ABSTRACT
Oratosquilla oratoria rich in myofibrillar protein is often shelled, but traditional methods of shelling have certain limitations. In order to better apply the ultra-high pressure (UHP) technology for shelling, the effects of UHP on rheological properties and structure of myofibrillar protein (MP) from O. oratoria treated with a pressure of 300–400 MPa were determined by rheometer and circular dichroism spectrometer. The results showed that in the frequency ramp tests using rheometer, the complex viscosity and G’ of MP decreased by 59.08% and 73.70%, respectively, when the pressure reached 400 MPa with holding time of 8 min. As frequency increased, the MP exhibited shear thinning; meanwhile, its storage modulus (G’) increased, and solid characteristics became more prominent. In the temperature ramp tests, the MP denatured and its fluidity increased with the increase of temperature. Moreover, the MP from O. oratoria treated with UHP was degraded, and the ionic bond and hydrogen bond were destroyed, while the hydrophobic interaction and disulfide bond increased; the structure of α-helix and β-sheet in the MP converted to β-turn with the increase of pressure and holding time. These findings can provide references for the application of UHP in food processing.
Introduction
Oratosquilla oratoria (O. oratoria), a species of mantis shrimp (also known as climbing shrimp), is widely distributed in coastal areas of China and other coastal countries. The muscles of O. oratoria mainly constitute of myofibrillar proteins containing various trace elements and structure-balanced amino acids with extremely high nutritional value.[Citation1,Citation2] Besides consuming as fresh meat, O. oratoria shrimps are mainly sold as dried products after shelling. However, the traditional methods of shelling have many drawbacks including poor efficiency, low completeness, and excessive use of chloramphenicol.[Citation3] Therefore, it is necessary to explore more efficient shelling methods for high-quality O. oratoria products.
Ultra-high pressure (UHP) technology can inactivate microorganisms and enzymes while maintaining the original flavor, color, and nutrients of the food.[Citation4] Therefore, it has become a new food processing technology that can effectively assist in shelling shrimp and shellfish. Jantakoson, Kijroongrojana, & Benjakul[Citation5] reported that changes in weight loss, texture, and color of black tiger shrimp treated by high-pressure (200, 400, 600, and 800 MPa for 20 min at 28°C) were significantly lower than that of the samples applying for heat treatment (100°C for 2 min). Gómez-Estaca, Montero, Fernández-Martín, Calvo, & Gómez-Guillén[Citation6] reported that denaturation of hemocyanin by high-pressure decreased the activity of active polyphenol oxidase (PPO) thereby extend the shelf life of shrimp.
Since myofibrillar protein is the principal component of O. oratoria muscle, its conformational and rheological changes influence the properties of shrimp protein and shrimp-related foods. Earlier studies showed that UHP treatment could change the structure (conformation) and properties (physicochemical and rheological) of myofibrillar proteins due to the change of chemical bonding and volume.[Citation7] Zhang et al.[Citation7] reported protein surface hydrophobicity and formation of disulfide bonds increased with increase in pressure (0.1–500 MPa). Ahmed, Al-Ruwaih, Mulla, & Rahman[Citation8] found that oscillatory rheological measurement of the high pressure-treated kidney bean protein isolate (KBPI) dispersions revealed significant increase in storage modulus (G’) and energy consumption modulus (G’’) with the increase in frequency; a distinct thermal denaturation temperature (Td) was also observed. Therefore, it is very important to study the effects of the structure and rheological properties of O. oratoria muscle myofibrillar protein (MP) by ultra-high pressure.
Currently, research on the rheological properties and structure of myofibrillar protein in aquatic products mainly focuses on marine fish surimi. To the best of our knowledge, no publications or reports have documented about the effects of UHP treatment on the dynamic rheological properties and structure of MP. Since O. oratoria shrimp meat is widely consumed as sushi meat, it is, therefore, important to optimize the UHP processing conditions so that the nutrients remain intact. This study aims to shed light on the effect of UHP (300–400 MPa) on the rheological properties and structure of MP by SDS-PAGE, chemical bonds and circular dichroism (CD).
Materials and methods
Materials
Freshly caught samples of O. oratoria were purchased from Ningbo Lorin Aquatic Products Market, Ningbo, China. The fresh samples, which were covered with ice, were quickly transported to the laboratory. The average weight and length of the O. oratoria were 21 ± 4 g and 13 ± 3 cm, respectively.
