Abstract
Dihydromyricetin (DHM) is an important natural flavonoid that has attracted much attention because of its various functions such as protecting the cardiovascular system and liver, treating cancer and neurodegenerative diseases, and anti-inflammation effect, etc. Despite its great development potential in pharmacy, DHM has some problems in pharmaceutical applications such as low solubility, permeability, and stability. To settle these issues, extensive research has been carried out on its physicochemical properties and dosage forms to produce all kinds of DHM preparations in the past ten years. In addition, the combined use of DHM with other drugs is a promising strategy to expand the application of DHM. However, although invention patents for DHM preparations have been issued in several countries, the current transformation of DHM research results into market products is insufficient. To date, there is still a lack of deep research into the pharmacokinetics, pharmacodynamics, toxicology, and action mechanism of DHM preparations. Besides, preparations for combined therapy of DHM with other drugs are scarcely reported, which necessitates the development of dosage forms for this application. Apart from medicine, the development of DHM in the food industry is also of great potential. Due to its multiple effects and excellent safety, DHM preparations can be developed for functional drinks and foods. Through this review, we hope to draw more attention to the development potential of DHM and the above challenges and provide valuable references for the research and development of other natural products with a similar structure-activity relationship to this drug.
1. Introduction
Dihydromyricetin (2R,3R-3,5,7,3′,4′,5′-hexahydroxy-2,3-dihydroflavonol, DHM) is a polyphenol hydroxyl dihydroflavonol compound () with high content in ampelopsis grossedentata which has long been used for herbal tea and traditional Chinese medicine in China (CC Sun et al., Citation2021). Many studies have shown that DHM has many pharmacological activities. For example, DHM can protect the cardiovascular system through PI3K/Akt, Nrf2/HO-1, and SIRT3 signal pathways, and can also prevent or inhibit gastric cancer, lung cancer, hepatocellular carcinoma, colorectal cancer, breast cancer, and melanoma through Akt/STAT3, ERK/Akt, Akt/Bad, AMPK/MAPK/XAF1, Akt-mTOR, ROS/NF-κB, and mitochondrial apoptosis signal pathways (Liu et al., Citation2016; KJ Fan et al., Citation2017; Park et al., Citation2017; Zhang et al., Citation2017; Zhou et al., Citation2017; Chen et al., Citation2018; Li et al., Citation2021; Wang et al., Citation2021; Zhang et al., Citation2021). DHM can treat neurodegenerative diseases by Nrf2, SIRT1, and p53/p21 signal pathways, and can improve alcoholic liver disease, nonalcoholic fatty liver disease, and acute liver injury through JNK/AP-1/iNOS, p53, TGF-β1/Smad3, and AMPK/PGC-1α/ERRα signal pathways (Xie et al., Citation2015; Kou et al., Citation2016; Hu et al., Citation2018; JQ Ma et al., Citation2019; Yan et al., Citation2019; Zeng et al., Citation2019). DHM also has anti-inflammatory, antibacterial, antiviral, and skin protection effects, so it has great development potential () (Moon et al., Citation2018; Liang et al., Citation2020; Y Sun et al., Citation2021; Landis et al., Citation2022; Shi et al., Citation2022). DHM has not been reported to be toxic in the conventional dose range, and there is no significant change in physiological indexes such as the body weight and temperature after oral administration of 150–1500 mg/kg DHM in rats (Ma et al., Citation2014). Moreover, no obvious cytotoxicity of DHM has been found in normal cells, which indicates its high bio-safety (Cai et al., Citation2016).
Figure 1. The chemical structure (A) and ball-stick model (B) of dihydromyricetin (DHM); (C) the different pharmacological effects of DHM.
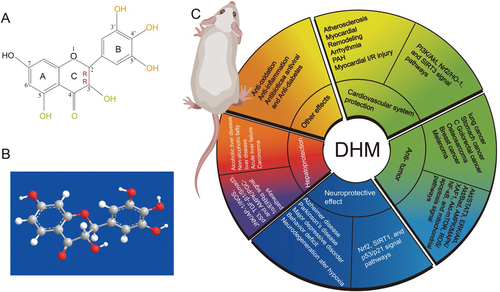
DHM has poor water solubility and only exists stably in low temperatures and weak acidic environments (pH 6.0) (CC Sun et al., Citation2021). If the temperature exceeds 100 °C, an irreversible oxidation reaction could occur. At 25 °C and 37 °C, the solubility of DHM in water is about 0.2 mg/ml and 0.9 mg/ml, respectively (L Fan et al., Citation2017). To increase its solubility, permeability, and stability, researchers have developed many new dosage forms for DHM, such as nano-preparations, microemulsions, gels, crystals, phospholipid complexes, and cyclodextrin complexes, etc., thereby improving the medicinal properties of DHM (CC Sun et al., Citation2020; S Geng, Jiang, et al., Citation2021; L Liu et al., Citation2022). It has been reported that DHM can distribute in various tissues and can pass through the blood-brain barrier after oral administration in rats, which provides further conditions for curing various diseases (L Fan et al., Citation2017). In addition, since the effect of this drug is not ideal when used alone because of its low titer, synergistic application of DHM with other anti-tumor drugs, liver protection drugs, anti-neurodegenerative diseases drugs, etc., should be considered.
So far, the physicochemical properties, analytical methods, pharmacological properties, and potential mechanisms of DHM have been reviewed in detail elsewhere (D Liu et al., Citation2019; Martínez-Coria et al., Citation2019; CC Sun et al., Citation2021). Sun et al. fully reviewed the physicochemical properties of DHM and discussed the effect of ascorbic acid on its stability and bioavailability (CC Sun et al., Citation2021). In addition, Liu et al. detailed the quantification methods and the main biological characteristics of DHM, as well as the methods to enhance its bioavailability (D Liu et al., Citation2019). Moreover, the pharmacological research progress and potential mechanisms of DHM in anti-tumor, metabolic diseases, liver diseases, cardiovascular diseases, skin diseases, and neurological diseases have also been reviewed in detail (J Zhang et al., Citation2018; Martínez-Coria et al., Citation2019; Tong et al., Citation2020; J Chen et al., Citation2021; L Chen et al., Citation2021; Wu et al., Citation2022). Therefore, these contents will not be reiterated here. As DHM has poor water solubility, permeability, and stability, over the past decade, many modern techniques have been adopted to design various dosage forms for DHM to settle these problems. However, although previous studies have reported a large number of DHM preparations, there is a lack of attention to the problems in the application of these preparations. Furthermore, despite the potential dosage forms reported in the previous articles and patents, most of these studies only focused on the preparation and the characterization of DHM preparations but lacked further investigations such as toxicology studies and drug efficacy studies that are essential for the transformation of this drug into clinical applications. Moreover, there are many other issues to consider in the future application of DHM. Based on the facts above, this review focused on the pharmaceutical designs of DHM and analyzed the reported dosage forms and some potential dosage forms of DHM. Through this review, we also analyzed the challenges and potential strategies of pharmaceutical design for the future applications of this drug combined with other drugs (e.g. antitumor drugs, antiviral drugs, antibacterial drugs, and hepatoprotective drugs), as well as some other issues to consider in the drug delivery of DHM, in order to provide valuable references for the development and application of DHM in the medicine and food industries and provide ideas for the research and development of natural products with a similar structure to DHM.
2. Pharmaceutical designs of DHM
2.1. Nano-preparations
2.1.1. Liposomes
Liposomes are drug carriers with the advantages of low toxicity, high biocompatibility, and low immunogenicity, which can carry and contain various hydrophilic and lipophilic drugs (Yuba, Citation2020). Common liposomes are easily engulfed by the mononuclear phagocyte system after entering the blood as drug carriers, leading to their rapid clearance (Cheng et al., Citation2022). To overcome such problems, modification of liposomes (e.g. PEGylation) is usually adopted to reduce the immunogenicity and improve the stability and the circulation time of liposomes (Ibaraki et al., Citation2021). Luo et al. used egg yolk phospholipids and cholesterol as carrier materials to prepare tea saponin (TS) coated polyethylene glycol DHM nanoliposomes (TS/CTS@DHM-lips). In these nanoliposomes chitosan (CTS) and TS form a cationic coating, which made the liposome surface positively charged, thus showing deep cell membrane permeability to negatively charged Escherichia coli and Staphylococcus aureus by electrostatic interaction, while DHM exerted increased anti-bacterial effect via sustained release through the coating. TS/CTS@DHM-lips had excellent entrapment efficiency and drug loading, and improved the hydrophilicity and solubility of DHM, making it a potential antibacterial agent (F Luo, Zeng, Chen, et al., Citation2021). In another study, DHM nanoliposomes prepared by Zhang et al. with soybean phosphatidylcholine and cholesterol as carrier materials and distearoyl phosphatidylethanolamine-polyethylene glycol-2000 as modifier reduced the release rate of DHM in vitro and prolong the residence time of DHM in rats, which improved the oral bioavailability of DHM (WJ Zhang et al., Citation2018). However, repeated intravenous injection of PEGylated liposomes into the same animal has been reported to result in an accelerated blood clearance phenomenon (Dams et al., Citation2000; El Sayed et al., Citation2020). Therefore, it is urgent and necessary to develop a strategy that can reduce or eliminate the immune response induced by PEGylated liposomes without affecting the therapeutic effect in vivo.
Multivesicular liposomes (MVL) are aggregates formed by close packing of non-concentric lipid bimolecular vesicles. Compared with ordinary liposomes, MVL can achieve high encapsulation and low leakage of drugs because of its ‘foam’ structural characteristics and ensure the storage and release of drugs in their original forms (Chaurasiya et al., Citation2022). DHM liposomes (DHM-lips) prepared by Luo et al. with cholesterol and egg yolk lecithin as drug carriers and PEG-4000 as modifier could effectively improve the solubility and membrane permeability of DHM, prolong the release rate and anti-bacterial time of DHM, and avoid the drug resistance of S. aureus (F Luo, Zeng, Yang, et al., Citation2021). The length of the non-polar terminal carbon chain and the number of unsaturated bonds of phospholipids used in MVL preparation may affect the tightness and fluidity of the liposome bilayer membrane, which would lead to the problems of sedimentation, aggregation, and drug leakage during MVL storage, thereby affecting the stability, entrapment efficiency, and drug release rate of MVL (Lu et al., Citation2021). We suggest using phospholipids with strong antioxidant capacity, such as dioleoyl lecithin, or coating a layer of sodium alginate on the liposome to improve the stability and entrapment efficiency of MVL. Moreover, in order to obtain an ideal drug release rate, some proper excipients can be adopted to modulate the drug release (e.g. using trioleic acid glyceride to slow down or trioctanoic acid glyceride to accelerate the drug release) (Y Li et al., Citation2020).
