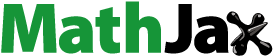
Abstract
Transfersome has been developed to enhance dermal delivery of amniotic mesenchymal stem cell metabolite products (AMSC-MP). AMSC-MP contains many growth factors for managing skin aging, thus improving the quality of an adjusted life year. This study aims to determine the effect of surfactant types acting as the edge activator on transfersome-loading AMSC-MP. Transfersome was prepared by thin-layer hydration method and composed of l-α-phosphatidylcholine as a phospholipid and three types of surfactants, namely; cationic (stearylamine), anionic (sodium cholate), and nonionic surfactant (Tween 80) at a weight ratio of 85:15, respectively. Transfersomes were evaluated for physical characteristics, penetration, effectiveness, and safety. The results showed that sodium cholate, an anionic surfactant, produced the smallest transfersome particle size, i.e., 144.2 ± 3.2 nm, among all formulas. Trans-SA containing stearylamine had a positive charge of 41.53 ± 6.03 mV compared to Trans-SC and Trans-TW, whose respective charges were –56.9 ± 0.55 mV and –41.73 ± 0.86 mV. The small particle size and low negative value of zeta potential enabled high dermal penetration by transfersomes containing AMSC-MP, while the positive charge of stearylamine hindered its penetration of deeper skin layers. Trans-SC and Trans-TW produced higher collagen density values at 77.11 ± of 4.15% and 70.05 ± of 6.95%, than that of Trans-SA. All the AMSC-MP transfersomes were relatively safe with 0.5–1.0 macrophage cell numbers invaded the dermis per field of view. In conclusion, sodium cholate, an anionic surfactant, demonstrated considerable capacity as the edge activator of transfersome-loading AMSC-MP for skin anti-aging therapy.
1. Background
Skin aging, a complex progressive biological process caused by both intrinsic and extrinsic factors, negatively affects its appearance (Sjerobabski-Masnec & Šitum, Citation2010). The extrinsic impact of UV radiation is referred to as photoaging (Ahmad & Damayanti, Citation2018) which produces free radicals potentially detrimental to the structure and lining of the dermis by reducing both the number of fibroblast cells and collagen density. Reduced collagen synthesis results in skin aging, characterized by compromised skin elasticity, as well as the appearance of fine lines and dark blemishes. The use of anti-aging cosmetics seeks to prevent skin damage by increasing collagen density and the number of fibroblast cells (Ganceviciene et al., Citation2012).
Amniotic mesenchymal stem cell metabolite products (AMSC-MP) constitute a conditioned media of mesenchymal stem cell cultures of the amnion membrane. AMSC-MP contain significant amounts of growth hormone that possesses anti-aging properties (Islam et al., Citation2014) including transforming growth factor beta (TGF-β), epidermal growth factor (EGF), basic fibroblast growth factor (bFGF), and keratinocytes growth factor (KGF) (Sari et al., Citation2020). Growth hormones, especially TGF-β, can increase extracellular matrix (ECM) production, including collagen and fibroblasts (Shin et al., Citation2019), and inhibit ECM degradation. TGF-β controls collagen homeostasis by regulating collagen production and degradation through the Smad pathway. On the other hand, the growth hormone in AMSC-MP consists predominantly of hydrophilic macromolecules >25 kDa in size, while hydrophilic molecules measuring >500 Da experience difficulty in penetrating the skin (Pratiwi et al., Citation2018). Consequently, penetrating the dermis to produce effects requires delivery carriers such as transfersomes.
Transfersomes represent an artificial vesicular system possessing ultra-deformable properties and an aqueous core surrounded by a double layer of phospholipids (Cevc Citation2004; Kamran et al. Citation2016). Their ability to deform enables them to pass through narrow skin pores and serve as carriers of drugs either high or low in molecular weight. They penetrate the epidermis by modifying intercellular lamellar lipids present in the stratum corneum (Cevc and Blume Citation1992; Imam et al. Citation2017). A study conducted by Surini et al. (Citation2018) developed a transfersomal anti-aging product containing Centella asiatica extract, a transfersomal gel with twice the penetrative ability than that of the control gel, as the active cosmetic ingredient. In addition, transfersomes have also been employed as a delivery system for proteins and peptides that penetrate the skin with difficulty due to large biogenic molecules and degradation in the gastrointestinal tract when administered orally (Pawar et al., Citation2016)
Transfersomes consist of phospholipids and edge activators, whereas phospholipids are xerophobic and tend to avoid a dry environment. Transfersomes follow the osmotic gradient of the skin, penetrating its deeper layers where the water content is higher than that of the surface, through the intercellular gap in the stratum corneum (Cevc, Citation2003). The addition of edge activators may affect transfersome deformability (Yang et al., Citation2019). Surfactants have been known to act as edge activators that increase the deformability of the double layer of phospholipids by lowering interfacial tension and affecting membrane curvature (Surini et al., Citation2018).
The difference in charge between the functional groups of the surfactant will affect transfersome penetration (Reningtyas & Mahreni, Citation2015). Gupta & Rai (Citation2017) reported that surfactants with nonionic charges penetrate more rapidly than those containing ionic functional groups. They also explained that cationic-charged surfactants will be adsorbed on the surfaces of cell membranes contained in the negatively charged, cutaneous surface whereas anionic-charged surfactants are only adsorbed in a neutral double layer. The difference in charge within the surfactant functional group represents the basis for selecting those surfactants to be studied, namely nonionic, cationic, and anionic.
Lee et al. (Citation2005) reported that transfersomal cream formulations made for DNA delivery are distinguished by the type of surfactant, i.e., anionic (sodium cholate) or nonionic (Tween 80), they contain. The use of sodium cholate and Tween 80 as the edge activators in transfersomes has also been reported (Abdel-Hafez et al., Citation2018). Transfersomes prepared with Tween 80 have a larger particle size and a lower zeta potential value compared to sodium cholate. The higher the potential zeta value, the stronger the repulsive force between particles. Transfersomes prepared with sodium cholate in DNA delivery are more stable than those prepared with Tween 80 (Moghassemi & Hadjizadeh, Citation2014).