UHP treatment
After cleaning, the fresh O. oratoria shrimps were kept in polyethylene (PE) bags (five shrimps in 150 mL water per bag) and sealed. The PE bags were then treated using high-pressure equipment (CQC2L-600, Beijing Speedway Zhongtian Co., Ltd., Beijing, China) with different pressure 300 MPa, 350 MPa and 400 MPa applied for 5 min, 6.5 min, and 8 min, respectively, at 18 ± 2°C. The pressure come-up time was 1 min, adiabatic temperature was 18 ± 2ºC, and depressurization time was 5 s. Samples were treated under nine different UHP conditions: 300 MPa −5 min, 300 MPa −6.5 min, 300 MPa −8 min, 350 MPa −5 min, 350 MPa −6.5 min, 350 MPa −8 min, 400 MPa −5 min, 400 MPa −6.5 min, and 400 MPa −8 min; for comparison, O. oratoria shrimps in the control group kept untreated.
Preparation of MP solution
The MP was extracted following the method reported by Lin et al.[Citation9] with slight modification. Briefly, 10 mL pre-cooled (4°C) Tris-maleate buffer solution containing KCl (50 mM KCl-20 mM Tris-maleate, pH 7.0) was mixed with 1 g O. oratoria meat. After homogenization, the mixture was centrifuged (12,500 g, 4°C, 10 min). The precipitate was then collected, mixed with 10 mL of Tris-maleate buffer (0.6 M KCl-20 mM Tris-maleate, pH 7.0), homogenized and kept in a refrigerator at 4°C for 1 h. The MP solution was finally obtained as the supernatant after centrifuging the above mixture at 12,500 g at 4°C for 10 min.
Rheological properties
Rheological properties were analyzed following a published method with slight modification.[Citation10] The sample was prepared as follows: (i) 1.75 ml of MP solution (1.84 mg/mL) was added into the bottom plane of a mold (a 20 mm diameter plate clamp with a parallel plate spacing at 1 mm), (ii) the mold was sealed with paraffin film to prevent moisture evaporation during scanning under different temperature conditions, and (iii) the excess sample was scraped off by paper for frequency scanning. After preparing the sample as above, the frequency sweep and temperature scanning were performed. For frequency sweep: the frequency range was 0.1–20 Hz (1 Hz = 2π rad/s), and the temperature was kept at 20°C. For temperature scanning: the sample was heated from 20°C to 85°C at a rate of 2°C/min, held at 85°C for 2 min, and then cooled down to 20°C at a rate of 5°C/min; the oscillation frequency was 1 Hz.
Sodium dodecyl sulfate–polyacrylamide gel electrophoresis (SDS-PAGE)
The SDS-PAGE was performed following the method by Laemmli[Citation11] with modification. The sample was prepared by mixing 30 μL of MP (1.2 mg/mL) solution with 10 μL buffer containing SDS, buffered saline solution, DTT and bromophenol blue. The solution was then heated in a boiling water bath for 10 min followed by centrifuging at 3,100 g for 5 min, and the supernatant was collected. Each sample (10 µL) was loaded onto the polyacrylamide gel (separation gel of 15% acrylamide and concentration gel of 5% acrylamide) and subjected to electrophoresis at 100 V and 170 mA. The polyacrylamide gels were stained with Coomassie Brilliant Blue and then destained in water.
Determination of chemical bonds
Chemical bonds present in O.oratoria muscle were analyzed following the reported methods.[Citation12,Citation13] Five different solutions were made by mixing 1 g of O. oratoria muscle with 5 mL solutions of A, B, C, D, E separately, where A, B, C, D, and E were 0.05 mol.L−Citation1 NaCl, 0.6 mol.L−Citation1 NaCl, a mixture of 0.6 mol.L−Citation1 NaCl and 1.5 mol.L−Citation1 urea, a mixture of 0.6 mol.L−Citation1 NaCl and 8 mol.L−Citation1 urea, and a mixture of 0.6 mol.L−Citation1 NaCl, 8 mol.L−Citation1 urea and 0.05 mol.L−Citation1 β-mercaptoethanol, respectively. After stilling on the stand at 4°C for 1 h, samples were centrifuged at 12,500 g for 15 min. The protein content in the supernatant was then determined by Folin-phenol. Ionic bond, hydrogen bond, hydrophobic interaction, and disulfide bond were calculated by the protein content difference between solution B and solution A, difference between solution C and solution B, difference between solution D and solution C, and difference between solution E and solution D, respectively.
