ABSTRACT
The binding capacity of Pb on modified rice bran-insoluble fibers RBIF-0.2, RBIF-1.25, and RBIF-2.0 (0.2%, 1.25%, and 2.0% H2SO4 with 1.25% KOH) having the variable specific surface area and cation-exchange capacity was evaluated. The Pb binding in terms of maximum binding capacity (BCmax) with RBIF increased with pH (2–7). However, the presence of sodium and calcium reduced the binding of Pb to RBIFs. Further, adsorption kinetics followed pseudo-second-order kinetic model when fitted to real Pb sorption data. However, sorption mechanism data (scanning electron microscopy images) were well fitted to Langmuir model instead of Freundlich and Dubinin–Radushkevich that suggests monomolecular adsorption of Pb to RBIF (<8 kJ/mol). Moreover, negative ΔG and positive ΔH of Pb2+ sorption confirmed the occurrence of spontaneous and endothermic process. The modeling of single-metal (Pb) data will be advantageous to use as a universal model for other heavy metals that will save the experimental costs. The data on all other heavy metals could be used with chosen models to elucidate their sorption mechanism to control the metallic-induced toxicity.
Introduction
Heavy metal pollution of wastewater is a common environmental threat since the toxic metal ions dissolved can eventually reach the top of the food chain and thus become a risk for human health. Various researchers reported metal-binding capacities of cellulosic fibers that can avoid their dietic toxicity.[Citation1,Citation2] Cellulose and lignin could bind with heavy metals, which are dependent on their source of origin and relevant fraction of various constituents.[Citation3–Citation5] Similarly, a group of researchers reported that hemicellulose and pectin also could bind heavy metals (Pb, Cu, and Cd) that can improve health via fiber-containing food.[Citation6] Various researchers investigated dietary fiber in terms of its potential to scavenge heavy metals after it enters the body[Citation7–Citation9] and to elucidate the dietic factors that could affect the heavy metal-binding capacity, some endogenous factors like proteins and exogenous factors like pH.[Citation10,Citation11]
Rice bran is an abundant, low-cost, underutilized lignocellulosic material and has usually been discarded directly or used as feed without effective utilization. The heavy metal adsorption capacity of rice bran-insoluble fiber (RBIF) has not been reported till to date. Therefore, our current study will enhance its usage in the industry as a heavy metal toxicity reducer. The proper cation-exchange capacity (CEC) of reactive hydroxyl and carboxyl groups on the RBIF has been confirmed in our previous work.[Citation12] Other researchers also proved the binding capacity of rice bran fiber that made this agriculture waste a source of potential heavy metal scavenger.[Citation13,Citation14]
Therefore, to reduce the harmful effects of food-derived lead (Pb2+) on humans, the water-insoluble dietary fibers extracted from rice bran were used. In order to find out the best adsorbent for Pb and illuminate the adsorption process, three RBIFs using different treatments were comparatively investigated. The effects of different acid–alkaline treatments on the structure and properties of RBIF have been reported in our previous work.[Citation12] In spite of structure, the binding capacities of RBIF toward Pb2+ as a function of pH and other relevant factors including the presence of essential metal ions were also investigated. Further, modeling of adsorption mechanism experimental data was checked through kinetic and adsorption isotherm to achieve a universal model for other heavy metals too. Moreover, the thermodynamic parameters were also used to identify the adsorption behavior. The Pb sorption model will provide universal key for other heavy metals available in the literature to evaluate their sorption mechanisms to fibers without further experimental procedures. The safe disposal of the used adsorbent will be done in the future.
Materials and methods
Preparation of dietary fiber
Rice bran was obtained from small rice processing mills situated in Wuxi city, Jiangsu province, China. They were passed through a 40-mesh sieve, followed by defatting (using n-hexane, 1:5, w/v) and then removal of starch (using sulfuric acid) and protein (using potassium hydroxide) according to the methods described in our previous work.[Citation12] The residues obtained as the rice bran-insoluble dietary fibers were sequentially dried in an oven at 60°C for 12 h and were ground with a high-speed universal grinder (DFY-200, Linda Machinery Co., Zhejiang, China).