In this study, the use of surfactants with different charges was evaluated for its effect on the physical characteristics, in vivo skin penetration, and in vivo anti-aging effectiveness of transfersomes containing AMSC-MP. This was both in terms of collagen density and fibroblast count, as well as safety evaluations in UV aging-induced mice. The types of surfactants comprised Tween 80 as the nonionic surfactant, stearylamine as the cationic surfactant, and sodium cholate as the anionic surfactant.
2. Materials and method
2.1. Materials
AMSC-MP were obtained from the Stem Cell Research and Development Center, Universitas Airlangga, Indonesia. Approval for collecting human placenta tissues was granted by the Ethical Committee of Universitas Airlangga Hospital with certificate number 101/KEH/2019, dated January 10, 2019. l-α-Phosphatidylcholine was a product of Sigma-Aldrich Ltd. (Buchs, Switzerland). Tween 80 stearylamine was acquired from Sigma-Aldrich Ltd (Switzerland), while sodium cholate was purchased from Sigma-Aldrich Ltd. (New Zealand). For the purposes of the penetration study, 1,2-dipalmitoyl-sn-glycero-3-phosphoethanolamine-N-(lissamine rhodamine B sulfonyl) (ammonium salt) (16: 0 Liss-Rhod PE) was obtained from Avanti Polar Lipids Inc. (USA). All other reagents used were of the non-technical grade available.
2.2. Preparation of transfersomes
Transfersomes were prepared by dissolving l-α-phospatidylcholine and the surfactants i.e., Tween 80, sodium cholate, and stearylamine in chloroform before mixing them homogeneously in a 50-mL round-base flask at the appropriate amounts shown in . The chloroform was subsequently evaporated using a rotary vacuum evaporator at a temperature of 55 °C and a velocity of 150 rpm. After the solvent had been completely evaporated, a thin lipid film that formed at the bottom of the flask was hydrated using AMSC-MP. The mixtures were vortexed and sonicated at room temperature for 30 min until a suspension was formed which was then extruded through 400 nm and 200 nm polycarbonate membranes (Avanti Mini Extruder®, Avanti Lipids Inc., USA), and AMSC-MP. The loaded transfersomes obtained were characterized by the formation of a transparent emulsion-like liquid. For the in vivo skin penetration study, transfersomes were added to Liss Rhod PE at a concentration of 0.1% of the total lipid moles and prepared using the same method.
Table 1. Formulation of transfersome-loading amniotic mesenchymal stem cell metabolite products prepared with different types of surfactant.
2.3. Physical characteristics and morphology of transfersome
Approximately100 μL of the transfersome was diluted with 2 mL of demineralized water. Tests relating to particle size, polydispersity index, and zeta potential were conducted through dynamic light scattering and electrophoresis light scattering methods involving the use of Malvern Zetasizer Instruments (Malvern Panalytical Ltd., UK) at a temperature of 25 °C. Evaluation of transfersome morphology was undertaken using a scanning electron microscope (SEM) at the Division of Materials Characterization, Faculty of Industrial Engineering, Tenth of November Institute of Technology, Surabaya. The samples were air-dried onto SEM stubs using carbon tape before being sputter-coated with iridium to a thickness of 20 nm.
2.4. Fourier-transform infrared spectroscopy analysis
The Fourier-transform infrared (FTIR) profiles of transfersome-loaded AMSC-MP were analyzed using an FTIR spectrophotometer (Shimadzu, Kyoto, Japan). The freeze-dried transfersomes were prepared with potassium bromide at a weight ratio of 1:100, before being pressed to form thin, translucent pellets that were subsequently examined at wavenumbers of 4000–400 cm−1.
2.5. Differential thermal analysis of transfersomes
The freeze-dried transfersomes were placed in aluminum crucibles and heated from 30 °C to 300 °C at a rate of 10 °C/min using a differential thermal analysis (DTA) instrument (Mettler Toledo FP 85, Switzerland).
2.6. SDS-PAGE analysis
Qualitative analysis of the AMSC-MP-loaded transfersomes was conducted by means of sodium dodecyl sulfate polyacrylamide gel electrophoresis (SDS-PAGE) that is used to detect and separate proteins according to their molecular weight. The preparation was analyzed using Mini-Protean Tetra Cell® (Bio-Rad Laboratories Ltd.) at the Institute of Tropical Disease, Campus C, Universitas Airlangga. The samples were diluted with phosphate buffered saline pH 7.4 (1:1 vol/vol) and inserted into 30 μL of gel which was then incubated in a Fixer solution containing 40% ethanol, 10% acetic acid, and 50% demineralized water for 1 h, before being washed with demineralized water for 30 min. The gel was incubated in 0.02% sodium thiosulfate solution for 1 min, washed with demineralized water for 3 × 20 s, incubated in a 0.1% silver nitrate solution for 200 min at a temperature of 4 °C and, finally, washed again with demineralized water for 3 × 20 s. The gel was then placed on a coloring tray, washed with demineralized water for 1 min, developed with 3% sodium carbonate solution and re-washed with demineralized water for 20 s. At this point, the staining process was stopped by adding 5% acetic acid solution prior to incubation of 5 min duration. Finally, the gel was deposited in a 1% acetic acid solution at a temperature of 4 °C.
2.7. In vivo skin penetration study
For the in vivo studies, 6- to 8-week-old mice (Mus musculus) weighing 20–25 g which had been acquired from the Faculty of Veterinary, Universitas Airlangga served as the experimental subjects with a study protocol approved by its Ethics Commission (Certificate number 2.KE.057.05.2021, dated May 25, 2021).