Circular dichroism (CD)
A 0.17 mL MP solution was taken into a 1 mm quartz cuvette, and the CD spectrum was recorded in the wavelength range of 190–250 nm with a scanning speed of 100 nm/min at 25°C (± 1°C) using a J-1500–150 CD spectrometer (Jasco J-1500–150, Jasco Corp., Tokyo, Japan). The measurement response time was 1 s. CD was represented by the average residue molar elliptic value [θ]×10−Citation3, and the unit was deg·cm Citation2·dmol−Citation1. After obtaining the CD spectrum, the experimental data were estimated using secondary structure analysis software following Yang’s method.[Citation14]
Statistical analysis
All tests were performed thrice parallelly, and the results were expressed as mean ± SD. Statistical analysis was performed by one-way analysis of variance (ANOVA), and significant differences were analyzed by Duncan multiple comparisons (P < .05) using software SAS8.1 (SAS Campus Drive, Cary, NC USA). The results were considered statistically significant at P < .05.
Results and discussion
Rheological properties
Effect of frequency on the complex viscosity of MP
shows a significant decrease (P < .05) in the complex viscosity of MP with increasing angular frequency. This could be due to the appearance of shear thinning which caused by the orientation of protein molecular principal axis along shear flowing direction and the form of oligomers by non-covalent bonds dissociate into monomers during shearing. However, when the angular frequency reached 12 rad/s, a slight change in the complex viscosity was observed. The results can be explained as follows. The MP contains polymeric colloidal particles, which are composed of long molecular chains, and at lower flow rates or at rest, these polymer chains get entangled with each other, thus resulting in high viscosity. On the other hand, the mutual hooking, rolling and rotating of these relatively dispersed chain-like particles get inhibited since they shrink into a block by the shear stress between the flow layers at higher flow rate, thus resulting in decreasing viscosity. In addition, the viscosity of MP treated by UHP was lower than that of the control group (P < .05). The viscosity decreased with increasing pressure and holding time; a decrease by 58.82% was noted when the pressure reached 400 MPa (8 min) and angular frequency 100 rad/s. Saricaoglu, Gul, Tural, & Turhan[Citation15] found similar changes in the rheological properties of mechanically deboned chicken meat proteins treated by high-pressure homogenization. Similar results have also been reported for soy protein, where the authors suggested[Citation16,Citation17] that the decrease in viscosity resulted from UHP treatment, because such treatment degraded protein molecules, destroyed chemical bonds, increased hydrophobic interaction and electrostatic interaction, thereby enhancing homogenization that led to the increase of fluidity.
Effect of frequency on the elastic modulus of MP
Instead of steady shear, studying oscillatory shear of small strain or stress can shed more light on the viscoelastic properties of a material. During dynamic viscoelastic measurements, a continuous sinusoidal stress or strain is applied to the material, and then the response is recorded. The storage modulus G’ (also known as the elastic modulus), an elastic characteristic of a material, reflects the formation of the protein gel network structure. The energy consumption modulus G”, also referred to as the viscous modulus, is the viscous characteristic of a material. Generally, the loss tangent (tan δ), the ratio of the energy consumption modulus G” to the storage modulus G’, is used to characterize the viscoelasticity of a system; tan δ < 1 indicates a solid system, where the elastic component dominates; tan δ > 1 indicates a fluid system, where the viscous component predominates.[Citation18,Citation19]
As shown in , the storage modulus G’ and slopes of all samples increased significantly (P < .05) with increasing frequency. At lower flow rates or at rest, tan δ, which was high and close to 1, decreased significantly with increasing frequency within 0–2 Hz (P < .05); however, no significant change was noted when the frequency reached 5 Hz (P > .05). Fluid properties were observed at lower flow rates or at rest, and then the samples gradually started showing distinct solid properties from fluid properties at the frequencies greater than 4 Hz. As the flow rate increased, the elastic properties gradually dominated the viscoelasticity properties, and the gel state appeared, which was affected by frequency. Changes in viscoelasticity and fluidity of the MP samples were due to the changes in particle size and sample homogenization caused by shear stress, and this in turn affected the mutual friction and inertial resistance between the particles and soluble molecules, resulting in the changes in storage modulus G’ and tan δ.[Citation20,Citation21] Additionally, the G’ and tan δ of the samples under UHP treatment were smaller than that of the control group (P < .05), and both decreased further as the pressure and holding time increased. When the pressure reached 400 MPa (8 min) and frequency reached 20 Hz, G’ decreased by 73.47% and tan δ decreased by 69.12% which was close to zero. As the frequency increased further, the samples exhibited more pronounced solid properties, which is in agreement with the previously published reports.[Citation15,Citation21] It was attributed to the alternation in chemical bonds caused by UHP treatment, affecting the intermolecular interaction, the apparent viscosity, and the inertial resistance; which in turn decreases the free volume and stabilizes electrostatic interactions.[Citation22]