Characterization of the modified RBIF
Hemicellulose and cellulose contents were determined according to the procedure described by Sun et al.[Citation15] and Egüé et al.[Citation16] Specific surface area measurements were determined by an automatic Brunauer, Emmett and Teller (BET) surface area analyzer (ASAP 2020 MP; Micromeritics Instrument Corp., Atalanta, Georgia State, USA). The CEC was measured according to the procedure described by our previous work.[Citation12]
Scanning electron microscope (SEM) observation was carried out by the equipment of Quanta-200 (FEI Co., The Netherlands) with an accelerating potential of 5.0 kV and magnifications of 600 and 300. The RBIF powders were deposited on a metal stub, coated with a thin layer of gold (approximately 30 Å) in a vacuum for 30 s by an ion sputter. Then, the surface and microstructure were observed by the SEM.
Maximum binding capacity (BCmax) of RBIF to Pb2+
The maximum binding capacity (BCmax) was determined according to the methods reported by Ou et al;[Citation7] 2.0 g RBIF was mixed with 200 mL Pb2+ standard solution (10 mmol/L Pb(NO3)2) at the pH 2 and 7 to simulate the stomach and small intestine, respectively. The slurries were stirred (120 rpm) for 3 h in a water-bath incubator maintained at 37°C. At the end of adsorption, 2 mL sample was collected and centrifuged at 4,000 × g for 20 min. The concentrations of Pb in the supernatant were determined by inductively coupled plasma-atomic emission spectroscopy (AA-240FS, USA). The maximum adsorption capacity of RBIF for Pb2+ was calculated according to the following equation:
IC: initial concentration of Pb2+, mmol/L; EC: equilibrium concentration of Pb2+, mmol/L; V: total volume of solution, mL; W: the weight of the sample, g
Competition between some essential minerals and Pb2+
RBIF (0.5 g) was mixed with 25 mL 10 mmol/L CaCl2/Pb(NO3)2 and NaCl/Pb(NO3)2 standard solutions at pH 7, respectively. Slurries were shaken (120 rpm) for 3 h in acid-washed bottles at 37°C. The complexes of dietary fiber and minerals were centrifuged at 4,000 rpm for 20 min. Two milliliters of supernatant were collected, and the concentrations of Pb in the supernatant were determined by inductively coupled plasma-atomic emission spectroscopy (AA-240FS, USA).
Batch adsorption studies
Adsorption kinetic tests related to the effect of time on the Pb2+ adsorption by RBIF were performed in a series of 100-mL conical flasks containing 50 mL Pb(NO3)2 solutions (natural pH) with varying initial concentrations (5–25 mmol/L) and 0.5 g of adsorbent at 37 ± 1°C. At predetermined 10-min interval (30–240 min), 2 ml of each sample was taken, rapidly filtered with 0.45 μm membranes, and filtrate was analyzed. The amount of adsorbed Pb2+ at time t, qt (mg/g), was calculated using EquationEquation (2)(2) . All of the experiments were performed in duplicate, and the mean values were reported.
where qt is the adsorption capacity (mg/g) of adsorbent at time t; Co and Ct are the initial concentration and the concentration of Pb2+ at time t in solution (mmol/L), respectively; V is the volume of Pb2+ solution (L); and W is the mass of adsorbent used (g).
Pseudo-first-order kinetic model
The pseudo-first-order model is expressed as:
where qe (mg/g) and q are the amounts of adsorbed metal ions on the biosorbent at equilibrium at time t and k1 (per min) is the Lagergren rate constant of the first-order biosorption. qe and k1 can be calculated from the slopes and the intercept of the plot log(qe −q) versus t.