The in vivo skin penetration study focused on four treatment groups, each containing four subjects, i.e., control (liposome), AMSC-MP-loaded transfersome prepared with stearylamine (Trans-SA), Tween 80 (Trans-TW), and sodium cholate (Trans-SC). In this study, Lis RHOD PE was added to the liposomes or transfersomes. First, the hair on the subjects’ backs was shaved before skin aging was induced through daily exposure to UV rays at a dose of 80 mJ/cm2 for a period of 1 week. The subjects were anesthetized with ketamine through an intraperitoneal dose of 20 mg/kg body weight. A glass ring with a diffusion area of 2.54 cm2 was adhered to the skin of the subjects’ backs to which the samples were subsequently applied non-occlusively. The subjects were sacrificed by means of cervical dislocation either 1 or 2 h after administration of the samples. An area of skin was gently cleaned with saline applied by means of cotton swabs before being excised for further analysis. The skin tissue was wrapped in aluminum foil and placed in an ultradeep freezer (–80 °C) in preparation for cryosection to be performed with a 1959 UV Cryostat Leica CM to a thickness of 16 μm. The tissue slides were later observed using a fluorescence microscope.
2.8. In vivo skin anti-aging efficacy study: evaluation of collagen density and number of fibroblasts
Skin aging in the subjects whose back fur had been removed was induced by UV-B light exposure at an intensity of 80 mJ/cm2. Daily irradiation lasting 34 min was carried out for seven days. The sample was subsequently applied to a 2.54 cm2 area of skin on each subject’s back at two-day intervals for a period of 2 weeks. On day 15, the subjects were sacrificed with their skin being excised and soaked in Neutral Buffered Formalin (NBF) solution in order to make histopathological tissue preparations. The skin tissue was then cut using a microtome and stained with Mallory acid for collagen fibril evaluation and hematoxyline–eosine staining to enable calculation of the number of fibroblasts. The tissue slides prepared were observed under a light microscope. Analysis of collagen fibrils was carried out using J-Images Software, while the fibroblasts were counted manually.
2.9. Skin irritation evaluation: number of macrophages
For the purposes of this study, the subjects’ shaved back skin was applied to the samples within an area measuring 2.54 cm2. Twenty-four hours after application, the subjects were sacrificed and a skin sample excised, prepared for tissue slides by paraffin block method, and stained with hematoxyline-eosine. The skin tissue was then subjected to quantitative analysis for evidence of irritation by calculating the number of macrophages under a light microscope.
2.10. Statistical analysis
The numerical data that was analyzed for normal distribution by means of a Kolmogorov–Smirnov test indicated the average ± standard deviation. If the data was normal (p value ≥.05), it was subjected to a quantitative one-way analysis of variance. If the p value <.05, the data analysis was followed by a post hoc Tukey HSD test to evaluate the significant differences between the groups. In cases of data that was not distributed normally, a non-parametric analysis was performed using Kruskal–Wallis and pairwise comparison tests.
3. Results
3.1. Physical characteristics of transfersome-loading AMSC-MP
The results in indicate that the particle sizes of all transfersomes were below 500 nm. The highest particle size of 472.2 ± 11.7 nm was observed in the case of Trans-SA formula, followed by 179.6 ± 1.1 nm for Trans-TW, and 144.2 ± of 3.2 nm for Trans-SC. A polydispersity index (PDI) analysis showed that Trans-SA, Transf-TW, and Trans-SC had PDI values of 0.251 ± 0.031, 0.198 ± 0.006, and 0.168 ± 0.099, respectively, indicating homogeneous particle size distribution (Miatmoko et al., Citation2021). Measurement of the zeta potential was undertaken to quantify the stability of the nanoparticles during storage (Sadeghi et al., Citation2015). The stability of the system increased if the value of the potential zeta ≥ │±30│mV. From the data aforementioned, the highest successive zeta potential values were confirmed as follows: Trans-SC –56.9 ± 0.55 mV, Trans-TW –41.73 ± 0.86 mV, and Trans-SA 41.53 ± 6.03 mV. All formulas had a potential zeta value of ≥ │±30│mV meaning that the entire formula demonstrated high levels of system stability due to the repulsive force between particles that prevented aggregation (Pertiwi et al., Citation2018).
Figure 1. Physical characteristics of transfersome-loading AMSC-MP prepared with different types of surfactants, i.e., Tween 80 (Trans-TW), stearylamine (Trans-SA), and sodium cholate (Trans-SC) measured for (A) particle size, (B) polydispersity index, and (C) zeta potential. The results were measured in three replications. *p < .05 compared to Trans-SA. #p < .05 compared to Trans-TW.
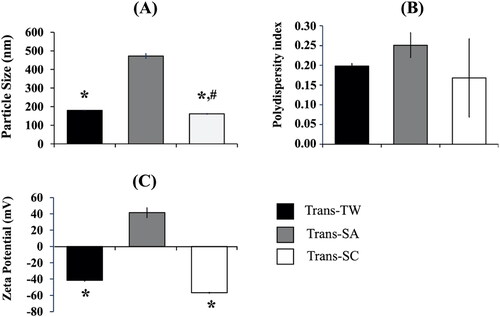
3.2. Morphology of transfersome-loading AMSC-MP by scanning electron microscopy
On morphological evaluation, the results showed that Trans-SA and Trans-TW had a single, spherical vesicular form, whereas, as shown in , the Trans-SC contained clustered spherical vesicles.
3.3. FTIR analysis of transfersome-loading AMSC-MP
The FTIR analysis results were further evaluated to establish the nature of the interaction between AMSC-MP and transfersome components. This involved determining changes to the absorption bands of particular functional groups within specific wavenumbers, as shown in .
Figure 3. Fourier transform infrared spectra of (A) components of transfersomes, i.e., AMSC-MP, l-α phosphatidylcholine, stearylamine, Tween 80, sodium cholate; and (B) liposome and transfersome-loading AMSC-MP prepared with different surfactants as the edge activators, i.e., Trans-SA, Trans-TW, and Trans-SC.