Effect of temperature on the viscoelasticity of MP
presents the effect of temperature on the viscoelasticity of MP samples. A steady increase in G’ initially with increasing temperature was found till G’ reached to the maximum peak; thereafter, a drop in G’ value was observed until a certain temperature, and G’ started increasing again with increasing temperature within 20–85°C. For the control, the maximum G’ was attained at 47°C. As for the UHP groups, with the increase in pressure and its holding time, the temperature, at which the maximum G’ attained, gradually increased, and the peak height decreased significantly. As temperature increased, initially tan δ increased and reached maximum value at around 32°C and then decreased with further increase in temperature; the value was much smaller than 1. These findings indicated that the samples achieved their highest elastic properties as G’ reached to a maximum value, and the proteins were held together by the action of chemical bonds to form gel.[Citation23] As the temperature increased, the protein’s spiral-like structure was gradually destroyed probably because of the disruption of hydrogen bonds, which in turn produced a certain degree of fluidity as the protein networks got separated[Citation23], thus resulting in the decrease in G’. However, when the temperature exceeded 70°C, G’ started to increase significantly (P < .05), and this increase was attributed to the rapid denaturation of proteins caused by high temperatures. At higher temperature, the number of cross-links between proteins or chains increases, resulting in the deposition of denatured proteins and the enhancement of gel-form.[Citation22] Our results indicate that the fluidic properties of the samples enhance with the increase in temperature. Cando, Herranz, Borderías, & Moreno[Citation24] reported similar observation and attributed such changes to the continual denaturation of myosin and actin.
Figure 3. Effect of temperature on the storage modulus G’ and tan δ of MP under different UHP conditions
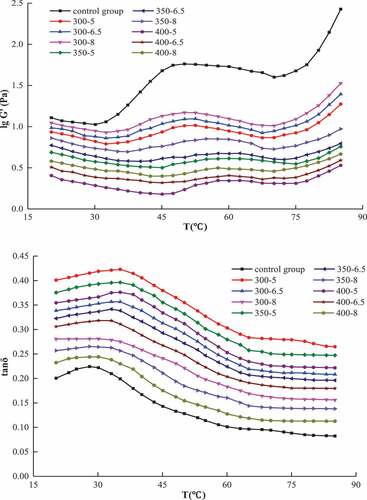
While studying the effect of pressure on the viscoelasticity of MP, it was found that the G’ of the samples in the control group was much higher than that of the UHP groups, which varied in the range of 1.026–2.427. Decrease in G’ was observed, varied from 0.180 to 0.529, when the pressure reached 400 MPa (5 min). Compared with the samples in the control group, the variation range of G’ in UHP groups was significantly reduced, and the upper and lower limits became smaller. Xue et al.[Citation25] reported similar observations regarding the effect of high pressure on the physicochemical and functional properties of myofibrillar proteins. The tan δ of all samples in the UHP group was larger than that of the control group, which decreased with the increase in pressure and holding time. This is in agreement with the published report by Zhang, Yang, Zhou, Zhang, & Wang.[Citation7]
Protein profiles
illustrates the SDS-PAGE profiles of MP. Followings are the main myofibrillar protein bands appeared: myosin heavy chain (MHC, 180 kDa), actin (45 kDa), myosin light chain (MLC 1–3, 17 kDa, 18 kDa, 20 kDa), and a protein band at a molecular weight of about 72 kDa. The profile is consistent with the previous reports.[Citation6,Citation26] A slight aggregation and degradation trend was observed in the UHP groups with increasing pressure and holding time. The formation of polymer by UHP led to the decrease of the main components, such as MHC. Then they degraded with increasing pressure and holding time. Actin and MLC were also degraded to different extents, especially, when the pressure reached 400 MPa (8 min). Interestingly, there was no significant difference in the protein band at a molecular weight of about 72 kDa, it might be because of the aggregation of protein. The results above are consistent with the explanation of rheological properties () which showed protein denaturation induced by UHP. Martínez et al.[Citation27] studied the effects of high-pressure processing on the protein fractions of blue crab (Callinectes sapidus) meat, they found that the myosin band of the intensity of the 250 kDa significantly decreased with increasing pressure, similar to our findings for shrimp meat (O. oratoria).