2.1.2. Polymer micelles
Polymer micelles have become ideal dosage forms for improving the bioavailability of flavonoids due to their good stability, biocompatibility, and solubilization (X Ma et al., Citation2019). In previous studies, our research group found that the polymer micelles prepared by electrostatic adsorption of negative-charged DHM with positive-charged natural polymers such as chitin and chitosan could improve the solubility and stability of the drug. Solutol®HS15 (HS 15) was a novel amphiphilic carrier material reported to have low toxicity and good solubilization (Nair et al., Citation2020). HS 15-loaded DHM self-assembled polymer micelle (DHM·MS) prepared by Ye et al. significantly improved the solubility, sustained release, and intestinal permeability of DHM and also showed better antioxidant and liver protection in drunk rats (Ye et al., Citation2021). However, DHM·MS only showed passive targeting delivery, thereby we suggest that HS 15 can be combined with ligands targeting liver tissue such as glycyrrhizic acid, or targeted by pH and inflammatory stimulation to improve the accumulation of DHM·MS in the liver tissue (Stecanella et al., Citation2021). It is noteworthy that, polymeric micelles are usually heterogeneous mixtures, even above the critical micelle concentration (CMC). In addition, polymeric micelles generally suffer from their structural instability and may easily disassemble to free polymers under dilute conditions or when exposed to environmental variations (e.g. pH and ionic strength), which may lead to low stability and certain toxic/side effects of the drug-loaded polymer micelles (S Lv et al., Citation2020). In previous studies, we found that micelles formed by mixed materials could make full advantage of each material, resulting in better properties such as higher stability and drug loading (Y Sun, Li, et al., Citation2019). Therefore, it is expected to increase the stability of the micelle system if other surfactants with the same hydrophilic chain as HS 15 were used to form mixed micelles (Y Sun, Li, et al., Citation2019).
2.1.3. Protein nanoparticles
Compared with other drug delivery systems, the protein nano-carrier delivery system has low antigenicity, good biocompatibility, and biodegradability (Lim et al., Citation2017). Nevertheless, protein nanoparticles have some disadvantages such as complex preparation process, poor stability, and difficulty in controlling side reactions, which need to pay attention to in practical use (Kianfar, Citation2021). Zein has strong hydrophobicity and good self-assembly properties, which makes it ideal for controlling the release of insoluble drugs (Kianfar, Citation2021). Sun et al. prepared nanoparticles with zein as the carrier material and sodium tyrosine as the stabilizer, and encapsulated DHM by hydrophobic interaction, resulting in DHM-embedded zein-caseinate nanoparticles (DZP) with good dispersion and stability. DZP not only improved the adhesion and retention time of DHM in mouse gastrointestinal tract but also significantly increased the bioavailability of DHM in rats compared with DHM suspension (CC Sun et al., Citation2020). Among various protein nano-carrier materials reported, albumin has many advantages such as water solubility, biodegradability, biocompatibility, non-toxicity, non-immunogenicity characteristics, and particularly tumor targeting ability, thereby it is a very promising protein nano-carrier material to ensure the chemotherapy efficacy and safety of anti-tumor drugs (Kunde & Wairkar, Citation2022). Protein nano-delivery system is easy to modify the surface, using magnetic materials, pH-responsive materials, or connecting targeting ligands, to further enhance the targeting and curative effect of drug-loaded nanoparticles (Aziz et al., Citation2022).
2.1.4. Nanocapsules
With the emergence of multidrug-resistant strains, the use of polymer nanocapsule delivery systems loaded with active compounds is considered as a promising strategy to overcome biomembrane resistance (Deng et al., Citation2020; Nikezic et al., Citation2020). The pharmaceutical nanocapsule is a nanosized drug-loaded spherical particle with core-shell morphology, which has the advantages of increased solubility and stability, increased efficiency of encapsulation, the possibility of reducing adverse effects of drugs, and reduction of therapeutic doses (Frank et al., Citation2015). However, there are still many safety problems in nanocapsules, such as the surface charge that may have a toxic effect on cells and some toxic reagents involved in the preparation process, which have hindered their further application (Lima et al., Citation2022). Eudradit Rs100® polymer is a positively charged material with strong mucosal adhesion and can provide higher adhesion for positively charged bacteria (Dalcin et al., Citation2017). DHM nanocapsules (NC-DHM) prepared by Dalcin et al. using Eudradit Rs100® can significantly reduce the formation of Pseudomonas aeruginosa biomembrane in the urinary catheters. Compared with free DHM, NC-DHM showed prolonged drug effects and stronger antibacterial activity (Dalcin et al., Citation2019). It is noteworthy that Eudradit Rs100® polymer can induce the death of the PBMC cell line (i.e. the most sensitive human cell line for evaluating the toxicity of various compounds), and lead to decreased cell viability and increased DNA damage. However, the addition of DHM into Eudradit Rs100® nanocapsules could not only avoid cytotoxicity and genotoxicity, but also prevent the pyrolysis and photolysis degradation of DHM, and maintain the antioxidant effect of DHM (Wei et al., Citation2022). Considering the strong antioxidant activity of DHM itself, we suggest that DHM could be added as an excipient into the nanocapsules of Eudradit Rs100® to prevent cytotoxicity when delivering other drugs (Guo et al., Citation2016).
In summary, the DHM nano-delivery system usually has excellent controlled drug release, special tissue targeting, and strong modifiability, so it is easy to obtain high drug bioavailability and efficacy. It should not be ignored that the preparation of DHM nano-preparations involves many toxic reagents, expensive excipients, and complex processes, thus researchers need to continue to study low-toxic, economic reagents and materials, and simplified processes. In addition, the previous research on DHM nano-delivery systems mainly focused on the preparation process and drug quality investigation but often ignored the further study of their pharmacological activity and safety, which should be paid more attention to in the future.
2.2. Microemulsion preparation
Compared with the nano-drug delivery systems, the microemulsion is another widely studied drug delivery system, which is excellent in improving oral absorption and preventing drug oxidation (Garavand et al., Citation2021). It has been reported that DHM microemulsion systems include ordinary microemulsion preparation, Pickering emulsion, and self-emulsifying drug delivery system.
Solanki et al. found that DHM microemulsion prepared with Capmul MCM as oil phase (DHM/ME-C), Cremophor EL as surfactant, and T-transcutol as co-surfactant would change from water-in-oil to bicontinuous phase with the addition of water, which regulated the dissolution and oral bioavailability of DHM (Solanki et al., Citation2012). Our group has prepared DHM microemulsions with different polarity oil phases and emulsifiers with different HLB values, during which we found that the selection of proper emulsifier and oil phase in the microemulsion system is very important. We also found that the dissolution of DHM in the weakly polar organic phase is better, probably due to its abundant hydroxyl groups, thereby weakly polar organic solvents (such as Capmul MCM) are expected to be better for preparing DHM microemulsions. The problem to be solved in the clinical application of DHM microemulsion is the irritation caused by the addition of a large amount of oil phase, emulsifier, and co-surfactant. Therefore, developing safe and non-irritative microemulsion components would be an important task for future research. In addition, the pH-dependent solubility of some drugs in the microemulsion preparation has also been a problem that needs to be solved in the future (Egito et al., Citation2021).
Pickering emulsion is a kind of emulsion with ultrafine solid particles as the emulsifier. It has excellent coalescence stability, loading capacity, controlled release, biodegradability, and biocompatibility, which can make up for the shortcomings of traditional emulsions (XM Li et al., Citation2020; Mwangi et al., Citation2020). The natural fiber extract cellulose nanocrystalline (CNC) is a stable and effective solid particle emulsifier with good biocompatibility and biodegradability. Pickering emulsion stabilized by CNC can achieve increased loading and sustained release of DHM and can improve the stability of DHM (P Shen et al., Citation2022). Lysozyme (LY) is a multifunctional natural single-chain protein, which not only acts as an antibacterial agent but also has many beneficial effects such as regulating intestinal flora and reducing inflammation (H Liu, Wang, et al., Citation2022). In the research of Geng et al., DHM was adsorbed on the surface of LY to form a three-dimensional network structure to stabilize Pickering high internal phase emulsion (HIPE). Therefore, Pickering HIPE loaded with DHM has the valuable properties of antibacterial effect, lipid oxidation resistance, and lutein protection (Geng et al., Citation2022). It has been reported that some toxic and side effects such as allergy, nerve injury, and renal injury can be caused by some particle stabilizers in Pickering emulsion, which necessitated further safety studies of Pickering emulsion in the future (Zhang et al., Citation2020).
Self-emulsifying drug delivery system (SEDDS) is a homogeneous mixture of drugs, co-emulsifiers, hydrophilic emulsifiers, and oil phase, which can spontaneously form O/W emulsion or microemulsion under gastrointestinal peristalsis or mild stirring in ambient temperature, but it has some defects such as instability and large gastrointestinal irritation (Salawi, Citation2022). However, the solid self-emulsifying drug delivery system (S-SMEDDS) can well overcome these defects (C Sun et al., Citation2018). Wang et al. prepared DHM-loaded liquid self-microemulsion (DHM/L-SEDS) with octyl and decyl glycerate as the oil phase and polyglyceryl-6 monooleate and polyglycerol polyricinoleate as the co-emulsifiers. Then DHM-loaded solid self-microemulsion (DHM/S-SEDS) was prepared by adsorbing DHM/L-SEDS onto the solid adsorbent Aerosil 300. In vitro and in vivo experiments showed that DHM/S-SEDS improved the antioxidant activity and bioavailability of DHM, and showed good fluidity and stability under various storage conditions within 10 weeks (Wang et al., Citation2019). Theoretically, the increase in the specific surface area, the number of voids, and the depth of pores in the particle may lead to the difficulty of drug dissolution and the spontaneous aggregation of drug molecules (i.e. the ‘aging’ of crystal nucleus). Therefore, the above issues should be considered to prevent the aging phenomenon of S-SMEDDS during preparation (Kim et al., Citation2014).
2.3. Gel preparation
The reported gel preparations of DHM included emulsion gel and hydrogel. Emulsion gel is a kind of emulsion with a soft-solid structure, which endows liquid oil with the ability of controlled drug release. Pickering emulsion gel is stabilized by solid particles, which can be used as a controlled release carrier for bioactive compounds while maintaining gel properties (S Li et al., Citation2020; P Lv et al., Citation2020). However, due to the varied physicochemical properties of different solid particles, the Pickering emulsion gel may have flocculation and coalescence problems (S Li et al., Citation2020). It was reported that DHM could form a three-dimensional network with triglyceride, so DHM can be used as a particle stabilizer of Pickering emulsion gel. The prepared DHM-Pickering emulsion gel could not only effectively protect nutritious food in the oil phase, but also bring out a variety of health promotion functions of DHM (P Lv et al., Citation2020; S Geng, Jiang, et al., Citation2021). Similarly, Geng et al. prepared a Pickering emulsion gel with DHM and high-amylose corn starch (HCS), in which DHM formed supramolecular complexes (DHM/HCS) with some amylose molecules on the surface of HCS particles by non-covalent interaction. DHM/HCS stabilized Pickering emulsion gels by establishing a relationship of ‘molecular interaction-particle characteristics-emulsion gel properties’, which enhanced the gel structure and emulsifying ability of Pickering emulsion gels (S Geng, Liu, et al., Citation2021). Because DHM emulsion gel has the characteristics of high nutrition and high stability, it could be considered for functional food in the future.
Hydrogels are a major nutrient delivery system with the advantages of pH-responsive delivery and controlled release. Protein-polysaccharide hydrogels have excellent biocompatibility, and their gelation often occurs when the polymer concentration is low (Narayanaswamy & Torchilin, Citation2019). Wei et al. prepared hydrogel with ovotransferrin (OVT) fiber and xanthan gum (XG) as the materials. OVT fiber has good biocompatibility. XG is a common food additive. The hydrogel carrier assembled by electrostatic association has high gel strength and viscosity, which can significantly increase the drug loading of DHM in the hydrogel and release the drug slowly (Wei et al., Citation2020). Therefore, protein-polysaccharide hydrogel provides a new idea for the nutrition delivery system of natural drug extracts. Nevertheless, protein-polysaccharide hydrogels may suffer from poor stability (e.g. melting, aggregation, and condensation) and uncontrollable drug release rates under some extreme conditions (e.g. high temperature, strong acid and alkaline conditions), which is worthy of more attention in future research (K Liu et al., Citation2021; Manzoor et al., Citation2022).