Pseudo-second-order kinetic model
Second, metal sorption to RBIF was mathematically modeled using pseudo-second-order kinetic model that has already been used.[Citation17]
where t (min) is the contact time and q (mg/g) and qe (mg/g) are the amounts of Pb ion sorbed at time t and in equilibrium state. However, K2 (g/mg/h) is the pseudo-second-order rate constant.
Intraparticle diffusion model
Third, intraparticle diffusion (relationship between specific sorption (qt) and the square root of time) was used to model the Pb sorption to RBIF that has already been used by Ren et al.[Citation17]
where qt is the amount of Pb adsorbed (mg/g) at time t, ki is the intraparticle diffusion rate constant (mg/g/min1/2), and I is the intercept of the plot qt versus t1/2. Intraparticle diffusion may be the rate-controlling step. If this does occur, then the plot of qt versus t1/2 should be linear.When the plot passes through the origin, it suggests that the intraparticle diffusion is the sole rate-limiting process.[Citation18]
Sorption isotherm modeling
Adsorption isotherms describe the interaction mechanism of metal ions (adsorbates) with adsorbents (e.g., Fiber) and are critical in optimizing the use of adsorbents. Modeling the equilibrium data of sorption provided opportunity to design and optimize the operating procedures for a variety of biomaterials under different conditions.[Citation19] Therefore, the equilibrium point of Pb adsorption onto RBIF was modeled using the Langmuir, Freundlich, and Dubinin–Radushkevich (D–R) isotherms.
The adsorption isotherm studies were carried out in 50 mL 20 mmol/L Pb(NO3)2 solution with 0.5 g RBIF, with temperature ranging from 298 to 310 K at neutral pH. After equilibrium, 10 mL of each sample was withdrawn and filtered through a 0.45-μm membrane filter. The filtrate was analyzed, and the amount of Pb2+ adsorbed by per unit mass of RBIF was also calculated by EquationEquation (2)(2) , in which the concentrations of Pb2+ solutions at equilibrium time, Ce (mmol/L), were substituted for Ct,
where qe is the equilibrium adsorption capacity (mg/g); Co and Ce are the initial and equilibrium concentrations of Pb2+ in solution (mmol/L), respectively; V is the volume of Pb2+ solution (L); and W is the mass of adsorbent used (g).
Langmuir adsorption model
To get the equilibrium sorption (Pb on RBIF) data, initial Pb concentrations were varied at constant biomass. Four hours of equilibrium periods of sorption experiments were used. The most widely used model for equilibrium adsorption is the Langmuir adsorption model, based on the sorption on the homogeneous surface as monolayer without interaction between sorbed species. Langmuir model assumes that the adsorptive forces are similar to the forces in chemical interaction. This model is described by the equation:
where Ce is the equilibrium aqueous metal ion concentration (mmol/L), qe is the amount of metal ions adsorbed per gram of adsorbent at equilibrium (mmol/g), and qmax and b are constants related to the maximum adsorption capacity (mmol/g) and the energy of adsorption (L/mmol), respectively. qmax represents the practical limiting adsorption capacity when the surface is fully covered with sorbed species. The Langmuir constants qmax and b can be determined from the linearized form of EquationEquation (6)(6) ; the plot of 1/qe versus 1/Ce results in a straight line of slope 1/bqmax and an intercept of 1/qmax.
Finally, for Langmuir-type sorption process, shape was classified using a dimensionless separation factor (r) as described by Ho and Wang[Citation20] as well as Khezami and Capart.[Citation21] The r is defined as
where r is the dimensionless separation factor, C0 is the initial metal concentration (mmol/L), and b is Langmuir constant (L/mmol). The parameter r indicates the shape of the isotherm accordingly: r > 1 unfavorable, r = 1 linear, 0 < r < 1 favorable, r = 0 irreversible.