Chemical bonds
describes the changes of chemical bonds in MP while the shrimp meat is processed with high pressure. It was found that the ionic bonds in MP (in the UHP group) increased slightly at 300 MPa (with 8 min holding time) with a decreasing trend as pressure and holding time increased (P < .05). The hydrogen bonds also decreased with the increase in pressure and holding time. Both ionic bond and hydrogen bond were destroyed due to the reduction in volume under UHP treatment.[Citation28] The weakening effect of UHP on ionic bonds and hydrogen bonds was also mentioned by Wang, Ge, Chen, Xu, & Zhou[Citation14] when they studied the effect of UHP treatments on the activity and secondary structure of metmyoglobin reductase. Hydrophobicity, one of the leading forces of maintaining protein structure, plays an important role in the stability and functional properties of protein. As pressure and holding time increased, it was observed that the hydrophobic force increased slightly, while the disulfide bond increased significantly. The formation of disulfide bonds could reduce the conformational entropy of proteins and stabilize the structure of proteins to achieve good thermal stability.[Citation29] The UHP treatment changes the structure of the protein by releasing the embedded hydrophobic groups and sulfhydryl groups onto the surface. The exposed sulfhydryl groups then react with oxygen in the air and form disulfide bonds completely, thus resulting in increasing hydrophobicity and disulfide bonds.[Citation28] The formations of crosslinks and non-covalent molecular interactions, especially disulfide bonds and hydrophobicity within protein molecular network under UHP treatment, making the reformation of intermolecular bonds within or between protein molecules.[Citation30] These changes led to the aggregation of protein, which explained the protein band at about 72 kDa had no significant differences in .
CD spectra
In a CD spectrum of a protein, the absorption peaks in the range of 190–250 nm are attributed to the n–π* electronic transition of the peptide bond, which contains conformational information of protein main chain, i.e., the secondary structure information of a protein. The positive absorption peaks and negative absorption peaks are observed in the wavelength range of 190–202 nm and 202–250 nm, respectively. These absorption peaks are characterized as α-helix, β-sheet, β-turn, and random coil, and the changes in the secondary structure of a protein may cause the changes in absorption peaks in a CD spectrum.[Citation31] In our study, two opposite and trend peaks in the wavelength range of 190–250 nm in the CD spectrum () was found, wherein the peaks appearing in the wavelength range of 190–203 nm were positive. Decrease in the height and area of the peaks was also observed as the pressure and holding time increased. The peaks appearing in the range of 203–248 nm were negative, and were farther and farther from abscissa with the decreasing pressure and holding time, which indicated the height and area of the peaks decreased with the increase in pressure and holding time. These suggested that the secondary structure of the protein changed due to the UHP treatment, resulting in the changes in the peaks of the MP CD spectrum, which is in agreement with the study of Wang et al.[Citation14]
summarizes the contents of the secondary structure in MP. α-Helix, the main component of the secondary structure in MP, gradually decreased with increase in pressure and holding time (P < .05); when the pressure reached 400 MPa (8 min), it reduced by 20.10%. This could be due to the disruption of the hydrogen bonds and ionic bonds between the molecules caused by UHP treatment, weakening the interaction between molecules. Additionally, the hydrophobic binding and ion binding were also broken due to the reduction in volume, resulting in the unfolding of protein molecules and the exposure of non-polar groups.[Citation28] The β-Sheet content was significantly lower in the UHP group than that of the control group (P < .05), and it gradually increased with the increase in pressure and holding time (P < .05). This was because the chemical bonds between the molecules were broken by UHP treatment, causing partial unfolding of protein structure, resulting in the decreasing of β-sheet content. However, as the pressure and holding time increased, β-sheet content increased due to the aggregation of the protein.[Citation32] β-Turn content also increased significantly with the increase in UHP treatment (P < .05); however, there were no significant changes in random coil content (P > .05). UHP might make protein molecular re-organize into a more suitable structure to face the changes of external environment.[Citation33] A similar change in β-Turn and random coil was also reported for bullfrog skin collagen.[Citation34] The results of the secondary structure are consistent with the results obtained from the studies of rheological properties and chemical bonds.