It is worth mentioning that traditional hydrogels can be mixed with other dosage forms to produce new functions. Dalina et al. prepared hydrogels with ethyl cellulose (EC) as the matrix material, nipagin as the preservative, propylene glycol as the wetting agent, and Tween 80 as the solubilizer. DHM nanocapsules (NC-DHM as mentioned in ‘2.1.4 Nanocapsules’) were added to the hydrogels. This NC-DHM hydrogel exhibits photoprotective activity against ultraviolet radiation-induced DNA damage and has good safety for the skin (Dalcin et al., Citation2021), thereby we suggest that it could be developed for the cosmetics industry.
2.4. Crystal
Drug cocrystal is formed by hydrogen bond self-assembly between active pharmaceutical ingredients and appropriate cocrystal former. Drug cocrystal can modify the physicochemical properties of drugs without changing the covalent structure of drug molecules (Kavanagh et al., Citation2019). DHM is an ideal candidate for cocrystal due to its multiple hydrogen bond receptors and donors. Therefore, the preparation of highly soluble cocrystal can be an effective strategy to improve the physicochemical properties and biopharmaceutical properties of DHM (Morales et al., Citation2017).
Wang et al. obtained (+)DHM cocrystal by co-crystallizing DHM with the of theophylline. Compared with racemic DHM, (+)DHM metabolized more slowly in mice and showed better anti-inflammatory activity and bioavailability (C Wang, Xiong, et al., Citation2016). In order to prevent the DHM cocrystal from precipitating during the dissolution and maintain its supersaturation concentration, suitable precipitation inhibitors should be used. For example, when PVP K30 is used as the precipitation inhibitor, the stability of DHM-caffeine cocrystal and DHM-urea cocrystal in the medium can be enhanced, and the supersaturation concentration can be maintained, thus improving the oral bioavailability of DHM (C Wang, Tong, et al., Citation2016). The effects of optical isomers are often different. For example, (−)gossypol has significant anticancer properties, while (+)gossypol has almost no such biological activities (H Liu, Zhang, et al., Citation2022). Since the pharmacological effects of (+)DHM and (−)DHM can be different, it is necessary to develop monoisomer drug preparations in the future to reduce ineffective substances for patients and avoid potential adverse/side effects (Tong et al., Citation2015).
Drug-drug cocrystal consists of two or more active drug components, and the ideal balance of physicochemical properties can be achieved through the non-covalent interaction among different drugs (Shinozaki et al., Citation2019). Both DHM and pentoxifylline (PTX) have the effects of treating vascular diseases and anti-cancer. They can form DHM-PTX cocrystal hydrate by hydrogen bonding. After forming cocrystal, DHM and PTX can be released synchronously and have a synergistic anti-cancer effect on HepG2 cells in vitro (L Liu et al., Citation2022). Similarly, Li et al. prepared berberine hydrochloride (BER) and DHM as BER-DHM cocrystal, which not only improved the thermal stability and solubility of DHM but also showed a synergistic anticancer effect on HT29 cancer cells in vitro (P Li et al., Citation2020).
The melting point should be strictly controlled in the process of developing drug cocrystals. A high melting point will lead to poor solubility, while a low melting point will decrease the stability of drugs. Nevertheless, there are still some problems in drug cocrystal, such as the instability in supersaturation state, how to choose cocrystal ligands, and unclear absorption mechanism in vivo, which warrant further study (Chaves et al., Citation2020).
2.5. Gastric floating preparation
DHM can exist stably in low temperatures and weak acidic environments (pH 6.0). When pH drops from 8.0 to 6.0, the absorption of DHM is significantly enhanced, suggesting that DHM is easier to be absorbed under acidic conditions (Shen et al., Citation2015). After oral administration of DHM in rats, the maximum concentration of DHM in the gastrointestinal tract is much higher than that in the heart, spleen, liver, lung, and kidney. DHM is a natural extract with a low titer, which often needs a large dose for administration to produce a curative effect. Based on the above facts, we suggest the gastric floating dosage forms may be ideal for DHM preparations (Tong et al., Citation2015).
After oral administration, the volume of gastric floating preparations can expand under the action of the gastric environment, making its density less than that of gastric contents. Therefore, they can float in gastric juice, thus prolonging the residence time of the drug in the stomach (H Liu, Wang, et al., Citation2021). In our previous study, DHM sustained-release gastric floating tablets (DHM-GFTs) were prepared by using NaHCO3 as the foaming agent, ethyl cellulose (EC) as the adhesive, povidone K30 (PVP K30) and hydroxypropyl methylcellulose K4M (HPMC K4M) as the hydrophilic gel matrix. Compared with DHM powder, DHM-GFTs had a long-term gastric floating ability and sustained release for over 12 h, which significantly prolonged the residence time of DHM in rabbits and improved the oral bioavailability (H Liu et al., Citation2019). However, the drug loading content of DHM-GFTs was low (about 24%) and could not effectively avoid the large fluctuation of the blood drug concentration. Then, we further prepared DHM gastric floating pills (DHM-GFPs). With the optimal formulation, DHM-GFPs showed a much higher drug loading content (about 33%). Moreover, the gastric floating time and sustained release time were prolonged to over 24 h, which significantly reduced the fluctuation of the blood drug concentration and resulted in a better anti-inflammatory effect (H Liu, Gan, et al., Citation2021). Despite the advantages above, some gastric floating preparations may have incomplete absorption due to gastric emptying, gastric peristalsis, and gastric contents, thus reducing the oral bioavailability of the drug (Rajora & Nagpal, Citation2022). To overcome these problems, the development of gastric floating preparations with biological adhesion and mucinous adhesion, or using excipients with high density and high expansibility could be considered in the future (Iglesias et al., Citation2020).
2.6. Phospholipid complex
One of the reasons for the low oral bioavailability of drugs may be due to their limited solubility and/or low permeability (Kuche et al., Citation2019). Bombardelli et al. found that naturally extracted drugs have a special affinity with phospholipids, and they could form phospholipid complexes in water based on non-covalent interaction (Bombardelli, Citation1991). Phospholipid complex has amphiphilic characteristics, which can increase the solubility and stability of insoluble drugs, providing an effective method to solve the low oral bioavailability of naturally extracted drugs (Kuche et al., Citation2019). Liu et al. prepared a DHM-lecithin complex by solvent volatilization method. DHM and lecithin are completely combined by non-covalent bonds, producing a DHM-lecithin complex that could improve the solubility and antioxidant activity of DHM. As a natural antioxidant, DHM has the potential to replace butylated hydroxyanisole which is the most widely used synthetic antioxidant (Liu et al., Citation2012). Similarly, Zhao et al. prepared DHM phospholipid complex (DHM-HSPC com), in which DHM was dispersed in hydrogenated soybean phosphatidylcholine (HSPC) carrier in an amorphous state, so DHM-HSPC com significantly improved the solubility and drug loading content of DHM. In type II diabetes mellitus rats, DHM-HSPC com showed better hypoglycemic activity in vivo compared with the same dose of free DHM, and decreased the clearance rate and apparent distribution volume of DHM in vivo, thus improving the bioavailability of DHM (Zhao et al., Citation2019). Therefore, the DHM phospholipid complex would be a promising pharmaceutical preparation to improve the bioavailability of DHM. When preparing phospholipid complex, the drug/lipid ratio would affect the drug binding rate, thereby choosing the appropriate drug/lipid ratio is the key factor to improving the drug loading in phospholipid complex (Biswas et al., Citation2019). Despite the great potential of the phospholipid complex technology, the instability (e.g. risk of aggregation and chemical degradation) and the drug leakage that can lead to irregular pharmacokinetics are still major issues which need further studies to settle in future studies (Kuche et al., Citation2019).
2.7. Cyclodextrin inclusion complex
Cyclodextrins (CD) are a kind of semi-natural cyclic oligosaccharides with conical hollow structures, which can improve the solubility of drugs. The whole B ring and part of the C ring of DHM could be embedded into the cavity of CD through non-covalent interactions such as hydrogen bonding (YP Wu et al., Citation2020). Therefore, inclusion technology may be an effective strategy to improve the low water solubility of DHM. Particularly, the hydroxypropyl-β-cyclodextrin (HP-β-CD) not only has the good properties of β-cyclodextrin (β-CD) such as low price and good safety but also has the characteristics of biodegradability and high solubility (Mu et al., Citation2022). Ruan et al. prepared DHM inclusion complexes DHM/HP-β-CD and DHM/β-CD using HP-β-CD and β-CD respectively, in which the solubility and dissolution of DHM were positively correlated with the addition amount of HP-β-CD and β-CD (Ruan et al., Citation2005). In the hyperlipidemia zebrafish model, DHM/HP-β-CD and DHM/β-CD showed better lipid-lowering activity than free DHM. In particular, DHM/HP-β-CD could induce the apoptosis of human HepG2 cells in a dose-dependent manner (Yang et al., Citation2011). It is noteworthy that although DHM/HP-β-CD showed good solubility and thermal stability, Liu et al. found that the antioxidant capacity of DHM was weakened due to the shield of HP-β-CD, which necessitated further research of DHM/HP-β-CD to settle this problem (Liu et al., Citation2012). Moreover, attention should also be paid to the problems of poor stability in acidic or alkaline environments, the presence of residual organic solvents, low drug encapsulation efficiency, and complex preparation process, which have limited the application of cyclodextrin inclusion complexes (Cid-Samamed et al., Citation2022).
2.8. Others
In addition to the above-mentioned dosage forms for DHM, there are still several reported DHM dosage forms such as solid dispersions, covalent polymers, and composite active packaging films. Solid dispersions are one of the most promising strategies to improve the oral bioavailability of poorly water-soluble drugs (Tran & Park, Citation2021). The solid dispersion prepared by Ruan et al. with hydrophilic polymers PVP K30 and PEG-6000 as carriers significantly improved the solubility and dissolution rate of DHM (Ruan et al., Citation2005). However, the stability of the solid dispersion and its easy absorbing water can be two problems that lead to phase separation, crystal growth, or crystal conversion during storage, which may further result in decreased solubility and dissolution rate (Cid et al., Citation2019). Liu et al. prepared DHM-sugar beet pectin (SBP) covalent polymer (SBP/DHM), in which DHM improved the emulsifying ability of SBP through non-covalent interaction, while SBP increased the solubility and antioxidant performance of DHM. Because SBP/DMH had a strong protein binding ability, it could be used as a noncompetitive inhibitor and anti-competitive mixed inhibitor of α-glucosidase, and its inhibitory effect is stronger than free DHM, and it exhibited the potential to treat non-insulin-dependent diabetes mellitus (Liu et al., Citation2018). Based on this, the covalent polymers formed by DHM and some natural polysaccharides can be potential ingredients to develop functional foods. Flavonoid-grafted polysaccharides can not only change the structural characteristics of polysaccharides but also improve the physicochemical properties and biological characteristics of polysaccharides. However, studies on the applications of flavonoid-grafted polysaccharides are very limited, and the safety of flavonoid-grafted polysaccharides is often ignored (J Liu et al., Citation2020; Bhanushali et al., Citation2022). Active packaging is able to inhibit microbial growth, enzymatic reaction, and oxidation reaction by adding various active substances into the packaging materials, so as to prolong the shelf life of packaged foods and improve their safety. In recent years, active packaging is considered as one of the best food preservation measures (Gulcin, Citation2020). Xie et al. prepared composite active packaging film (KGM/GG-DHM) with konjac glucomannan (KGM), gellan gum (GG), and DHM. KGM/GG-DHM had good thermal stability, controlled release behavior, biocompatibility, antioxidant activity, and antibacterial activity against Escherichia coli and Staphylococcus aureus (W Xie et al., Citation2021). The main shortcoming of active packaging is the uncontrollable migration rate of active compounds inside. Because of the low molecular weight of most active compounds, their release rates from active packaging are potentially rapid, in turn leading to a shorter shelf-life (Almasi et al., Citation2021). Therefore, it is still necessary to study the stability, release mechanism, and diffusion kinetics of active food packaging materials in the future, and further evaluate the timeliness of active components in the active packaging films.