Freundlich isotherm
Freundlich isotherm assumes that the uptake of metal ions occurs on a heterogeneous surface by multilayer adsorption and the amount of adsorbate adsorbed increases infinitely with an increase in concentration.[Citation22] The equilibrium data were analyzed using the following linearized equation:
where Kf is roughly an indicator of the adsorption capacity and 1/n is the adsorption intensity. Kf and 1/n can be determined from the linear plot of lnqe vs. lnCe. qe is the amount of heavy metals adsorbed (mg/g) on RBIF at equilibrium, and Ce is the heavy metal concentration (mmol/L) of solution at the equilibrium.
Dubinin–Radushkevich (D–R) isotherm
D–R isotherm is widely used to determine the nature of sorption.[Citation23] D–R model equation is given as:
where qm is the maximum amount of ion that can be sorbed onto unit weight of sorbent (mg/g), β is the constant related to sorption energy (mol2/kJ2), and ε is the Polanyi potential which is equal to RTln (1 + 1/Ce), where R and T are the gas constant (kJ/mol/K) and the absolute temperature (K), respectively. The plot of lnq vs. ε2 results in a straight line. The slopes of the D–R plots give β constant, and qm value is calculated from the intercept of the plot.
The mean free energy of sorption can be calculated from D–R isotherm parameter β as follows[Citation24]:
E is the energy required to transfer 1 mol of a sorbate to the surface from infinity in solution. The value of E is used to estimate the reaction mechanism occurring. If the magnitude of E is between 8 and 16 kJ/mol, the sorption process proceeds by ion exchange, while for values of E < 8 kJ/mol, the sorption process is a physical nature.[Citation25,Citation26]
Thermodynamic profiling analysis of Pb sorption to RBIF
To know whether the adsorption phenomenon was endothermic or exothermic, thermodynamic parameters such as enthalpy (ΔH◦), free energy (ΔG◦), and entropy (ΔS◦) changes were calculated using
where b is Langmuir constant related to the energy of adsorption, b0 is a constant, R the ideal gas constant (4.187 J/mol/K), and T is temperature (K).[Citation27] The enthalpy changes (ΔH◦) of the process were determined from the slope of the line obtained by plotting lnb vs. 1/T.
Results and discussion
Characteristics of modified RBIF
CEC is an important parameter for cation-binding capacity evaluation. Acid–alkaline (H2SO4 along with KOH)-treated RBIF having variable CEC was used to clarify the mechanism of metal adsorption. Different CEC may result in significant differences in metal adsorption capacities of fiber as evident by the findings of various researchers.[Citation28] The CEC data of used samples showed that the RBIF-0.2 exhibited the highest CEC for its proper net structure and higher hemicellulose content as our previous work confirmed.[Citation12]
However, the rate of sorption process depends on structural properties of the sorbent (e.g., porosity, specific area, and particle size) and on metallic ion characteristics/properties, e.g., ionic radius and number of coordination.[Citation14] Due to these characteristics, we evaluated specific surface area of RBIF. RBIF treated with a higher concentration of acid presented higher specific surface area as shown in . The specific surface area obtained at the highest acid–alkaline ratio was 0.67 m2/g. On the other hand, Betancur-Ancona et al.[Citation29] confirmed that ion affinity of fibrous material is linked to the cellulose content. In our case, RBIF-2.0 presented the highest cellulose content of 53.51 ± 0.06 g/100 g but showed low CEC. Further, Oliveira and Motanher[Citation14] pointed out the presence of several different active sites on the cellulose surface that orient metal removal.