Table 1. Calculated structural parameters of MP samples by UHP
Conclusion
On a quest to find the effect of UHP processing on mantis shrimp meat (O. oratoria), we extracted MP from O. oratoria muscle and found that the UHP treatment changed the rheological properties and structure of MP. The MP gradually degraded with the increase in pressure and holding time. The ionic bond and hydrogen bond were destroyed, while hydrophobic interaction and disulfide bond increased, which affected the intermolecular interactions, leading to the unfolding of MP molecule. The viscosity of MP treated by UHP was decreased, and shear thinning appeared when the frequency increased because of the changes in the sequence of amino acid residues and the destruction of the structure. The storage modulus G’ decreased firstly but increased later as the frequency increased, and the solid properties of MP became pronounced. Since the MP denatured with increase in temperature, the fluidity of samples increased. Moreover, α-helix, the main secondary structural component of MP, decreased as the pressure and holding time increased. In the UHP group, the contents of β-sheet and β-turn were lower and higher, respectively, than those of the control group. Additionally, the β-Sheet increased with the increase in pressure and holding time due to the aggregation of protein. These findings may help to optimize the UHP processing conditions to obtain high-quality shrimp meat or other processed meat for human consumption.
Acknowledgments
This work was supported by the National Key R&D Program of China (2018YFD0901105), the Public Welfare Technology Research Project of Zhejiang Province (LGN18C200020), grants from the Major Science and Technology projects of Ningbo (2015C110002), and Research Project of Zhejiang Education Department (Y201839330) sponsored by K. C. Wong Magna Fund in Ningbo University. The authors also thank the relevant researchers for their references.
Additional information
Funding
References
- Yan, H., Cui, X., Shen, X., Wang, L., Jiang, L.; Liu, H.; Liu, Y.; Liu, Q.; Jiang, C. De Novo Transcriptome Analysis and Differentially Expressed Genes in the Ovary and Testis of the Japanese Mantis Shrimp Oratosquilla Oratoria by Rna-seq. Comparative Biochemistry & Physiology Part D. Genomics Proteomics. 2018, 26, 69–78.
- Morita, M.;. Reproductive Biology of the Japanese Mantis Shrimp (crustacea Stomatopoda): Annual Cycle of Gonadal Development and Copulation. Mar. Biol. Res. 2009, 5(5), 415–426. DOI: 10.1080/17451000802644714.
- Wang, Z.; Yang, W.; Zhou, G.; Xu, D.; Lou, Q.; Zhang, J.; Cui, Y. Shelling of Solenocera Melantho Using Ultra High Pressure and Its Effect on the Quality of Muscle. Food Sci. 2017, 38(07), 43–48.
- Xi, X. X.; Xu, B. C.; Xu, S. C.; Liu, T.; Huang, J. Effects of Ultra-high Pressure Processing on Survival of Microorganisms in Meat Products and Microbial Inactivation Mechanisms: A Review. Meat Res. 2016, 30(8), 39–43.
- Jantakoson, T.; Kijroongrojana, K.; Benjakul, S. Effect of High Pressure and Heat Treatments on Black Tiger Shrimp (penaeus Monodon Fabricius) Muscle Protein. Int. Aquat. Res. 2012, 4(1), 1–12. DOI: 10.1186/2008-6970-4-19.
- Gómez-Estaca, J.; Montero, P.; Fernández-Martín, F.; Calvo, M. M.; Gómez-Guillén, M. C. The Effect of High-pressure Treatment on Functional Components of Shrimp (litopenaeus Vannamei) Cephalothorax. Innovative Food Sci. Emerg. Technol. 2016, 34, 154–160. DOI: 10.1016/j.ifset.2016.01.017.
- Zhang, Z. Y.; Yang, Y. L.; Zhou, P.; Zhang, X.; Wang, J. Y. Effects of High Pressure Modification on Conformation and Gelation Properties of Myofibrillar Protein. Food Chem. 2017, 217, 678–686. DOI: 10.1016/j.foodchem.2016.09.040.
