immunology and Immunopathology 86:79–88.
- Wu M, Li M, Yue Y, Xu W. (2016). DNA vaccine with discontinuous T-cell epitope insertions into HSP65 scaffold as a potential means to improve immunogenicity of multi-epitope Mycobacterium tuberculosis vaccine. Microbiol Immunol 60:634–45.
- Wu M, Zhao H, Li M, et al. (2017). Intranasal vaccination with mannosylated chitosan formulated DNA vaccine enables robust IgA and cellular response induction in the lungs of mice and improves protection against pulmonary mycobacterial challenge. Front Cell Infect Microbiol 7:445:1–12.
- Xu K, Liang ZC, Ding X, et al. (2018). Nanomaterials in the prevention, diagnosis, and treatment of Mycobacterium tuberculosis infections. Adv Healthcare Mater 7:1700509:1–14.
- Yan S, Zhao P, Yu T, Gu N. (2019). Current applications and future prospects of nanotechnology in cancer immunotherapy. Cancer Biol Med 16:486–97.
- Yang XY, Chen QF, Li YP, Wu SM. (2011). Mycobacterium vaccae as adjuvant therapy to anti-tuberculosis chemotherapy in never-treated tuberculosis patients: a meta-analysis. PLoS One 6:1–8.
- Yoshida S, Tanaka T, Kita Y, et al. (2006). DNA vaccine using hemagglutinating virus of Japan-liposome encapsulating combination encoding mycobacterial heat shock protein 65 and interleukin-12 confers protection against Mycobacterium tuberculosis by T cell activation. Vaccine 24:1191–204.
- Yu CY, Huang W, Li ZP, et al. (2016). Progress in self-assembling peptide-based nanomaterials for biomedical applications. Curr Top Med Chem 16:281–90.
- Yu F, Wang J, Dou J, et al. (2012). Nanoparticle-based adjuvant for enhanced protective efficacy of DNA vaccine Ag85A-ESAT-6-IL-21 against Mycobacterium tuberculosis infection. Nanomedicine 8:1337–44.
- Zaman K. (2010). Tuberculosis: a global health problem. J Health Popul Nutr 28:111–3.
- Zaman M, Skwarczynski M, Malcolm JM, et al. (2011). Self-adjuvanting polyacrylic nanoparticulate delivery system for group A streptococcus (GAS) vaccine. Nanomedicine 7:168–73.
- Zhang X, Divangahi M, Ngai P, et al. (2007). Intramuscular immunization with a monogenic plasmid DNA tuberculosis vaccine: enhanced immunogenicity by electroporation and co-expression of GM-CSF transgene. Vaccine 25:1342–52.
- Zhao C, Liu X, Zhang X, et al. (2017). A facile one-step method for preparation of Fe3O4/CS/INH nanoparticles as a targeted drug delivery for tuberculosis. Mater Sci Eng C Mater Biol Appl 77:1182–8.