Table 1. Physicochemical properties of RBIF
Pb2+-binding capacity of dietary fibers at acid and alkaline pH
Sorption of metal ions on dietary fiber (adsorbent) is strongly dependent on solution pH that ensures their solubility and made them available in ionic state which is suitable for surface adsorption.[Citation30,Citation31] In our case, RBIF-2.0 showed the BCmax to Pb at pH 7 () might be linked to porosity and greater specific surface area (). The BCmax to Pb at pH 7 was 241 ± 5.96 mg/g. On the other hand, BCmax at pH 2 was eightfold less (33 ± 1.58 mg/g) compared to neutral pH-binding capacity of RBIF. The possible reason behind low binding capacity/adsorption of Pb to RBIF is the availability of positive charge due to the presence of H+ ions that repulse the interaction of surface functional groups with metal ions.[Citation32] The current findings revealed the excellent adsorption of Pb with RBIF at small intestinal pH that might be a suggestion for the food industry to incorporate it in functional foods as nutrient source as well as a detoxification agent for toxic pollutant Pb.
Table 2. In vitro maximum binding capacity (BCmax) of RBIDF for Pb2+ at pH 2.0 and 7.0
Effect of some essential minerals on the binding capacity for Pb2+
Divalent and monovalent minerals such as Ca2+, Mg2+, and Na+ and structural change in fiber could affect the adsorption capacity of metal ions.[Citation27,Citation33] We, therefore, evaluated the possible effect of Ca2+ or Na+ on adsorption capacity of metal ions to the RBIF. As shown in , the BCmax of Pb with RBIF-0.2 in combination with Ca2+ reduced from 37 to 30 mg/g. However, in the case of Na+, this reduction was also comparable (37–29 mg/g). Similarly, both RBIF-1.25 and 2.0 showed the same decreasing trend in BCmax after interaction with Ca2+ or Na+. But, this reduction was significant with Na+ compared to Ca2+ (). These results were in accordance with the findings of Šćiban et al. who reported relatively higher influence of Na+ on lead (II) adsorption that was probably due to unfavorable pH and change in fiber structure which played important role in metal adsorption.[Citation34] In our case, RBIF-1.25 showed the highest values of BCmax, and it might be due to an appropriate porous structure that exposed functional groups required for metal adsorption. Thus, RBIF-1.25 might be a suitable candidate for the food industry to incorporate in functional foods as a nutraceutical as well as detoxicant in the diet.
Table 3. Effect of Na and Ca on maximum binding capacity (BCmax) for Pb
Effect of contact time on the sorption process
The kinetics of metal ion sorption is important for designing sorption systems that assist in selecting optimum operating conditions for full-scale batch process. Therefore, time-dependent adsorption of Pb2+ with RBIF was carried out. The maximum Pd adsorption (150–240 min) was achieved in RBIF-1.25 that was highly expected due to the optimal porous structure as shown in . is showing Pb2+ sorption (qt, mg/g) as a function of time, and the maximum adsorbed amount of Pb2+ for RBIF-0.2, RBIF-1.25, and RBIF-2.0 was achieved within 180, 150, and 140 min, respectively. For all samples, the sorption increased in the start and then gets steady.
Figure 2. Binding capacities of various RBIFs that produced with 0.2%, 1.25%, and 2.0% H2SO4 combined with 1.25% KOH for Pb2+ at different time
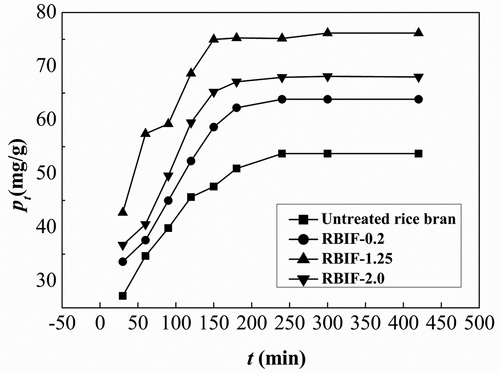
The possible reason behind this immediate adsorption and then no or negligible adsorption is the presence of holes in addition to porous structure (). The time to achieve adsorption equilibrium was shortest for RBIF-2.0, which might be due to its greater porosity. The maximum adsorption capacity for untreated rice bran was 53.73 mg/g at longer adsorption equilibrium time (240 min) than that of other samples that could be due to less exposed carboxylic and hydroxyl groups.[Citation14] As all RBIFs showed maximum equilibrium adsorption of Pb at 240 min, all the following experiments were conducted at this time duration.