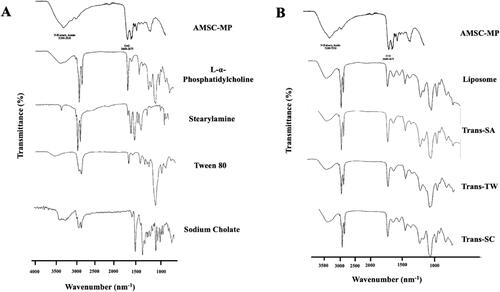
The results show that formulating AMSC-MP into transfersomes changed the infrared spectra profile of AMSC-MP. In contrast, adding surfactants, i.e., SA, SC, TW, to the Trans-SA, Trans-TW, Trans-SC, and liposomes, resulted in identical IR spectra profiles, as shown in . The AMSC-MP transfersomes and liposomes had an absorption band of the N–H group at a wavenumber of 3200–3350 cm−1 identical to the absorption band that appeared in AMSC-MP. Moreover, the presence of an absorption band for the C = O group at a wavenumber of 1082 cm–1 indicated that the observed spectra are identical in terms of liposomes and the three transfersome formulas. The N–H and C = O groups are characteristic of protein functional groups specific to AMSC-MP. In addition, specific absorption bands of the N–H group within the wavenumber range of 2854–3000 cm−1 appeared in both the spectra of Trans-SA, Trans-SC, and Trans-TW, as well as the liposome representing the acyl chain of l-α phosphatidylcholine. No specific absorption bands were observed for surfactants, i.e., SA, SC, and TW of the transfersomes. In addition, no new peaks indicating that physical interaction had occurred were evident, and no chemical interaction was detected.
3.4. DTA analysis of transfersome-loading AMSC-MP
The effects of surfactant use in transfersome-loading AMSC-MP were further evaluated for changes in the physical characteristics of the transfersomes due to DTA. As shown in , the l-α-phosphatidylcholine experienced an endothermic peak at 127.7 °C, whereas the stearylamine thermogram reached three endothermic peaks at temperatures of 66.9 °C, 108.9 °C, and 150.8 °C. The sodium cholate thermogram indicates two endothermic peaks at 221.4 °C and 262.0 °C.
Figure 4. Thermogram profiles (A) l-α-phosphatidylcholine, stearylamine, and sodium cholate constituting the transfersome components, and (B) liposome and transfersome-loading AMSC-MP prepared with different types of surfactants i.e., stearylamine (Trans-SA), Tween 80 (Trans-TW), and sodium cholate (Trans-SC).
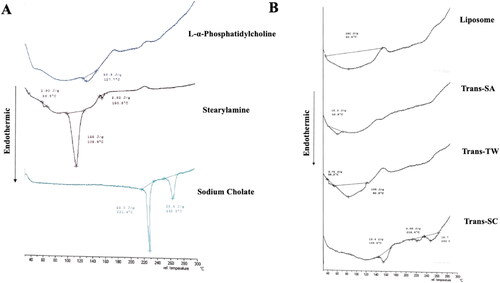
For the DTA evaluation, the three transfersome formulae, namely: Trans-SA, Trans-SC, and Trans-TW, had different thermogram profiles. An endothermic peak occurred in the thermogram of AMSC-MP liposome at 82.5 °C, indicating multiple peak shifts when compared to its component l-α phosphatidylcholine which was at 127.7 °C. The Trans-SA thermogram experienced an endothermic peak at 68.8 °C which was identical to that of stearylamine occurring at 66.9 °C. On the other hand, Trans-SC experienced endothermic peaks at 148.6 °C, 216.4 °C, and 249.5 °C with peak broadening appearing compared with sodium cholate. Meanwhile, Trans-TW experienced two endothermic peaks at 38.2 °C and 82.9 °C which were identical to those occurring in liposome and l-α-phosphatidylcholine.
3.5. SDS-PAGE analysis of transfersome-loading AMSC-MP
To measure the ability of transfersomes to load active substances of AMSC-MP, an SDS PAGE analysis was performed. The results confirmed the presence of an AMSC-MP-free major band in the same elution area as the protein ladder band with a molecular weight of 55–70 kDa. The use of Trans-TW confirmed the presence of marker proteins of major AMSC-MP components at molecular weights similar to 55–70 kDa, but fewer free proteins characterized by thin bands than liposomes (the control group) as presented in . Trans-SC was shown to contain fewer free proteins compared to Trans-TW, while Trans-SA contained the lowest level of such proteins compared to the others as confirmed by observation of the thinnest band. This indicated the highest capacity to entrap the active substances of AMSC-MP as shown by the contents of .
3.6. In vivo skin penetration study results
From the skin tissue preparations, the depth to which the vesicles of liposomes, Trans-SA, Trans-TW, and Trans-SC penetrate the skin layer is evident from the red-fluorescence intensity of Liss Rhod PE contained in the vesicles. The results shown in indicate that liposomes caused minimal dermis penetration, while fluorescence intensity was concentrated on the skin’s surface (stratum corneum). Trans-SA demonstrated the highest red fluorescence intensity in the area of the stratum corneum indicating that the vesicles had been retained in the upper skin layer. On the other hand, Trans-TW was observed to penetrate as far as the deeper skin layer which was not the case with Trans-SA. However, its intensity remained lower than that of Trans-SC which produced the highest vesicle penetration of the dermis. The surfactant charge significantly affected the skin penetration of transfersome-loading AMSC-MP.
Figure 6. In vivo skin penetration of liposome, transfersome containing stearylamine (Trans-SA), transfersome containing sodium cholate (Trans-SC), and transfersome containing Tween 80 (Trans-TW)-loading AMSC-MP with the addition of Lis Rhod PE at a concentration of 0.1% mole of total lipid at 1 and 2 hours after topical application to a 2.54 cm2 area of skin on the subjects’ backs.