In summary, the application of various techniques to prepare dosage forms for DHM can increase its solubility, permeability, and stability, which serves the needs of clinical treatment. This part introduced most of the reported DHM dosage forms, which were summarized in . In addition to the dosage forms introduced above, we can also consider the research of other dosage forms for DHM such as dropping pills, microcapsules, microspheres, etc., as well as the combination of various dosage forms in the future, so as to expand the application of DHM.
Table 1. Reported dosage forms for DHM and their main functions.
It is noteworthy that the current transformation of DHM research results into an application is not satisfactory. Although invention patents about DHM have been issued in various countries, so far only one DHM capsule has been approved for the market in the United States, which was probably due to the low titer of DHM that has led to unsatisfactory drug effect when used alone (D Liu et al., Citation2019; J Shen et al., Citation2022). The combination of DHM and other drugs may be a promising strategy to expand the application of DHM. Apart from medicine, it should be noted that there are still very few reports on the application of DHM in the food industry. As mentioned in the previous parts, researchers can also consider combining DHM with a healthy lifestyle and developing it as the ingredient in functional drinks and foods.
3. Pharmaceutical designs for the combined application of DHM with other drugs
3.1. Combination with anti-tumor drugs
DHM has a variety of anti-tumor mechanisms. Researchers have found that DHM combined with other anti-tumor drugs can reduce multidrug resistance (MDR), toxicity, and side effects, and enhance the curative effects, therefore the combination of DHM and other anti-tumor drugs have a good application prospect (Ferte et al., Citation1999).
Platinum drugs can combine with DNA to cause tumor cytotoxicity, which resulted in a high cure rate in clinical solid tumors (Zhang et al., Citation2022). However, due to their serious toxicity and side effects on normal tissues, especially kidneys, platinum drugs are limited in clinical application (Stankovic et al., Citation2020). It has been reported that DHM can be used as a reasonable chemosensitizer and attenuator of platinum drugs by activating the p53/Bcl-2 signal pathway, NF-κB/p65 signal pathway, and inhibiting the expression of multidrug resistance protein 2 (MRP2/ABCC2) () (Jiang et al., Citation2015; Wu et al., Citation2016; Wang et al., Citation2017). Adriamycin (ADR) is a highly effective anthracycline anti-tumor drug, which takes effects by inserting into the DNA of tumor cells. The clinical application of ADR is limited by disseminated intravascular coagulation (DIC) such as heart dysfunction and even heart failure (C Liu et al., Citation2020). Researchers have found that DHM can reverse MDR, reduce DIC, and enhance the curative effect by inhibiting activation of NLRP3 inflammatory corpuscle, P-gp function, MAPK/ERK signal pathway, p38MAPK signal pathway, and p53 signal pathway ( and ) (Zhao et al., Citation2014; Zhu et al., Citation2015; Xu et al., Citation2017; Y Sun et al., Citation2018; Y Sun, Liu, et al., Citation2019; Z Sun et al., Citation2020). Therefore, the combination with DHM is an effective way to solve the above problems of ADR. In addition to platinum drugs and ADR, DHM can also improve the efficacy of all-trans retinoic acid and reverse the MDR of 5-fluorouracil through various mechanisms (Zhou et al., Citation2014; He et al., Citation2018; M Wu et al., Citation2020).
Figure 2. Mechanism of synergistic application of DHM with platinum drugs (A: DHM acts in combination with nedaplatin (NDP) to regulate the balance of Bcl-2/Bax and Bcl-2/Bak ratios through the p53/Bcl-2 signaling pathway and inhibits NDP-induced ROS production, thereby enhancing the chemosensitivity of hepatocellular carcinoma cells to NDP; B: DHM enhances the anticancer activity of oxaliplatin (OXA) by inhibiting MRP2 transcription and suppressing the nuclear translocation of Nrf2; C: DHM inhibits MRP2 expression to restore the sensitivity of tumor cells to OXA and reduces NF-κB/p65 nuclear translocation by inhibiting the expression of NF-κB/p65, thereby inhibiting the expression of Nrf2 signaling to achieve a synergistic anti-cancer effect with OXA.).
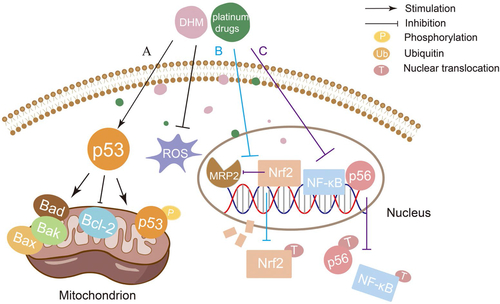
Figure 3. Mechanism of synergistic application of DHM with ADR (A: DHM enhances the antitumor activity of ADR in a p53-dependent manner and prevents ADR-induced DIC by inhibiting MDM2-mediated degradation of ARC ubiquitination; B: DHM downregulates P-gp by blocking the ERK pathway, causes intracellular Ca2+ accumulation by inhibiting SORCIN, induces apoptosis and reverses MDR through the mitochondrial, endoplasmic reticulum and p53 pathways; C: Combination of DHM and ondansetron (OND) suppresses P-gp expression by downregulating SORCIN, p-ERK and p-Akt expression, thereby enhancing the antitumor activity of ADR.).
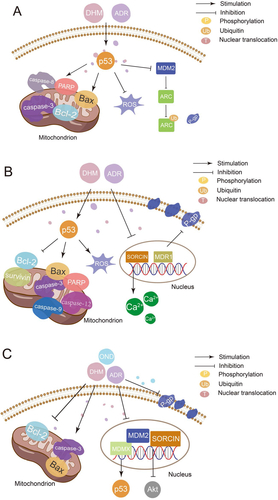
Figure 4. Mechanism of synergistic application of DHM with ADR (A: DHM can be used as an adjuvant to ADR by activating p38MAPK and AMPKα/GSK-3β/SOX2 signaling pathways; B: DHM attenuates ADR cardiotoxicity by activating SIRT1 and inhibiting NLRP3 inflammatory vesicles; C: DHM reverses the sensitivity of drug-resistant cancer cells to ADR by inhibiting p53-mediated survivin.).
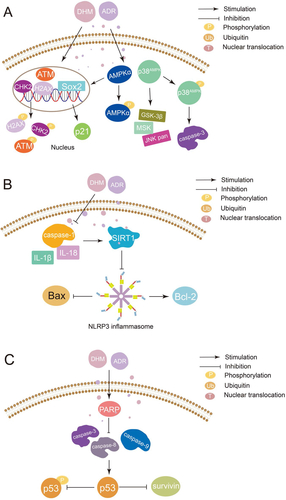
Although the combination of DHM and other anti-tumor drugs could lead to good coordination in mechanism, there are still some problems in the design of dosage forms, such as the differences in the physicochemical properties, absorption sites, pharmacokinetic behaviors, and effective doses of different drugs, resulting in the failure of the combination strategy (Santana et al., Citation2020). Therefore, when designing the combined preparations, we should consider the above problems and choose the appropriate ratio of drugs and dosage forms to achieve better synergistic drug efficacy. For example, the nano delivery system itself has a unique size effect and is easy to be functionalized, so it can enrich the drugs in tumor sites (Huang et al., Citation2022). Xie et al. have developed a nano delivery system for the co-delivery of curcumin (CUR) and methotrexate (MTX). When exposed to an aqueous solution, the DSPE-PEG-Imine-MTX pro-drug could spontaneously self-assemble into MTX-Imine-M nanoparticles encapsulating CUR within their hydrophobic DSPE core (Xie et al., Citation2018). The MTX-Imine-M-CUR nanoparticles could release the active form of MTX and CUR more efficiently in acidic media (pH 5) and exhibited significantly stronger tumor inhibition efficiency than the combination of both free drugs in vivo. Because the properties of nanoparticles in a water environment are more easily deteriorated than in a solid environment, solvent removal has become a potential strategy to improve the storage stability of drug-loaded nanoparticles (Franze et al., Citation2018). Previously, we prepared a solid mixture film (film-injection) consisting of the drug and the loading materials which could form drug-loaded nanoparticles when added with the proper solvent. The film-injection was prepared using the solvent evaporation technique and stored in a nitrogen atmosphere, minimizing the effect of the outside environment. This novel dosage form could significantly improve the long-term storage stability of nanomedicines and could be produced on a large scale (H Liu et al., Citation2020).
3.2. Combination with other drugs
In addition to anti-tumor activities, DHM also has many activities such as antiviral, antibacterial, liver protection, neuroprotection, antioxidant and anti-inflammatory, so it can also be used in combined therapy for other diseases (Wang et al., Citation2022). For example, the ortho-trihydroxy group in the B ring of DHM is the key pharmacophore to inhibit the activity of SARS-CoV-2 3CLpro which is an important target of coronavirus diseases, thereby the combination of DHM and SARS-CoV-2 3CLpro inhibitors such as acetoxyketone provides a new strategy to treat COVID-19 (Xiao et al., Citation2021; Xiong et al., Citation2021). In addition, DHM can inhibit ROS accumulation through pGC-1α/SIRT3 signal pathway and protect cells from apoptotic cell death, thus preventing hearing loss caused by oxidative damage of auditory hair cells (Han et al., Citation2020). DHM can also reverse the reduced bioavailability of ivermectin (IVA) due to the presence of P-glycoprotein, which is an innovative therapy for alcohol use disorder and secondary alcoholic liver disease (Silva et al., Citation2021). Moreover, the use of DHM as an adjunct to BACE1 inhibitors in the treatment of Alzheimer’s disease (AD) can reduce the cross-inhibitory toxicity of BACE1 inhibitors, and can delay or reverse the AD progression (Das et al., Citation2020). In addition, DHM and ellagic acid may have a synergistic protective effect on UV-B damage of HaCaT cells and skin tissue by activating TGF-β1 and wnt signal pathways, which is the potential to protect photoaging (Moon et al., Citation2018).