Sorption kinetics
Sorption kinetic modeling
To investigate the mechanism of biosorption of Pb to modified RBIF and the potential rate-controlling steps, such as mass transport and chemical reactions, kinetic models were used to test the experimental data. Mathematical models that can describe the behavior of batch biosorption process operated under different experimental conditions are very useful for scale up process optimization. A number of models with varying degrees of complexity have been developed to describe the kinetics of metal biosorption in batch systems. According to the kinetic model selection criteria, proposed by Ren et al.[Citation17] and Gupta et al.[Citation35], several reaction-based and diffusion-based models were tested for the simulation of the obtained experimental data. The finally selected kinetic model will be that does not only fit closely the data but also represent reasonable sorption mechanism. To adjust the experimental data of Pb biosorption on RBIF, we choose three different kinetic models including pseudo-first-order, pseudo-second-order, and intraparticle diffusion equations.[Citation17]
In many cases, the pseudo-first-order kinetic model does not fit well to the whole range of contact time and is generally applicable at the initial stage of the adsorption process.[Citation36] The pseudo-second-order model is based on the assumption that the rate-limiting factor may be chemisorption involving valent forces through sharing of electrons between the amino group and metal ions.[Citation36] Generally, in a batch reactor, intraparticle diffusion is often rate-limiting. Weber and Morris proposed an empirically found functional relationship that if intraparticle diffusion is the rate-controlling factor, uptake varies with the square root of time.[Citation37]
is presenting the kinetics parameters and regression coefficients of the three models. Experimental qe values in the table show values at flat plateau region (). Pseudo-second-order kinetic model yielded best fits to the experimental data (). Besides the highest regression coefficients (>0.9), calculated qe values are so close to actual values for this model. On the other hand, pseudo-first-order kinetic model showed more closeness to experimental and calculated values, but their regression coefficients (<0.9) were less than the standard criteria. These results were in accordance with the findings of Wan et al.[Citation38] and Zhang et al.[Citation39] who reported the feasibility of pseudo-second-order kinetic model when measured the sorption of metal ions on fiber. However, intraparticle diffusion equation-based constructed plots of qt vs. t½ exhibited an almost initial linear portion followed by an almost plateau protion; these two portions suggest more than one mechanism behind sorption process[Citation27] as shown in . From these findings of modeling, what we can suggest the sorption of Pb on RBIF was adjusted to heterogeneous diffusion model. Moreover, our results maybe provide that as long as the material exists multilayer, the sorption kinetic for heavy metals almost accord with pseudo-second-order kinetic model.
Table 4. Kinetic parameters for the adsorption of Pb by RBIF
Sorption isotherm modeling for mechanistic evaluation
The calculated values of Langmuir, Freundlich, and Dubinin–Radushkevich (D–R) constants at different temperatures for the Pb sorption to RBIF have been presented in . The correlation coefficient values (R2) showed that Langmuir isotherm model (R2 = 0.99) was the best model for Pb adsorption. However, correlation coefficients of the other two models (<0.95), Freundlich and Dubinin–Radushkevich (D–R), were less than standard (>0.99). These results were in accordance with the findings of other researchers who also found the Langmuir model was the best when studied the adsorption of metal ions on fibers or biomaterials.[Citation40–Citation42] Further, the Langmuir constant b indicator of binding affinity increased with increasing temperature, suggesting the enhanced sorption capacity of Pb2+ with RBIF at a higher temperature. On the other hand, E values generated using Dubinin–Radushkevich (D–R) model (8 kJ/mol, 25–37 ◦C) confirmed the physical sorption behavior of Pb2+ with RBIF (). Similarly, the same trend of physical sorption for esterified lemon fiber to metal has already been observed by Arslanoglu and Altundogan[Citation27] using Dubinin–Radushkevich (D–R) model (E values) as an indicator.