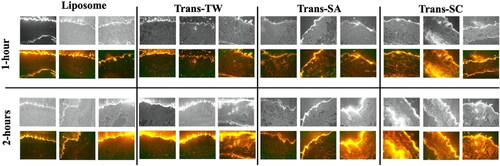
3.7. In vivo antiaging effectivity study: collagen density
The data shows that skin aging due to UV exposure that can reduce and, in more extreme cases, damage skin collagen through the production of reactive oxygen species (ROS) in the dermis resulted in decreased collagen density. The negative control group experienced the lowest level of collagen, as shown in . The collagen density values ranging from highest to lowest were as follows: Trans-SC of 77.11 ± 4.15%, Trans-SA of 71.81 ± 5.93%, and Trans-TW of 70.05 ± 6.95%. Trans-SC and Trans-TW had higher collagen density values than that of the normal skin group of 67.69 ± 2.87%, as presented in .
Figure 7. Photomicroscopy of collagen density in the dermis layer of the dorsal skin of subjects without UV light induction (normal skin group) and those in the UV light induction group (UV-aging skin negative control) with administration of free AMSC-MP, transfersome containing stearylamine (Trans-SA), transfersome containing sodium cholate (Trans-SC), and transfersome containing Tween 80 (Trans-TW)-loading AMSC-MP. The samples were subsequently applied to a 2.54 cm2 area of skin on the back of each subject once every two days for a period of two weeks.
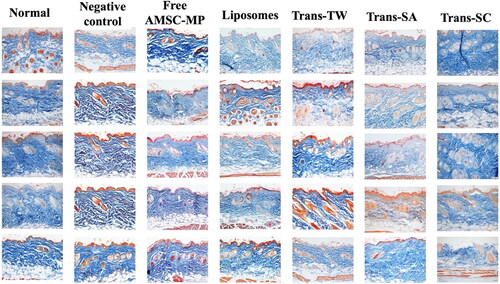
Figure 8. Quantitative histogram analysis of collagen density of the dermis layer of the dorsal skin of subjects without UV light induction (normal skin group) and the UV light induction group (UV-aging skin negative control) with administration of free AMSC-MP, transfersome containing stearylamine (Trans-SA), transfersome containing sodium cholate (Trans-SC), and transfersome containing Tween 80 (Trans-TW)-loading AMSC-MP. The samples were subsequently applied to a 2.54 cm2 area of skin on the back of each subject once every two days for a period of two weeks. *p < .05.
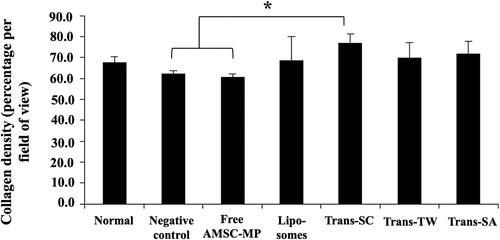
3.8. In vivo antiaging effectivity study: fibroblast number
The evaluation results relating to anti-aging activity were analyzed by evaluating the number of fibroblast cells capable of producing collagen. The more numerous the fibroblasts, the greater the quantity of collagen formed. Compared to the normal skin group, AMSC-MP administration increased the number of fibroblast cells to 30 ± 6.79 cells per field of view, a level relatively similar to Trans-SA or Trans-SC, as shown in .
Figure 9. The number of fibroblasts contained in the dorsal skin tissue slides of subjects not subjected to UV light induction (normal skin group) and the UV light induction group (UV-aging skin negative control) following administration of free AMSC-MP, transfersome containing stearylamine (Trans-SA), transfersome containing sodium cholate (Trans-SC), and transfersome containing Tween 80 (Trans-TW)-loading AMSC-MP. The samples were subsequently applied to a 2.54 cm2 area of the skin on the back of each subject once every two days for a period of two weeks.
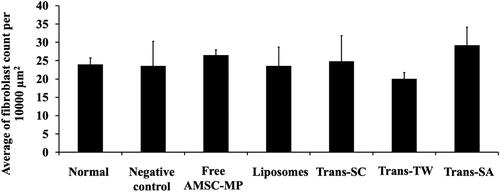
3.9. In vivo skin irritation study: macrophage cell number
As presented in , the minimum number of macrophage cells, 0.3 cells per field of view, was recorded in the AMSC-MP-treated group, while the use of liposomes and transfersomes relatively increased the number of macrophages to as many as 0.8–1 cell per field of view. In contrast, Trans-TW registered the lowest number of cells at 0.5 per field of view. Although formulating AMSC-MP into transfersomes resulted in more numerous macrophage cells, its effect remained minimal. Nevertheless, the results seems to be considerable taking into account the deviation of macrophage numbers observed in negative control groups which could be due to the natural variation of the immune response to foreign matter.
Figure 10. The histopathological evaluation of macrophage cell numbers on the dorsal skin of subjects’ tissue slides without UV light induction (normal skin group) and the UV light induction group (UV-aging skin negative control) following administration of free AMSC-MP, transfersome containing stearylamine (Trans-SA), transfersome containing sodium cholate (Trans-SC), and transfersome containing Tween 80 (Trans-TW)-loading AMSC-MP to a 2.54 cm2 area of skin on the back of each subject for twenty-four hours. *p < .05; ***p < .005.
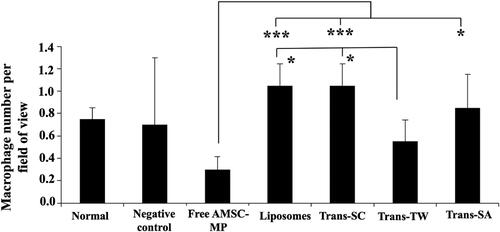
4. Discussion
This study evaluates the effect of surfactants with various charges on the physical characteristics of transfersomes. This significantly influences the in vivo skin penetration and anti-aging effectiveness of AMSC-MP in terms of collagen density and the number of fibroblasts. It also affects the safety evaluations reviewed according to the number of macrophages present in dermal tissue. The types of surfactants used included Tween 80 as a nonionic surfactant, stearylamine as a cationic surfactant, and sodium cholate as an anionic surfactant.