In general, DHM in combination with multiple drugs may produce significant synergistic effects, as summarized in . However, the combined application of DHM was mostly for the treatment of cancer, while more studies for other diseases should be considered. In addition, so far preparations for combined therapy of DHM with other drugs are scarcely reported, which necessitates the development of dosage forms for this application. When designing the dosage forms for combined therapies, not only the action mechanism but also the physicochemical properties and administration routes of the co-delivered drugs should be considered.
Table 2. The combined applications of DHM with other drugs.
4. Some other issues to consider in the drug delivery of DHM
Due to the unstable structure of these natural products, the contact of DHM with metal ions, high temperature, and alkaline conditions should be minimized (Samsonowicz & Regulska, Citation2017). For example, the antioxidant effect of DHM depends on its phenolic hydroxyl groups in the B ring which need protection during the production, processing, transporting, and storage. It is worth mentioning that most of the current studies are about the racemes of DHM. DHM contains two chiral carbon atoms, thereby theoretically there are four optical isomers. However, the pharmacological activity of the different optical isomers is yet to verify (Zuo et al., Citation2018). In order to avoid or reduce potential side effects from the non-drug parts, we suggest that the right single optical isomer DHM should be carefully selected for future research.
The therapeutic efficacy of traditional Chinese medicine is often the comprehensive effect under the simultaneous action of multiple components. The combination of various traditional Chinese medicines fully exhibited the unique advantages of multi-system, multi-channel, and multi-target treatment strategies (Y Xie et al., Citation2021). DHM has many pharmacological activities, but it alone has low titer and unsatisfactory effects, so it can be considered as an adjuvant to other drugs in the future, which provides a wider application prospect for this natural product. However, the current research on the combination of DHM and other drugs is basically blind and inefficient. To settle this issue, we suggest using network pharmacology and high-throughput screening to purposefully find and select appropriate combination strategies for DHM (Isgut et al., Citation2018). Based on this, the pharmaceutics, feasibility, and safety of the combined application of DHM and other drugs should also be investigated.
5. Conclusion
Herein, the pharmaceutical design for DHM alone or with other drugs was reviewed. To date, various techniques have been adopted for producing all kinds of DHM preparations including nano-preparations, microemulsion, gels, crystal, and gastric floating preparation, etc., which has improved the solubility, permeability, and stability of this natural product. However, although invention patents about DHM preparations have been issued in several countries, the current transformation of DHM research results into marketable products is insufficient. The combined use of DHM with other drugs is a promising strategy to expand the application of DHM. So far, the combined applications are mostly for the treatment of cancer, while more studies are needed for the treatment of other diseases. For better synergistic effects, network pharmacology and high-throughput screening can be utilized to purposefully find and select appropriate combination strategies for DHM. Besides, preparations for combined therapy of DHM with other drugs are scarcely reported, which necessitates the development of dosage forms for this application. When designing the dosage forms for combined therapies, not only the action mechanism but also the physicochemical properties and administration routes of the co-delivered drugs should be considered. Apart from medicine, the development of DHM in the food industry is also of great potential. Due to its multiple effects and excellent safety, DHM preparations can be combined with a healthy lifestyle and developed as the ingredients in functional drinks and foods. Through this review, we hope to draw more attention from researchers in the related fields and provide valuable references for the research and development of other natural products with a similar structure-activity relationship to DHM. Although considerable time and costs are to consume in the future pharmaceutical studies of DHM, we believe that the research results of this natural compound will be gradually turned into market products with the development of new carrier materials and drug delivery systems.
Disclosure statement
The authors report no conflict of interest.
Additional information
Funding
References
- Almasi H, Jahanbakhsh Oskouie M, Saleh A. (2021). A review on techniques utilized for design of controlled release food active packaging. Crit Rev Food Sci Nutr 61:2601–21. https://doi.org/10.1080/10408398.2020.1783199.
- Aziz A, Sefidbakht Y, Rezaei S, et al. (2022). Doxorubicin-loaded, pH-sensitive albumin nanoparticles for lung cancer cell targeting. J Pharm Sci 111:1187–96. https://doi.org/10.1016/j.xphs.2021.12.006.
- Bhanushali JS, Dhiman S, Nandi U, Bharate SS. (2022). Molecular interactions of niclosamide with hydroxyethyl cellulose in binary and ternary amorphous solid dispersions for synergistic enhancement of water solubility and oral pharmacokinetics in rats. Int J Pharm 626:122144. https://doi.org/10.1016/j.ijpharm.2022.122144.
- Biswas S, Mukherjee PK, Harwansh RK, et al. (2019). Enhanced bioavailability and hepatoprotectivity of optimized ursolic acid-phospholipid complex. Drug Dev Ind Pharm 45:946–58. https://doi.org/10.1080/03639045.2019.1583755.
- Bombardelli E. (1991). Phytosome: new cosmetic delivery system. Boll Chim Farm 130:431–8. https://www.ncbi.nlm.nih.gov/pubmed/1809296.
- Cai S, Zhang P, Zhou L, et al. (2016). Determination and correlation of the solubility of myricetin in ethanol and water mixtures from 288.15 to 323.15K. Phys Chem Liq 55:1–11. https://doi.org/10.1080/00319104.2016.1163560.
- Chaurasiya A, Gorajiya A, Panchal K, et al. (2022). A review on multivesicular liposomes for pharmaceutical applications: preparation, characterization, and translational challenges. Drug Deliv Transl Res 12:1569–87. https://doi.org/10.1007/s13346-021-01060-y.
- ChavesJrJV, Dos Santos JAB, Lins TB, et al. (2020). A new ferulic acid-nicotinamide cocrystal with improved solubility and dissolution performance. J Pharm Sci 109:1330–7. https://doi.org/10.1016/j.xphs.2019.12.002.
- Chen J, Wang X, Xia T, et al. (2021). Molecular mechanisms and therapeutic implications of dihydromyricetin in liver disease. Biomed Pharmacother 142:111927. https://doi.org/10.1016/j.biopha.2021.111927.
- Chen L, Shi M, Lv C, et al. (2021). Dihydromyricetin acts as a potential redox balance mediator in cancer chemoprevention. Mediators Inflamm 2021:6692579. https://doi.org/10.1155/2021/6692579.
- Chen Y, Luo HQ, Sun LL, et al. (2018). Dihydromyricetin attenuates myocardial hypertrophy induced by transverse aortic constriction via oxidative stress inhibition and SIRT3 pathway enhancement. IJMS 19:2592. https://doi.org/10.3390/ijms19092592.
- Cheng X, Yan H, Pang S, et al. (2022). Liposomes as multifunctional nano-carriers for medicinal natural products. Front Chem 10:963004. https://doi.org/10.3389/fchem.2022.963004.
- Cid AG, Simonazzi A, Palma SD, Bermúdez JM. (2019). Solid dispersion technology as a strategy to improve the bioavailability of poorly soluble drugs. Ther Deliv 10:363–82. https://doi.org/10.4155/tde-2019-0007.
- Cid-Samamed A, Rakmai J, Mejuto JC, et al. (2022). Cyclodextrins inclusion complex: preparation methods, analytical techniques and food industry applications. Food Chem 384:132467. https://doi.org/10.1016/j.foodchem.2022.132467.
- Dalcin AJF, Roggia I, Felin S, et al. (2021). UVB photoprotective capacity of hydrogels containing dihydromyricetin nanocapsules to UV-induced DNA damage. Colloids Surf B Biointerfaces 197:111431. https://doi.org/10.1016/j.colsurfb.2020.111431.
- Dalcin AJF, Santos CG, Gundel SS, et al. (2017). Anti biofilm effect of dihydromyricetin-loaded nanocapsules on urinary catheter infected by Pseudomonas aeruginosa. Colloids Surf B Biointerfaces 156:282–91. https://doi.org/10.1016/j.colsurfb.2017.05.029.
- Dalcin AJF, Vizzotto BS, Bochi GV, et al. (2019). Nanoencapsulation of the flavonoid dihydromyricetin protects against the genotoxicity and cytotoxicity induced by cationic nanocapsules. Colloids Surf B Biointerfaces 173:798–805. https://doi.org/10.1016/j.colsurfb.2018.10.066.
- Dams ET, Laverman P, Oyen WJ, et al. (2000). Accelerated blood clearance and altered biodistribution of repeated injections of sterically stabilized liposomes. J Pharmacol Exp Ther 292:1071–9.
- Das S, Majumder T, Sarkar A, et al. (2020). Flavonoids as BACE1 inhibitors: QSAR modelling, screening and in vitro evaluation. Int J Biol Macromol 165:1323–30. https://doi.org/10.1016/j.ijbiomac.2020.09.232.
- Deng S, Gigliobianco MR, Censi R, Di Martino P. (2020). Polymeric banocapsules as banotechnological alternative for drug delivery system: current status, challenges and opportunities. Nanomaterials 10:847. https://doi.org/10.3390/nano10050847.
- Egito EST, Amaral-Machado L, Alencar EN, Oliveira AG. (2021). Microemulsion systems: from the design and architecture to the building of a new delivery system for multiple-route drug delivery. Drug Deliv Transl Res 11:2108–33. https://doi.org/10.1007/s13346-020-00872-8.
- El Sayed MM, Takata H, Shimizu T, et al. (2020). Hepatosplenic phagocytic cells indirectly contribute to anti-PEG IgM production in the accelerated blood clearance (ABC) phenomenon against PEGylated liposomes: appearance of an unexplained mechanism in the ABC phenomenon. J Control Release 323:102–9. https://doi.org/10.1016/j.jconrel.2020.04.011.
- Fan KJ, Yang B, Liu Y, et al. (2017). Inhibition of human lung cancer proliferation through targeting stromal fibroblasts by dihydromyricetin. Mol Med Rep 16:9758–62. https://doi.org/10.3892/mmr.2017.7802.
- Fan L, Tong Q, Dong W, et al. (2017). Tissue distribution, excretion, and metabolic profile of dihydromyricetin, a flavonoid from vine tea (Ampelopsis grossedentata) after oral administration in rats. J Agric Food Chem 65:4597–604. https://doi.org/10.1021/acs.jafc.7b01155.
- Fan M, Zhang G, Pan J, Gong D. (2017). An inhibition mechanism of dihydromyricetin on tyrosinase and the joint effects of vitamins B6, D3 or E. Food Funct 8:2601–10. https://doi.org/10.1039/c7fo00236j.
- Ferte J, Kuhnel JM, Chapuis G, et al. (1999). Flavonoid-related modulators of multidrug resistance: synthesis, pharmacological activity, and structure-activity relationships. J Med Chem 42:478–89. https://doi.org/10.1021/jm981064b.
- Frank LA, Contri RV, Beck RC, et al. (2015). Improving drug biological effects by encapsulation into polymeric nanocapsules. Wiley Interdiscip Rev Nanomed Nanobiotechnol 7:623–39. https://doi.org/10.1002/wnan.1334.
- Franze S, Selmin F, Samaritani E, et al. (2018). Lyophilization of liposomal formulations: still necessary, still challenging. Pharmaceutics 10:139. https://doi.org/10.3390/pharmaceutics10030139.
- Gao J, Shi N, Guo H, et al. (2021). UPLC-Q-TOF/MS-based metabolomics approach to reveal the hepatotoxicity of emodin and detoxification of dihydromyricetin. ACS Omega 6:5348–58. https://doi.org/10.1021/acsomega.0c05488.