- Ahmed, J.; Al-Ruwaih, N.; Mulla, M.; Rahman, M. H. Effect of High Pressure Treatment on Functional, Rheological and Structural Properties of Kidney Bean Protein Isolate. LWT- Food Sci. Technol. 2018, 91, 191–197. DOI: 10.1016/j.lwt.2018.01.054.
- Lin, X.; Zhen, J. I.; Shu, Y.; Yang, W.; Xu, D. L.; Yan, X. Cryoprotective Effects of Mackerel Hydrolysate Addition on the Hairtail Surimi during Frozen Storage. J. Nucl. Agric. Sci. 2015, 29(5), 940–945.
- Delgado-Pando, G.; Cofrades, S.; Ruiz-Capillas, C.; Triki, M.; Jiménez-Colmenero, F. Low-fat Pork Liver Pâtés Enriched with N-3 Pufa/konjac Gel: Dynamic Rheological Properties and Technological Behaviour during Chill Storage. Meat Sci. 2012, 92(1), 44–52. DOI: 10.1016/j.meatsci.2012.04.002.
- Laemmli, U. K.;. Cleavage of Structural Proteins during the Assembly of the Head of Bacteriophage T4. Nature. 1970, 227, 680–685. DOI: 10.1038/227680a0.
- Gómez-Guillén, M. C.; Borderı́As, A. J.; Montero, P. Chemical Interactions of Non-Muscle Proteins in the Network of Sardine (sardina Pilchardus) Muscle Gels ☆. LWT - Food Sci. Technol. 1997, 30(6), 602–608. DOI: 10.1006/fstl.1997.0239.
- Careche, M.; Alvarez, C.; Tejada, M. Suwari and Kamaboko Sardine Gels: Effect of Heat Treatment on Solubility of Networks. J.Agric.Food Chem. 1995, 43(4), 1002–1010. DOI: 10.1021/jf00052a030.
- Wang, W.; Ge, Q.; Chen, Y.; Xu, X. L.; zhou, G. H. Effect of Ultra-high Pressure Treatments on the Activity and Secondary Structure of Metmyoglobin Reductase (metmbase). J. Chin. Inst. Food Sci. Technol. 2015, 15(10), 134–140.
- Saricaoglu, F. T.; Gul, O.; Tural, S.; Turhan, S. Potential Application of High Pressure Homogenization (hph) for Improving Functional and Rheological Properties of Mechanically Deboned Chicken Meat (mdcm) Proteins. J. Food Eng. 2017, 215, 161–171. DOI: 10.1016/j.jfoodeng.2017.07.029.
- Song, X.; Zhou, C.; Fu, F.; Chen, Z.; Wu, Q. Effect of High-pressure Homogenization on Particle Size and Film Properties of Soy Protein Isolate. Ind. Crops Prod. 2013, 43(1), 538–544. DOI: 10.1016/j.indcrop.2012.08.005.
- Ahmed, J.; Thomas, L.; Arfat, Y. A.; Joseph, A. Rheological, Structural and Functional Properties of High-pressure Treated Quinoa Starch in Dispersions. Carbohydr. Polym. 2018, 197, 649–657. DOI: 10.1016/j.carbpol.2018.05.081.
- Zhao, Y. L.; Wang, J. B.; Shan, L. M.; Jin, C.; Ma, L.; Xiao, X. H. Effect of Radix Isatidis, Polysaccharides on Immunological Function and Expression of Immune Related Cytokines in Mice. Chin. J. Integr. Med. 2008, 14(3), 207–211. DOI: 10.1007/s11655-008-0207-2.
- Yong, L. I.; Cheng, Y. D. Rheological Properties of Silver Carp Surimi. Food Sci. 2007, 28(10), 100–104.
- Leite, T. S.; Augusto, P. E. D.; Cristianini, M. Processing Frozen Concentrated Orange Juice (fcoj) by High Pressure Homogenization (hph) Technology: Changes in the Viscoelastic Properties. Food Eng. Rev. 2014, 7(2), 1–10.
- Gul, O.; Saricaoglu, F. T.; Mortas, M.; Atalar, I.; Yazici, F. Effect of High Pressure Homogenization (hph) on Microstructure and Rheological Properties of Hazelnut Milk. Innovative Food Sci. Emerg. Technol. 2017, 41, 411–420. DOI: 10.1016/j.ifset.2017.05.002.