The particle size data provides an overview of the penetration of the skin by active cosmetic ingredients. The smaller the particle size, the greater the contact between the active ingredient and the stratum corneum and the larger the skin pores, both factors that facilitate penetration of the dermis by active substances (Pardeike et al., Citation2010). AMSC-MP transfersomes prepared with the anionic surfactant (sodium cholate) tend to have a smaller particle size than the uncharged surfactant (Tween 80). This finding is in accordance with that of the research conducted by Namdeo & Jain (Citation1999). The use of a negatively charged surfactant has been reported to reduce the average size of the particles because the negative charge renders the complex lipid bilayer of the transfersome liable to curvature due to the attractive force between the positively charged choline group on phospholipids and the negative charge on the surfactant sodium cholate (Gillet et al., Citation2011). The cationic surfactant stearylamine has a larger particle size than the nonionic surfactant, Tween 80, probably because the positively charged amine group in the stearylamine will be repulsed by the positively charged choline group of l-α-phosphatdiylcholine.
The zeta potential value is highly influenced by the ion charge of the surfactant which will also cause the transfersome to become charged. This, in turn, will affect the penetration and, consequently, effectiveness of the preparation in bringing the active ingredient into the therapeutic target in relation to the negative charge of the dermal cells. Transfersomes with nonionic surfactant, Tween 80, have a slight negative charge and are, therefore, considered neutral (Dragicevic-Curic et al., Citation2010) due to the adsorption of hydroxyl ions (OH–) from water to the particle surface (Tian et al., Citation2016). Transfersomes with cationic surfactant, i.e., stearylamine, have a positive zeta potential value due to the positively charged amine groups making up the zeta potential of the bilayer membrane on liposomes (Tian et al., Citation2016). Transfersomes with the anionic surfactant, sodium cholate, are negatively charged because this surfactant adsorbs hydroxyl ions (OH–) from water to the particle surface (Tian et al., Citation2016).
A morphological evaluation using SEM obtained from Trans-SC indicates an apparent clustering of particles, probably due to the presence of attractive forces between the protein molecules in AMSC-MP. Positively charged growth hormones and negatively charged sodium cholate cause these clustered vesicles that may indicate unstable particle dispersion. In response, charge stabilizing agents can be added to stabilize these vesicles in order to prevent their aggregation.
The use of different surfactants in transfersome-loading AMSC-MP produces identical FTIR profiles with no new absorption band. However, the major bands observed differed from their constituents, an indication that the physical interaction occurred between the transfersome components and protein contained in the AMSC-MP. This line of argument is supported by the DTA thermograms that contain the endothermic peaks in Trans-TW that are identical to those evident in l-α-phosphatidylcholine and AMSC-MP liposomes. The absence of endothermic peaks and peak shifts in Trans-SA, Trans-TW, and Trans-SC, when compared to liposomes, confirms the weak energy present in the transition phase which is possibly due to the decrease in van der Waals interactions within the phospholipid bilayer membrane. These results indicate reduced regularity of the phospholipid structure in the vesicles due to surfactant insertion (Miatmoko et al., Citation2021).
Based on the evaluation of SDS-PAGE, transfersomes prepared with stearylamine demonstrated the optimum trapping efficiency of the thinnest free protein band present in the gel compared to other groups. The trapping efficiency of cationic surfactants is superior to that of anionic surfactants as demonstrated by the research conducted by Chang & Flanagan (Citation1994). Cationic surfactants in transfersomes will experience spontaneous electrostatic forces of AMSC-MP proteins. From the results, it appears that the transfersomes had a qualitatively reduced amount of free protein in comparison to the liposome which indicates that transfersomes can trap more protein as the active ingredients in vesicles than can conventional liposomes. The authors strongly recommend that future studies should evaluate whether the interaction involves membrane–protein interplay inside the aqueous core of transfersomes or the outer surface of the vesicle, or whether it also affects the lipid bilayer membrane, thereby promoting potential formulations for protein-related substance delivery.
In the case of transfersomes, potentially high levels of skin penetration are mainly influenced by the transcellular penetration mechanism. The evaluation of Trans-SA showed that particles can penetrate the dermis and that they tend to interact with and, therefore, penetrate the uppermost layer of skin. This could be due to the positive charge of the cationic stearylamine molecules reacting to the negatively charged skin layer (Gillet et al., Citation2011) resulting in a preferential accumulation in the stratum corneum. In contrast, the Trans-TW results showed that transfersome vesicles can penetrate the skin more effectively than those of Trans-SA. Transfersomes have been reported as improving the skin permeability of drugs by carrying intact the encapsulated drug penetrating the stratum corneum across a transepidermal osmotic gradient (El Zaafarany et al., Citation2010). On the other hand, transfersomes modify the intercellular lipids of the stratum corneum, thus increasing its fluidity, before the drug can penetrate (El Maghraby et al., Citation2001). Tween itself has the ability to compromise the stratum corneum structure by extracting some of the intercellular lipids present (Hathout et al., Citation2010). In addition, as previously reported (El Zaafarany et al., Citation2010), the presence of Tween 80 results in the high deformability of transfersome vesicles due to its non-bulky structure and flexible hydrocarbon chain. The hydrophilic properties of Tween 80 results in an extensive covered area on the surface moiety of the vesicles, thus reducing interfacial tension (Khan et al., Citation2021).
The results for Trans-SC indicated that transfersome vesicles can penetrate more effectively than any other type of surfactant. Uncharged or nonionic group molecules tend not to be retained in the upper skin layer and can, therefore, penetrate the deeper ones. Sodium cholate, an anionic surfactant, will cause transfersome vesicles to become negatively charged, thereby increasing transfersome penetration of the skin because the stratum corneum layer is negatively charged (Sinico et al., Citation2005; Yoo et al., Citation2008). The transfersome vesicles do not interact markedly with the skin layer with the result that diffusion into the deeper layers by carrying the AMSC-MP active ingredient encapsulated in the vesicle becomes easier. This finding matches that relating to the use of anionic surfactant (sodium cholate) which indicates that a more negative zeta potential value renders the resulting formula more stable and with an enhanced penetrative ability (Al Shuwaili et al., Citation2016). In a study conducted by Shaji & Lal, (Citation2014), the presence of an anionic surfactant, sodium deoxycholate, in the transfersomal system of transdermal delivery of COX-2 inhibitors was shown to achieve highly effective penetration (Shaji & Lal, Citation2014). Moreover, nonionic surfactants contain highly flexible hydrocarbon chains that facilitate their penetration of the skin (Gupta & Rai, Citation2017).