- Garavand F, Jalai-Jivan M, Assadpour E, Jafari SM. (2021). Encapsulation of phenolic compounds within nano/microemulsion systems: a review. Food Chem 364:130376. https://doi.org/10.1016/j.foodchem.2021.130376.
- Geng S, Jiang Z, Ma H, et al. (2021). Fabrication and characterization of novel edible Pickering emulsion gels stabilized by dihydromyricetin. Food Chem 343:128486. https://doi.org/10.1016/j.foodchem.2020.128486.
- Geng S, Li Y, Lv J, et al. (2022). Fabrication of food-grade Pickering high internal phase emulsions (HIPEs) stabilized by a dihydromyricetin and lysozyme mixture. Food Chem 373:131576. https://doi.org/10.1016/j.foodchem.2021.131576.
- Geng S, Liu X, Ma H, et al. (2021). Multi-scale stabilization mechanism of Pickering emulsion gels based on dihydromyricetin/high-amylose corn starch composite particles. Food Chem 355:129660. https://doi.org/10.1016/j.foodchem.2021.129660.
- Gulcin I. (2020). Antioxidants and antioxidant methods: an updated overview. Arch Toxicol 94:651–715. https://doi.org/10.1007/s00204-020-02689-3.
- Guo RX, Fu X, Chen J, et al. (2016). Preparation and characterization of microemulsions of myricetin for improving its antiproliferative and antioxidative activities and Oral bioavailability. J Agric Food Chem 64:6286–94. https://doi.org/10.1021/acs.jafc.6b02184.
- Han H, Dong Y, Ma X. (2020). Dihydromyricetin protects against gentamicin-induced ototoxicity via PGC-1alpha/SIRT3 signaling in vitro. Front Cell Dev Biol 8:702. https://doi.org/10.3389/fcell.2020.00702.
- He MH, Zhang Q, Shu G, et al. (2018). Dihydromyricetin sensitizes human acute myeloid leukemia cells to retinoic acid-induced myeloid differentiation by activating STAT1. Biochem Biophys Res Commun 495:1702–7. https://doi.org/10.1016/j.bbrc.2017.12.030.
- Hu Q, Zhang T, Yi L, et al. (2018). Dihydromyricetin inhibits NLRP3 inflammasome-dependent pyroptosis by activating the Nrf2 signaling pathway in vascular endothelial cells. Biofactors 44:123–36. https://doi.org/10.1002/biof.1395.
- Huang Y, Wang T, Yang J, et al. (2022). Current strategies for the treatment of hepatocellular carcinoma by modulating the tumor microenvironment via nano-delivery systems: a review. Int J Nanomed 17:2335–52. https://doi.org/10.2147/IJN.S363456.
- Ibaraki H, Takeda A, Arima N, et al. (2021). In vivo fluorescence imaging of passive inflammation site accumulation of liposomes via intravenous administration focused on their surface charge and PEG modification. Pharmaceutics 13:104. https://doi.org/10.3390/pharmaceutics13010104.
- Iglesias N, Galbis E, Romero-Azogil L, et al. (2020). In-depth study into polymeric materials in low-density gastroretentive formulations. Pharmaceutics 12:636. https://doi.org/10.3390/pharmaceutics12070636.
- Isgut M, Rao M, Yang C, et al. (2018). Application of combination high-throughput phenotypic screening and target identification methods for the discovery of natural product-based combination drugs. Med Res Rev 38:504–24. https://doi.org/10.1002/med.21444.
- Jiang L, Zhang Q, Ren H, et al. (2015). Dihydromyricetin enhances the chemo-sensitivity of nedaplatin via regulation of the p53/Bcl-2 pathway in hepatocellular carcinoma cells. PLoS One 10:e0124994. https://doi.org/10.1371/journal.pone.0124994.
- Kavanagh ON, Croker DM, Walker GM, Zaworotko MJ. (2019). Pharmaceutical cocrystals: from serendipity to design to application. Drug Discov Today 24:796–804. https://doi.org/10.1016/j.drudis.2018.11.023.
- Kianfar E. (2021). Protein nanoparticles in drug delivery: animal protein, plant proteins and protein cages, albumin nanoparticles. J Nanobiotechnol 19:159. https://doi.org/10.1186/s12951-021-00896-3.
- Kim DW, Kwon MS, Yousaf AM, et al. (2014). Comparison of a solid SMEDDS and solid dispersion for enhanced stability and bioavailability of clopidogrel napadisilate. Carbohydr Polym 114:365–74. https://doi.org/10.1016/j.carbpol.2014.08.034.
- Kou X, Liu X, Chen X, et al. (2016). Ampelopsin attenuates brain aging of D-gal-induced rats through miR-34a-mediated SIRT1/mTOR signal pathway. Oncotarget 7:74484–95. https://doi.org/10.18632/oncotarget.[PMC][27780933]
- Kuche K, Bhargavi N, Dora CP, Jain S. (2019). Drug-phospholipid complex-a go through strategy for enhanced oral bioavailability. AAPS PharmSciTech 20:43. https://doi.org/10.1208/s12249-018-1252-4.
- Kunde SS, Wairkar S. (2022). Targeted delivery of albumin nanoparticles for breast cancer: a review. Colloids Surf B Biointerfaces 213:112422. https://doi.org/10.1016/j.colsurfb.2022.112422.
- Landis HE, Getachew B, Tizabi Y. (2022). Therapeutic potential of flavonoids and Zinc in COVID-19. Medpress Nutr Food Sci 1:202111001.
- Li P, Ramaiah T, Zhang M, et al. (2020). Two cocrystals of berberine chloride with myricetin and dihydromyricetin: crystal structures, characterization, and antitumor activities. Cryst Growth Des 20:157–66. https://doi.org/10.1021/acs.cgd.9b00939.
- Li S, Zhang B, Li C, et al. (2020). Pickering emulsion gel stabilized by octenylsuccinate quinoa starch granule as lutein carrier: role of the gel network. Food Chem 305:125476. https://doi.org/10.1016/j.foodchem.2019.125476.
- Li X, Wang X, Wang B, et al. (2022). Dihydromyricetin protects against Doxorubicin-induced cardiotoxicity through activation of AMPK/mTOR pathway. Phytomedicine 99:154027. https://doi.org/10.1016/j.phymed.2022.154027.
- Li XM, Li X, Wu Z, et al. (2020). Chitosan hydrochloride/carboxymethyl starch complex nanogels stabilized Pickering emulsions for oral delivery of beta-carotene: protection effect and in vitro digestion study. Food Chem 315:126288. https://doi.org/10.1016/j.foodchem.2020.126288.
- Li Y, Zhang R, Li X, et al. (2020). The preparation of dexamethasone sodium phosphate multivesicular liposomes thermosensative hydrogel and its impact on noise-induced hearing loss in the Guinea pigs. Exp Cell Res 387:111755. https://doi.org/10.1016/j.yexcr.2019.111755.
- Li Y, Zhou Y, Wang M, et al. (2021). Ampelopsin inhibits breast cancer cell growth through mitochondrial apoptosis pathway. Biol Pharm Bull 44:1738–45. https://doi.org/10.1248/bpb.b21-00470.
- Liang H, He K, Li T, et al. (2020). Mechanism and antibacterial activity of vine tea extract and dihydromyricetin against Staphylococcus aureus. Sci Rep 10:21416. https://doi.org/10.1038/s41598-020-78379-y.
- Lim SI, Lukianov CI, Champion JA. (2017). Self-assembled protein nanocarrier for intracellular delivery of antibody. J Control Release 249:1–10. https://doi.org/10.1016/j.jconrel.2017.01.007.
- Lima AL, Gratieri T, Cunha-Filho M, Gelfuso GM. (2022). Polymeric nanocapsules: a review on design and production methods for pharmaceutical purpose. Methods 199:54–66. https://doi.org/10.1016/j.ymeth.2021.07.009.
- Liu B, Ma Y, Yuan C, et al. (2012). Characterization, stability and antioxidant activity of the inclusion complex of dihydromyricetin with hydroxypropyl-β-cyclodextrin. J Food Biochem 36:634–41. https://doi.org/10.1111/j.1745-4514.2011.00577.x.
- Liu C, Ma X, Zhuang J, et al. (2020). Cardiotoxicity of doxorubicin-based cancer treatment: what is the protective cognition that phytochemicals provide us? Pharmacol Res 160:105062. https://doi.org/10.1016/j.phrs.2020.105062.
- Liu D, Mao YQ, Ding LJ, Zeng XA. (2019). Dihydromyricetin: a review on identification and quantification methods, biological activities, chemical stability, metabolism and approaches to enhance its bioavailability. Trends Food Sci Technol 91:586–97. https://doi.org/10.1016/j.tifs.2019.07.038.
- Liu H, Gan C, Shi H, et al. (2021). Gastric floating pill enhances the bioavailability and drug efficacy of dihydromyricetin in vivo. J Drug Delivery Sci Technol 61:102279. https://doi.org/10.1016/j.jddst.2020.102279.
- Liu H, Wang D, Ren Y, et al. (2022). Multispectroscopic and synergistic antioxidant study on the combined binding of caffeic acid and (−)-epicatechin gallate to lysozyme, Spectrochim. Spectrochim Acta A Mol Biomol Spectrosc 272:120986. https://doi.org/10.1016/j.saa.2022.120986.
- Liu H, Wang S, Shi H, et al. (2021). Gastric floating tablet improves the bioavailability and reduces the hypokalemia effect of gossypol in vivo. Saudi Pharm J 29:305–14. https://doi.org/10.1016/j.jsps.2021.03.001.
- Liu H, Zhang R, Zhang D, et al. (2022). Cyclic RGD-decorated liposomal gossypol AT-101 targeting for enhanced antitumor effect. Int J Nanomed 17:227–44. https://doi.org/10.2147/IJN.S341824.
- Liu H, Zhang YH, Hu MH, et al. (2020). Film-injection as a dosage form for etomidate: enhancing the stability of nanomedicines using solid intermediate products. J Drug Delivery Sci Technol 56:101541. https://doi.org/10.1016/j.jddst.2020.101541.
- Liu H, Zhao W, Hu Q, et al. (2019). Gastric floating sustained-release tablet for dihydromyricetin: development, characterization, and pharmacokinetics study. Saudi Pharm J 27:1000–8. https://doi.org/10.1016/j.jsps.2019.08.002.
- Liu J, Wang X, Yong H, et al. (2018). Recent advances in flavonoid-grafted polysaccharides: synthesis, structural characterization, bioactivities and potential applications. Int J Biol Macromol 116:1011–25. https://doi.org/10.1016/j.ijbiomac.2018.05.149.
- Liu J, Yong H, Liu Y, Bai R. (2020). Recent advances in the preparation, structural characteristics, biological properties and applications of gallic acid grafted polysaccharides. Int J Biol Macromol 156:1539–55. https://doi.org/10.1016/10.1016/j.ijbiomac.2019.11.202.
- Liu K, Chen YY, Zha XQ, et al. (2021). Research progress on polysaccharide/protein hydrogels: preparation method, functional property and application as delivery systems for bioactive ingredients. Food Res Int 147:110542. https://doi.org/10.1016/j.foodres.2021.110542.
- Liu L, Li Y, Zhang M, et al. (2022). A drug-drug cocrystal of dihydromyricetin and pentoxifylline. J Pharm Sci 111:82–7. https://doi.org/10.1016/j.xphs.2021.06.021.