- Moreno, H. M.; Bargiela, V.; Tovar, C. A.; Cando, D.; Borderias, A. J.; Herranz, B. High Pressure Applied to Frozen Flying Fish (parexocoetus Brachyterus) Surimi: Effect on Physicochemical and Rheological Properties of Gels. Food Hydrocolloids. 2015, 48(4), 127–134. DOI: 10.1016/j.foodhyd.2015.01.029.
- Qiu, C.; Xia, W.; Jiang, Q. Effect of High Hydrostatic Pressure (HHP) on Myofibril-bound Serine Proteinases and Myofibrillar Protein in Silver Carp (hypophthalmichthys Molistrix). Food Res. Int. 2013, 52, 199–205. DOI: 10.1016/j.foodres.2013.03.014.
- Cando, D.; Herranz, B.; Borderías, A. J.; Moreno, H. M. Effect of High Pressure on Reduced Sodium Chloride Surimi Gels. Food Hydrocolloids. 2015, 51, 176–187. DOI: 10.1016/j.foodhyd.2015.05.016.
- Xue, S.; Xu, X.; Shan, H.; Wang, H.; Yang, J.; Zhou, G. Effects of High-intensity Ultrasound, High-pressure Processing, and High-pressure Homogenization on the Physicochemical and Functional Properties of Myofibrillar Proteins. Innovative Food Sci. Emerg. Technol. 2018, 45, 354–360. DOI: 10.1016/j.ifset.2017.12.007.
- Chen, X.; Zhou, R.; Xu, X.; Zhou, G.; Liu, D. Structural Modification by High-pressure Homogenization for Improved Functional Properties of Freeze-dried Myofibrillar Proteins Powder. Food Res. Int. 2017, 100(Pt 1), 193–200. DOI: 10.1016/j.foodres.2017.07.007.
- Martínez, M. A.; Velazquez, G.; Cando, D.; Núñez-Flores, R.; Borderías, A. J.; Moreno, H. M. Effects of High Pressure Processing on Protein Fractions of Blue Crab (callinectes Sapidus) Meat. Innovative Food Sci. Emerg. Technol. 2017, 41, 323–329. DOI: 10.1016/j.ifset.2017.04.010.
- Cao, Y. Y.; Zhang, L.; Wang, P.; Zhou, G. H.; Xing-Lian, X. U. Combined Effect of Ultra High Pressure and Heating on Gel Properties and Secondary Structure of Myosin. Meat Res. 2013, 27(1), 1–7.
- Bulaj, G.;. Formation of Disulfide Bonds in Proteins and Peptides. Biotechnol. Adv. 2005, 23(1), 87–92. DOI: 10.1016/j.biotechadv.2004.09.002.
- Cao, Y.; Xia, T.; Zhou, G.; Xu, X. The Mechanism of High Pressure-induced Gels of Rabbit Myosin. Innovative Food Sci. Emerg. Technol. 2012, 16(39), 41–46. DOI: 10.1016/j.ifset.2012.04.005.
- Ding, X. L.; Gao, H. Q. Applications and Experimental Methods of Circular Dichroism Spectroscopy. Exp. Technol. Manage. 2008, 25(10), 48–52.
- Saricaoglu, F. T.; Tural, S.; Gul, O.; Turhan, S. High Pressure Homogenization of Mechanically Deboned Chicken Meat Protein Suspensions to Improve Mechanical and Barrier Properties of Edible Films. Food Hydrocolloids. 2018, 84, 135–145. DOI: 10.1016/j.foodhyd.2018.05.058.
- Feng, Y.; Lee, Y. Microfluidic Fabrication of Wrinkled Protein Microcapsules and Their Nanomechanical Properties Affected by Protein Secondary Structure. J. Food Eng. 2018, 246, 102–110. DOI: 10.1016/j.jfoodeng.2018.10.028.
- Nan, J.; Zou, M.; Wang, H.; Xu, C.; Zhang, J.; Wei, B.; He, L.; Xu, Y. Effect of Ultra-high Pressure on Molecular Structure and Properties of Bullfrog Skin Collagen. Int. J. Biol. Macromol. 2018, 111, 200–207. DOI: 10.1016/j.ijbiomac.2017.12.163.