It was also reported that the presence of charge on the surface of the vesicles will affect drug diffusion. The negative charge on the vesicles has a greater flux than the positive one which will increase accumulation in superficial skin (Gillet et al., Citation2011). Therefore, transfersome containing anionic surfactant of sodium cholate has been shown to increase the collagen density of the skin. AMSC-MP that contain growth hormone and cytokines when they reach the therapeutic target, the viable dermis layer, will then increase collagen formation (Lee et al., Citation2014). The free AMSC-MP treatment produced a collagen density value of 60.53 ± 1.47% which was similar to that of the UV negative control of 62.16 ± 1.47%. This is because the growth hormone present in AMSC-MP consists largely of hydrophilic macromolecules >25 kDa in size, while hydrophilic molecules measuring >500 Da have difficulty penetrating the skin (Pratiwi et al., Citation2018). This renders it more challenging for AMSC-MP to reach the target of anti-aging therapy which is located in the viable dermis layer of the skin with the result that it cannot repair UV exposure-induced collagen damage.
The application of anti-aging AMSC-MP is highly effective in preventing cell damage and is regarded as capable of inhibiting the aging process. AMSC-MP contain many growth hormones that function as anti-aging agents in the same way as TGF-β, EGF, bFGF, and KGF) (Islam et al., Citation2014). This study highlighted an increase in the number of fibroblast cells in the AMSC-MP treatment group which was in line with the anti-aging effects of AMSC-MP whose use promotes the proliferation and migration of dermal fibroblasts and increases collagen synthesis of fibroblasts (Ardhaninggar et al., Citation2020).
These results indicate that AMSC-MP did not cause skin irritation, although the AMSC-MP formulation in nanocarriers produced an increase, albeit relatively limited, in the number of inflammatory cells per field of view. This is possibly due to the nature of the constituent materials and the use of surfactants, such as sodium cholate. However, it is tolerable.
5. Conclusions
The anionic surfactant, sodium cholate, induced changes in physical characteristics such as small particle size, more uniform polydispersity index, and negative zeta potential compared to transfersomes using cationic (stearylamine) and nonionic surfactants (Tween 80). The application of sodium cholate successfully improved skin penetration by transfersome-loading AMSC-MP, thereby enhancing their anti-aging effectiveness, in terms of collagen density and the number of fibroblasts, in UV aging-induced mice models. Although the nature of transfersome constituents may cause skin irritation, as evidenced by the increased number of macrophages, the AMSC-MP loaded-transfersomes formulation was relatively safe and the effect remained tolerable. The preferential use of surfactant as the edge activator of transfersome determines, to a significant degree, the characteristics as well as the efficacy of AMSC-MP as a form of anti-aging skin therapy.
Acknowledgment
The authors express their gratitude to Devy Maulidya Cahyani and Berlian Sarasitha Hariawan for their kind support during the animal experiments and some of literature review which constituted parts of this research.
Data availability statement
The data that support the findings of this study are available from the corresponding author [AM] upon reasonable request.
Disclosure statement
No potential conflict of interest was reported by the authors.
Additional information
Funding
References
- Abdel-Hafez SM, Hathout RM, Sammour OA. (2018). Curcumin-loaded ultradeformable nanovesicles as a potential delivery system for breast cancer therapy. Colloids Surf B Biointerfaces 167:63–72.
- Ahmad Z, Damayanti D. (2018). Penuaan kulit: patofisiologi dan manifestasi klinis. Berkala Ilmu Kesehatan Kulit Dan Kelamin – Period Dermatol Venereol 30:208–15.
- Al Shuwaili AH, Abdul Rasool BK, Abdulrasool AA. (2016). Optimization of elastic transfersomes formulations for transdermal delivery of pentoxifylline. Eur J Pharm Biopharm 102:101–14.
- Ardhaninggar AA, Murtiastutik D, Sawitri S, Prakoeswa CRS. (2020). The efficacy of topical combination amniotic membrane stem cell metabolite product (AMSC-MP) and vitamin E after microneedling in photoaging. BIKK 32:126.
- Cevc G, Blume G. (1992). Lipid vesicles penetrate into intact skin owing to the transdermal osmotic gradients and hydration force. Biochim Biophys Acta 1104:226–32.
- Cevc G. (2003). Transdermal drug delivery of insulin with ultradeformable carriers. Clin Pharmacokinet 42:461–74.
- Cevc G. (2004). Lipid vesicles and other colloids as drug carriers on the skin. Adv Drug Deliv Rev 56:675–711.
- Chang H-C-C, Flanagan DR. (1994). Liposomal entrapment of suramin. J Pharm Sci 83:1043–6.
- Dragicevic-Curic N, Gräfe S, Gitter B, et al. (2010). Surface charged temoporfin-loaded flexible vesicles: in vitro skin penetration studies and stability. Int J Pharm 384:100–8.
- El Maghraby GM, Williams AC, Barry BW. (2001). Skin delivery of 5-fluorouracil from ultradeformable and standard liposomes in-vitro. J Pharm Pharmacol 53:1069–77.
- El Zaafarany GM, Awad G. a S, Holayel SM, Mortada ND. (2010). Role of edge activators and surface charge in developing ultradeformable vesicles with enhanced skin delivery. Int J Pharm 397:164–72.
- Ganceviciene R, Liakou AI, Theodoridis A, et al. (2012). Skin anti-aging strategies. Dermatoendocrinol 4:308–19.