- Liu S, Ai Q, Feng K, et al. (2016). The cardioprotective effect of dihydromyricetin prevents ischemia-reperfusion-induced apoptosis in vivo and in vitro via the PI3K/Akt and HIF-1alpha signaling pathways. Apoptosis 21:1366–85. https://doi.org/10.1007/s10495-016-1306-6.
- Lu B, Ma Q, Zhang J, et al. (2021). Preparation and characterization of bupivacaine multivesicular liposome: a QbD study about the effects of formulation and process on critical quality attributes. Int J Pharm 598:120335. https://doi.org/10.1016/j.ijpharm.2021.120335.
- Luo F, Zeng D, Chen R, et al. (2021). PEGylated dihydromyricetin-loaded nanoliposomes coated with tea saponin inhibit bacterial oxidative respiration and energy metabolism. Food Funct 12:9007–17. https://doi.org/10.1039/d1fo01943k.
- Luo F, Zeng DD, Yang YT, et al. (2021). Preparation, characterization and antibacterial properties of multivesicular dihydromyricetin-coated liposomes. CIESC J 72:2223–32. (In Chinese). https://doi.org/10.11949/0438-1157.20201159.
- Lv P, Wang D, Dai L, et al. (2020). Pickering emulsion gels stabilized by high hydrostatic pressure-induced whey protein isolate gel particles: characterization and encapsulation of curcumin. Food Res Int 132:109032. https://doi.org/10.1016/j.foodres.2020.109032.
- Lv S, Kim H, Song Z, et al. (2020). Unimolecular polypeptide micelles via ultrafast polymerization of N-carboxyanhydrides. J Am Chem Soc 142:8570–4. https://doi.org/10.1021/jacs.0c01173.
- Ma JQ, Sun YZ, Ming QL, et al. (2019). Ampelopsin attenuates carbon tetrachloride-induced mouse liver fibrosis and hepatic stellate cell activation associated with the SIRT1/TGF-beta1/Smad3 and autophagy pathway. Int Immunopharmacol 77:105984. https://doi.org/10.1016/j.intimp.2019.105984.
- Ma X, Bai S, Zhang X, et al. (2019). Enhanced tumor penetration and chemotherapy efficiency by covalent self-assembled nanomicelle responsive to tumor microenvironment. Biomacromolecules 20:2637–48. https://doi.org/10.1021/acs.biomac.9b00424.
- Ma Y, Zeng M, Sun R, Hu M. (2014). Disposition of flavonoids impacts their efficacy and safety. Curr Drug Metab 15:841–64. https://doi.org/10.2174/1389200216666150206123719.
- Manzoor A, Dar AH, Pandey VK, et al. (2022). Recent insights into polysaccharide-based hydrogels and their potential applications in food sector: a review. Int J Biol Macromol 213:987–1006. https://doi.org/10.1016/j.ijbiomac.2022.06.044.
- Martínez-Coria H, Mendoza-Rojas MX, Arrieta-Cruz I, López-Valdés HE. (2019). Preclinical research of dihydromyricetin for brain aging and neurodegenerative diseases. Front Pharmacol 10:1334. https://doi.org/10.3389/fphar.2019.01334.
- Moon NR, Kang S, Park S. (2018). Consumption of ellagic acid and dihydromyricetin synergistically protects against UV-B induced photoaging, possibly by activating both TGF-beta1 and wnt signaling pathways. J Photochem Photobiol B 178:92–100. https://doi.org/10.1016/j.jphotobiol.2017.11.004.
- Morales P, Maieves HA, Dias MI, et al. (2017). Hovenia dulcis Thunb. pseudofruits as functional foods: phytochemicals and bioactive properties in different maturity stages. J Funct Foods 29:37–45. https://doi.org/10.1016/j.jff.2016.12.003.
- Mu K, Jiang K, Wang Y, et al. (2022). The biological fate of pharmaceutical excipient beta-cyclodextrin: pharmacokinetics, tissue distribution, excretion, and metabolism of beta-cyclodextrin in rats. Molecules 27:1138. https://doi.org/10.3390/molecules27031138.
- Mwangi WW, Lim HP, Low LE, et al. (2020). Food-grade Pickering emulsions for encapsulation and delivery of bioactives. Trends Food Sci Technol 100:320–32. https://doi.org/10.1016/j.tifs.2020.04.020.
- Nair AR, Lakshman YD, Anand VSK, et al. (2020). Overview of extensively employed polymeric carriers in solid dispersion technology. AAPS PharmSciTech 21:309. https://doi.org/10.1208/s12249-020-01849-z.
- Narayanaswamy R, Torchilin VP. (2019). Hydrogels and their applications in targeted drug delivery. Molecules 24:603. https://doi.org/10.3390/molecules24030603.
- Nikezic AVV, Bondzic AM, Vasic VM. (2020). Drug delivery systems based on nanoparticles and related nanostructures. Eur J Pharm Sci 151:105412. https://doi.org/10.1016/j.ejps.2020.105412.
- Park GB, Jeong JY, Kim D. (2017). Ampelopsin-induced reactive oxygen species enhance the apoptosis of colon cancer cells by activating endoplasmic reticulum stress-mediated AMPK/MAPK/XAF1 signaling. Oncol Lett 14:7947–56. https://doi.org/10.3892/ol.2017.7255.[PMC][29250183]
- Rajora A, Nagpal K. (2022). A critical review on floating tablets as a tool for achieving better gastric retention. Crit Rev Ther Drug Carrier Syst 39:65–103. https://doi.org/10.1615/CritRevTherDrugCarrierSyst.2021038568.
- Ruan LP, Yu BY, Fu GM, Zhu DN. (2005). Improving the solubility of ampelopsin by solid dispersions and inclusion complexes. J Pharm Biomed Anal 38:457–64. https://doi.org/10.1016/j.jpba.2005.01.030.
- Salawi A. (2022). Self-emulsifying drug delivery systems: a novel approach to deliver drugs. Drug Deliv 29:1811–23. https://doi.org/10.1080/10717544.2022.2083724.
- Samsonowicz M, Regulska E. (2017). Spectroscopic study of molecular structure, antioxidant activity and biological effects of metal hydroxyflavonol complexes. Spectrochim Acta A Mol Biomol Spectrosc 173:757–71. https://doi.org/10.1016/j.saa.2016.10.031.
- Santana R, Zuluaga R, Ganan P, et al. (2020). PTML model for selection of nanoparticles, anticancer drugs, and vitamins in the design of drug-vitamin nanoparticle release systems for cancer cotherapy. Mol Pharm 17:2612–27. https://doi.org/10.1021/acs.molpharmaceut.0c00308.
- Shen C, Chen R, Qian Z, et al. (2015). Intestinal absorption mechanisms of MTBH, a novel hesperetin derivative, in Caco-2 cells, and potential involvement of monocarboxylate transporter 1 and multidrug resistance protein 2. Eur J Pharm Sci 78:214–24. https://doi.org/10.1016/j.ejps.2015.07.022.
- Shen J, Li J, Yu P, Du G. (2022). Research status and hotspots of anticancer natural products based on the patent literature and scientific articles. Front Pharmacol 13:903239. https://doi.org/10.3389/fphar.2022.903239.
- Shen P, Tang Q, Chen X, Li Z. (2022). Nanocrystalline cellulose extracted from bast fibers: preparation, characterization, and application. Carbohydr Polym 290:119462. https://doi.org/10.1016/j.carbpol.2022.119462.
- Shi C, Wang J, Zhang R, et al. (2022). Dihydromyricetin alleviates Escherichia coli lipopolysaccharide-induced hepatic injury in chickens by inhibiting the NLRP3 inflammasome. Vet Res 53:6. https://doi.org/10.1186/s13567-022-01024-1.
- Shinozaki T, Ono M, Higashi K, Moribe K. (2019). A novel drug-drug cocrystal of levofloxacin and metacetamol: reduced hygroscopicity and improved photostability of levofloxacin. J Pharm Sci 108:2383–90. https://doi.org/10.1016/j.xphs.2019.02.014.
- Silva J, Carry E, Xue C, et al. (2021). A novel dual drug approach that combines ivermectin and dihydromyricetin (DHM) to reduce alcohol drinking and preference in mice. Molecules 26:1791. https://doi.org/10.3390/molecules26061791.
- Solanki SS, Sarkar B, Dhanwani RK. (2012). Microemulsion drug delivery system: for bioavailability enhancement of ampelopsin. ISRN Pharm 2012:108164. https://doi.org/10.5402/2012/108164.
- Stankovic JSK, Selakovic D, Mihailovic V, Rosic G. (2020). Antioxidant supplementation in the treatment of neurotoxicity induced by platinum-based chemotherapeutics: a review. IJMS 21:7753. https://doi.org/10.3390/ijms21207753.
- Stecanella LA, Bitencourt APR, Vaz GR, et al. (2021). Glycyrrhizic acid and its hydrolyzed metabolite 18 beta-glycyrrhetinic acid as specific ligands for targeting nanosystems in the treatment of liver cancer. Pharmaceutics 13:1792. https://doi.org/10.3390/pharmaceutics13111792.
- Sun C, Gui Y, Hu R, et al. (2018). Preparation and pharmacokinetics evaluation of solid self-microemulsifying drug delivery system (S-SMEDDS) of osthole. AAPS PharmSciTech 19:2301–10. https://doi.org/10.1208/s12249-018-1067-3.
- Sun CC, Li Y, Yin ZP, Zhang QF. (2021). Physicochemical properties of dihydromyricetin and the effects of ascorbic acid on its stability and bioavailability. J Sci Food Agric 101:3862–9. https://doi.org/10.1002/jsfa.11022.
- Sun CC, Su H, Zheng GD, et al. (2020). Fabrication and characterization of dihydromyricetin encapsulated zein-caseinate nanoparticles and its bioavailability in rat. Food Chem 330:127245. https://doi.org/10.1016/j.foodchem.2020.127245.
- Sun Y, Li Y, Shen Y, et al. (2019). Enhanced oral delivery and anti-gastroesophageal reflux activity of curcumin by binary mixed micelles. Drug Dev Ind Pharm 45:1444–50. https://doi.org/10.1080/03639045.2019.1628041.
- Sun Y, Liu S, Yang S, et al. (2021). Mechanism of dihydromyricetin on inflammatory diseases. Front Pharmacol 12:794563. https://doi.org/10.3389/fphar.2021.794563.
- Sun Y, Liu W, Wang C, et al. (2019). Combination of dihydromyricetin and ondansetron strengthens antiproliferative efficiency of adriamycin in K562/ADR through downregulation of SORCIN: a new strategy of inhibiting P-glycoprotein. J Cell Physiol 234:3685–96. https://doi.org/10.1002/jcp.27141
- Sun Y, Wang C, Meng Q, et al. (2018). Targeting P-glycoprotein and SORCIN: dihydromyricetin strengthens anti-proliferative efficiency of adriamycin via MAPK/ERK and Ca2+-mediated apoptosis pathways in MCF-7/ADR and K562/ADR. J Cell Physiol 233:3066–79. https://doi.org/10.1002/jcp.26087.
- Sun Z, Lu W, Lin N, et al. (2020). Dihydromyricetin alleviates doxorubicin-induced cardiotoxicity by inhibiting NLRP3 inflammasome through activation of SIRT1. Biochem Pharmacol 175:113888. https://doi.org/10.1016/j.bcp.2020.113888.