Table 5. Isotherm constants for Pb removal by RBIDF
The r values from Langmuir constants for Pb sorption (5–20 mmol/L) to RBIF calculated at 25°C have been shown in . Since all r values are between 0 and 1, it suggests that the Langmuir model is favorable for all studied concentrations of Pb to RBIF. The results obtained from this modeling of sorption of Pb to RBIF will provide an insight into the food industry for scaling up the process to avoid dietic toxicity induced by metal pollutants. Furthermore, the results can also provide an insight into adjust the sorption isotherm modeling of fiber material for heavy metal. If the material present a monotonous or homogeneous active group, the sorption isotherm modeling for heavy metals generally fit the Langmuir model.
Table 6. Calculated r values for Pb2+ sorption by RBIFs
Thermodynamic analysis
Uptake of Pb2+ on RBIF (contact time: 360 min; 10 mM Pb) at variable temperature range (25–37°C) revealed the increase in Pb sorption as temperature increased which suggests the endothermic nature of adsorption (). The binding capacity (qmax) for RBIF-1.25 increased more (0.14–0.18 mmol/g) compared to the other two (RBIF-0.2 and 2.0) as shown in . These results were in line with Wang, Qin, and Li who reported the same endothermic adsorption phenomenon of rice bran with zinc.[Citation40]
Figure 4. Binding capacities of various RBIFs that produced with 0.2%, 1.25%, and 2.0% H2SO4 combined with 1.25% KOH for Pb2+ at different temperatures
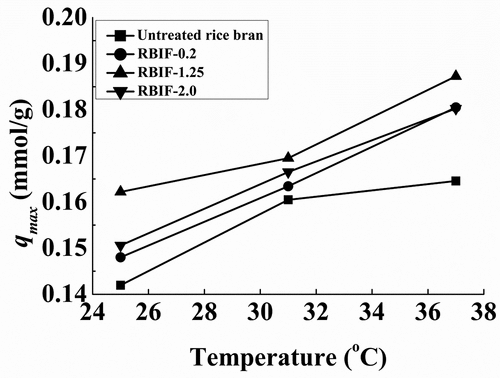
The thermodynamic parameter profiling of Pb sorption onto RBIF has been presented in . The positive values of ΔH◦ for all modified RBIF confirm endothermic nature of adsorption as obtained from the above experimental results (). However, negative ΔG◦ suggests the spontaneous nature of sorption having a high preference of Pb2+ to RBIF. Further, positive ΔS◦ showed increased randomness at the solid/solution interface (Pb to RBIF) with some structural changes. The negative ΔS◦ values suggest a stable arrangement of metal ions on the sorbent surface and vice versa.[Citation43,Citation44] In our case, all modified RBIF after interaction with Pb showed structural change as all ΔS◦ values were positive.
Table 7. Thermodynamic parameters for Pb2+ by RBIF
Conclusion
Three modified RBIF prepared from rice bran were used in batch sorption experiments to investigate the sorption behavior of lead (Pb) metal ion. The adsorption of Pb ions onto RBIF was pH dependent and sharply decreased with acidic pH. However, specific surface area and hemicellulose content of RBIF resulted in an increased Pb uptake. Further, kinetic studies demonstrated sorption equilibrium (150–240 min) for all three different RBIF and a pseudo-second-order model fitted well to Pb ion uptake vs. time profiles. On the other hand, sorption was better fitted by the Langmuir isotherm model than Freundlich and D–R adsorption models.Mean while, the thermodynamic profiling confirmed the nature of the adsorption process is endothermic and spontaneous. The enthalpy values revealed the physical adsorption was the main contributing factor. The modeling of Pb sorption experimental data will be advantageous to evaluate the mechanism of adsorption of other heavy metals and will save the experimental costs as various research groups working on heavy metal toxicity. Our aim is to use the abovementioned successful models to evaluate other heavy metal sorption mechanisms in the future work.