- Gillet A, Compère P, Lecomte F, et al. (2011). Liposome surface charge influence on skin penetration behaviour. Int J Pharm 411:223–31.
- Gupta R, Rai B. (2017). Effect of size and surface charge of gold nanoparticles on their skin permeability: a molecular dynamics study. Sci Rep 7:45292.
- Hathout RM, Mansour S, Mortada ND, et al. (2010). Uptake of microemulsion components into the stratum corneum and their molecular effects on skin barrier function. Mol Pharm 7:1266–73.
- Imam SS, Ahad A, Aqil M, et al. (2017). Formulation by design based risperidone nano soft lipid vesicle as a new strategy for enhanced transdermal drug delivery: in-vitro characterization, and in-vivo appraisal. Mater Sci Eng C Mater Biol Appl 75:1198–205.
- Islam R, Shaifur Ra M, Asaduzzama SM, Shahedur R M. (2014). Properties and therapeutic potential of human amniotic membrane. Asian J Dermatol 7:1–12.
- Kamran M, Ahad A, Aqil M, et al. (2016). Design, formulation and optimization of novel soft nano-carriers for transdermal olmesartan medoxomil delivery: in vitro characterization and in vivo pharmacokinetic assessment. Int J Pharm 505:147–58.
- Khan I, Needham R, Yousaf S, et al. (2021). Impact of phospholipids, surfactants and cholesterol selection on the performance of transfersomes vesicles using medical nebulizers for pulmonary drug delivery. J Drug Deliv Sci Technol 66:102822.
- Lee EH, Kim A, Oh Y-K, Kim C-K. (2005). Effect of edge activators on the formation and transfection efficiency of ultradeformable liposomes. Biomaterials 26:205–10.
- Lee HJ, Lee EG, Kang S, et al. (2014). Efficacy of microneedling plus human stem cell conditioned medium for skin rejuvenation: a randomized, controlled, blinded split-face study. Ann Dermatol 26:584–91.
- Miatmoko A, Nurjannah I, Nehru NF, et al. (2021). Interactions of primaquine and chloroquine with PEGylated phosphatidylcholine liposomes. Sci Rep 11:12420.
- Miatmoko A, Safitri SA, Aquila F, Cahyani DM, Hariawan BS, Hendrianto E, Hendradi E, Sari R. (2021). Characterization and distribution of niosomes containing ursolic acid coated with chitosan layer. Res Pharm Sci 16:660–73.
- Moghassemi S, Hadjizadeh A. (2014). Nano-niosomes as nanoscale drug delivery systems: an illustrated review. J Control Release 185:22–36.
- Namdeo A, Jain NK (1999). Niosomal delivery of 5-fluorouracil. J Microencapsul 16:731–40.
- Pardeike J, Schwabe K, Müller RH. (2010). Influence of nanostructured lipid carriers (nlc) on the physical properties of the cutanova nanorepair Q10 cream and the in vivo skin hydration effect. Int J Pharm 396:166–73.
- Pawar A, Jadhav KR, Chaudhari LH. (2016). Transfersome: a novel technique which improves transdermal permeability. Asian J Pharm 10:425–36.
- Pertiwi RD, Djajadisastra J, MUTALIB A, Pujiyanto A. (2018). Pembuatan, Karakterisasi Dan Uji In Vitro Nanopartikel Emas Berbasis Konjugat Gom Arab-Vinkristin. jifi 16:6.
- Pratiwi FD, Murtiastutik D, Rosita C, et al. (2018). Efek Pemberian Topikal Produk Metabolit Cell (PM-AMSC) Pada Penuaan Kulit (Effect of metabolite product amniotic Amniotic Membrane Stem membrane stem cell (MP-AMSC) on skin photoaging). Berkala Ilmu Kesehatan Kulit Dan Kelamin – Period Dermatol Venereol 30:95–101.
- Reningtyas R, Mahreni M. (2015). Biosurfactant. Eksergi 12:12–22.
- Sadeghi R, Gh Etemad S, Keshavarzi E, Haghshenasfard M. (2015). Investigation of alumina nanofluid stability by UV–vis spectrum. Microfluid Nanofluid 18:1023–30.
- Sari DIK, Erawati T, Miatmoko A, et al. (2020). Characterization and stability study of amniotic membrane stem cell metabolite product (AMSC-MP). Int J Pharma Res Health Sci 8:3126–30.
- Shaji J, Lal M. (2014). For enhanced transdermal delivery of COX-2 inhibitors. Int J Pharm Pharm Sci 6:464–77.
- Shin J-W, Kwon S-H, Choi J-Y, et al. (2019). Molecular mechanisms of dermal aging and antiaging approaches. Int J Mol Sci 20:2126.
- Sinico C, Manconi M, Peppi M, et al. (2005). Liposomes as carriers for dermal delivery of tretinoin: in vitro evaluation of drug permeation and vesicle-skin interaction. J Control Release 103:123–36.
- Sjerobabski-masnec I, Šitum M. (2010). Skin aging. Acta Clinica Croatia 48:515–518.
- Surini S, Sarah, Djajadisastra J. (2018). Formulation and in vitro penetration study of transfersomes gel containing gotu kola leaves extract (Centella asiatica L. Urban). JYP 10:27–31.
- Tian Y, Chen L, Zhang W. (2016). Influence of ionic surfactants on the properties of nanoemulsions emulsified by nonionic surfactants span 80/Tween 80. J Dispers Sci Technol 37:1511–17.
- Yang C, Dai X, Yang S, et al. (2019). Coarse-grained molecular dynamics simulations of the effect of edge activators on the skin permeation behavior of transfersomes. Colloids Surf B Biointerfaces 183:110462.
- Yoo J, Shanmugam S, Song CK, et al. (2008). Skin penetration and retention of l-ascorbic acid 2-phosphate using multilamellar vesicles. Arch Pharm Res 31:1652–8.