- Tian Y, Sang H, Liu M, et al. (2020). Dihydromyricetin is a new inhibitor of influenza polymerase PB2 subunit and influenza-induced inflammation. Microbes Infect 22:254–62. https://doi.org/10.1016/j.micinf.2020.05.021.
- Tong H, Zhang X, Tan L, et al. (2020). Multitarget and promising role of dihydromyricetin in the treatment of metabolic diseases. Eur J Pharmacol 870:172888. https://doi.org/10.1016/j.ejphar.2019.172888.
- Tong Q, Hou X, Fang J, et al. (2015). Determination of dihydromyricetin in rat plasma by LC-MS/MS and its application to a pharmacokinetic study. J Pharm Biomed Anal 114:455–61. https://doi.org/10.1016/j.jpba.2015.06.030.
- Tran P, Park JS. (2021). Application of supercritical fluid technology for solid dispersion to enhance solubility and bioavailability of poorly water-soluble drugs. Int J Pharm 610:121247. https://doi.org/10.1016/j.ijpharm.2021.121247.
- Wang C, Tong Q, Hou X, et al. (2016). Enhancing bioavailability of dihydromyricetin through inhibiting precipitation of soluble cocrystals by a crystallization inhibitor. Cryst Growth Des 16:5030–9. https://doi.org/10.1021/acs.cgd.6b00591
- Wang C, Xiong W, Reddy Perumalla S, et al. (2016). Solid-state characterization of optically pure (+)Dihydromyricetin extracted from Ampelopsis grossedentata leaves. Int J Pharm 511:245–52. https://doi.org/10.1016/j.ijpharm.2016.07.018.
- Wang D, Ma Y, Wang Q, et al. (2019). Solid self-emulsifying delivery system (S-SEDS) of dihydromyricetin: a new way for preparing functional food. J Food Sci 84:936–45. https://doi.org/10.1111/1750-3841.14508.
- Wang S, Ge F, Cai T, et al. (2021). Dihydromyricetin inhibits proliferation and migration of gastric cancer cells through regulating Akt/STAT3 signaling pathways and HMGB1 expression. Nan Fang Yi Ke Da Xue Xue Bao 41:87–92. (In Chinese). https://doi.org/10.12122/j.issn.1673-4254.2021.01.12.
- Wang Y, Wang J, Xiang H, et al. (2022). Recent update on application of dihydromyricetin in metabolic related diseases. Biomed Pharmacother 148:112771. https://doi.org/10.1016/j.biopha.2022.112771.
- Wang Z, Sun X, Feng Y, et al. (2017). Dihydromyricetin reverses MRP2-mediated MDR and enhances anticancer activity induced by oxaliplatin in colorectal cancer cells. Anticancer Drugs 28:281–8. https://doi.org/10.1097/CAD.0000000000000459.
- Wei C, Chen X, Chen D, et al. (2022). Dihydromyricetin enhances intestinal antioxidant capacity of growing-finishing pigs by activating ERK/Nrf2/HO-1 signaling pathway. Antioxidants 11:704. https://doi.org/10.3390/antiox11040704.
- Wei Z, Chen Y, Wijaya W, et al. (2020). Hydrogels assembled from ovotransferrin fibrils and xanthan gum as dihydromyricetin delivery vehicles. Food Funct 11:1478–88. https://doi.org/10.1039/c9fo02564b.
- Wu F, Li Y, Song H, et al. (2016). Preventive effect of dihydromyricetin against cisplatin-induced nephrotoxicity in vitro and in vivo. Evid Based Complement Alternat Med 2016:7937385. https://doi.org/10.1155/2016/7937385.
- Wu J, Xiao Z, Li H, et al. (2022). Present status, challenges, and prospects of dihydromyricetin in the battle against cancer. Cancers (Basel) 14:3487. https://doi.org/10.3390/cancers14143487.
- Wu JZ, Ardah M, Haikal C, et al. (2019). Dihydromyricetin and Salvianolic acid B inhibit alpha-synuclein aggregation and enhance chaperone-mediated autophagy. Transl Neurodegener 8:18. https://doi.org/10.1186/s40035-019-0159-7.
- Wu M, Jiang M, Dong T, et al. (2020). Reversal effect of dihydromyricetin on multiple drug resistance in SGC7901/5-FU cells. Asian Pac J Cancer Prev 21:1269–74. https://doi.org/10.31557/APJCP.2020.21.5.1269.
- Wu YP, Xiao Y, Yue YX, et al. (2020). A deep insight into mechanism for inclusion of 2R,3R-dihydromyricetin with cyclodextrins and the effect of complexation on antioxidant and lipid-lowering activities. Food Hydrocolloids 103:105718. https://doi.org/10.1016/j.foodhyd.2020.105718.
- Xiao T, Wei Y, Cui M, et al. (2021). Effect of dihydromyricetin on SARS-CoV-2 viral replication and pulmonary inflammation and fibrosis. Phytomedicine 91:153704. https://doi.org/10.1016/j.phymed.2021.153704.
- Xie J, Fan Z, Li Y, et al. (2018). Design of pH-sensitive methotrexate prodrug-targeted curcumin nanoparticles for efficient dual-drug delivery and combination cancer therapy. Int J Nanomed 13:1381–98. https://doi.org/10.2147/IJN.S152312.
- Xie J, Liu J, Chen TM, et al. (2015). Dihydromyricetin alleviates carbon tetrachloride-induced acute liver injury via JNK-dependent mechanism in mice. WJG 21:5473–81. https://doi.org/10.3748/wjg.v21.i18.5473.
- Xie W, Du Y, Yuan S, Pang J. (2021). Dihydromyricetin incorporated active films based on konjac glucomannan and gellan gum. Int J Biol Macromol 180:385–91. https://doi.org/10.1016/j.ijbiomac.2021.02.185.
- Xie Y, Mai CT, Zheng DC, et al. (2021). Wutou decoction ameliorates experimental rheumatoid arthritis via regulating NF-kB and Nrf2: integrating efficacy-oriented compatibility of traditional Chinese medicine. Phytomedicine 85:153522. https://doi.org/10.1016/j.phymed.2021.153522.
- Xiong Y, Zhu GH, Zhang YN, et al. (2021). Flavonoids in Ampelopsis grossedentata as covalent inhibitors of SARS-CoV-2 3CLpro: inhibition potentials, covalent binding sites and inhibitory mechanisms. Int J Biol Macromol 187:976–87. https://doi.org/10.1016/j.ijbiomac.2021.07.167.
- Xu Y, Wang S, Chan HF, et al. (2017). Dihydromyricetin induces apoptosis and reverses drug resistance in ovarian cancer cells by p53-mediated downregulation of survivin. Sci Rep 7:46060. https://doi.org/10.1038/srep46060.
- Yan Y, Wang K, Tang X, et al. (2019). Phytochemicals protect L02 cells against hepatotoxicity induced by emodin via the Nrf2 signaling pathway. Toxicol Res (Camb) 8:1028–34. https://doi.org/10.1039/c9tx00220k.
- Yang J, Liu B, Liu F, Zhang Y. (2011). Apoptosis induced by the inclusion complex of dihydromyricetin with hydroxypropyl-β-cyclodextrin in Human Hep G2 cells. J Med Plants Res 5:114–8. https://doi.org/10.5897/JMPR.9000249.
- Ye J, Bao S, Zhao S, et al. (2021). Self-assembled micelles improve the oral bioavailability of dihydromyricetin and anti-acute alcoholism activity. AAPS PharmSciTech 22:111. https://doi.org/10.1208/s12249-021-01983-2.
- Yuba E. (2020). Development of functional liposomes by modification of stimuli-responsive materials and their biomedical applications. J Mater Chem B 8:1093–107. https://doi.org/10.1039/c9tb02470k.
- Zeng X, Yang J, Hu O, et al. (2019). Dihydromyricetin ameliorates nonalcoholic fatty liver disease by improving mitochondrial respiratory capacity and redox homeostasis through modulation of SIRT3 signaling. Antioxid Redox Signal 30:163–83. https://doi.org/10.1089/ars.2017.7172.
- Zhang C, Xu C, Gao X, Yao Q. (2022). Platinum-based drugs for cancer therapy and anti-tumor strategies. Theranostics 12:2115–32. https://doi.org/10.7150/thno.69424.
- Zhang J, Chen Y, Luo H, et al. (2018). Recent update on the pharmacological effects and mechanisms of dihydromyricetin. Front Pharmacol 9:1204. https://doi.org/10.3389/fphar.2018.01204.
- Zhang Q, Liu J, Duan H, et al. (2021). Activation of Nrf2/HO-1 signaling: an important molecular mechanism of herbal medicine in the treatment of atherosclerosis via the protection of vascular endothelial cells from oxidative stress. J Adv Res 34:43–63. https://doi.org/10.1016/j.jare.2021.06.023.
- Zhang WJ, Chen YZ, Tang LR, et al. (2018). Preparation of long-circulating dihydromyricetin liposomes and its pharmacokinetics in rats. Zhong Cao Yao 49:806–13. (In Chinese). https://doi.org/10.7501/j.issn.0253-2670.2018.04.009.
- Zhang Y, Lu H, Wang B, et al. (2020). pH-responsive non-Pickering emulsion stabilized by dynamic covalent bond surfactants and nano-SiO2 particles. Langmuir 36:15230–9. https://doi.org/10.1021/acs.langmuir.0c02422.
- Zhang Z, Zhang H, Chen S, et al. (2017). Dihydromyricetin induces mitochondria-mediated apoptosis in HepG2 cells through down-regulation of the Akt/Bad pathway. Nutr Res 38:27–33. https://doi.org/10.1016/j.nutres.2017.01.003.
- Zhao X, Shi C, Zhou X, et al. (2019). Preparation of a nanoscale dihydromyricetin-phospholipid complex to improve the bioavailability: in vitro and in vivo evaluations. Eur J Pharm Sci 138:104994. https://doi.org/10.1016/j.ejps.2019.104994.
- Zhao Z, Yin JQ, Wu MS, et al. (2014). Dihydromyricetin activates AMP-activated protein kinase and P38MAPK exerting antitumor potential in osteosarcoma. Cancer Prev Res (Phila) 7:927–38. https://doi.org/10.1158/1940-6207.CAPR-14-0067.
- Zhou DZ, Sun HY, Yue JQ, et al. (2017). Dihydromyricetin induces apoptosis and cytoprotective autophagy through ROS-NF-kappaB signalling in human melanoma cells. Free Radic Res 51:517–28. https://doi.org/10.1080/10715762.2017.1328552.
- Zhou Y, Liang X, Chang H, et al. (2014). Ampelopsin-induced autophagy protects breast cancer cells from apoptosis through Akt-mTOR pathway via endoplasmic reticulum stress. Cancer Sci 105:1279–87. https://doi.org/10.1111/cas.12494.
- Zhu H, Luo P, Fu Y, et al. (2015). Dihydromyricetin prevents cardiotoxicity and enhances anticancer activity induced by adriamycin. Oncotarget 6:3254–67. https://doi.org/10.18632/oncotarget.2410.
- Zuo AR, Dong HH, Yu YY, et al. (2018). The antityrosinase and antioxidant activities of flavonoids dominated by the number and location of phenolic hydroxyl groups. Chin Med 13:51. https://doi.org/10.1186/s13020-018-0206-9.