Acknowledgments
The authors are grateful to Dayang Rice Company of China (Jiangsu, China) for providing rice bran used in this study.
Additional information
Funding
References
- Zhang, N.; Huang, C.; Ou, S. In Vitro Binding Capacities of Three Dietary Fibers and Their Mixture for Four Toxic Elements, Cholesterol, and Bile Acid. J. Hazard. Mater. 2011, 186, 236–239. DOI: 10.1016/j.jhazmat.2010.10.120.
- Kanu, S. A.; Moyo, M.; Zvinowanda, C. M.; Okonkwo, J. O. Biosorption of Pb(II) from Aqueous Solution Using Rooibos Shoot Powder (RSP). Desalin. Water Treat. 2016, 57(12), 5614–5622. DOI: 10.1080/19443994.2015.1004116.
- Omorogie, M. O.; Babalola, J. O.; Unuabonah, E. I.; Song, W.; Gong, J. R. Efficient Chromium Abstraction from Aqueous Solution Using a Low-cost Biosorbent: Nauclea diderrichii Seed Biomass Waste. J. Saudi Chem. Soc. 2016, 20(1), 49–57. DOI: 10.1016/j.jscs.2012.09.017.
- Babalola, J. O.; Bamidele, T. M.; Adeniji, E. A.; Odozi, N. W.; Olatunde, A. M.; Omorogie, M. O. Adsorptive Modelling of Toxic Cations and Ionic Dyes onto Cellulosic Extract. Model. Earth Syst. Environment. 2016, 2, 4. DOI: 10.1007/s40808-016-0246-z.
- Babalola, J. O.; Olayiwola, F. T.; Olowoyo, J. O.; Alabi, A. H.; Unuabonah, E. I.; Ofomaja, A. E.; Omorogie, M. O. Adsorption and Desorption Kinetics of Toxic Organic and Inorganic Ions Using an Indigenous Biomass: Terminalia ivorensis Seed Waste. Int. J. Ind. Chem. 2017, 8(2), 207–220. DOI: 10.1007/s40090-016-0109-5.
- Hu, G.; Huang, S.; Chen, H.; Wang, F. Binding of Four Heavy Metals to Hemicelluloses from Rice Bran. Food Res. Int. 2010, 43, 203–206. DOI: 10.1016/j.foodres.2009.09.029.
- Ou, S. Y.; Gao, K. R.; Li, Y. An In Vitro Study of Wheat Bran Binding Capacity for Hg, Cd, and Pb. J. Agric. Food Chem. 1999, 47, 4714–4717. DOI: 10.1021/jf9811267.
- Wang, T. L.; Kao, T. H.; Inbaraj, S. B.; Su, Y. T.; Chen, B. H. Inhibition Effect of Poly(γ- Glutamic Acid) on Lead-induced Toxicity in Mice. J. Agric. Food Chem. 2010, 58, 12562–12567. DOI: 10.1021/jf1034509.
- Manini, P.; Panzella, L.; Eidenberger, T.; Giarra, A.; Cerruti, P.; Trifuoggi, M.; Napolitano, A. Efficient Binding of Heavy Metals by Black Sesame Pigment: Toward Innovative Dietary Strategies to Prevent Bioaccumulation. J. Agric. Food Chem. 2016, 64(4), 890–897. DOI: 10.1021/acs.jafc.5b05191.
- Torre, M.; Rodriguez, A. R.; Saura-Calixto, F. Interaction of Fe(II), Ca(II) and Fe(III) with High Dietary Fiber Materials: A Physicochemical Approach. Food Chem. 1995, 54, 23–31. DOI: 10.1016/0308-8146(95)92658-7.
- Idouraine, A.; Khan, M. J.; Weber, C. W. In Vitro Binding Capacity of Wheat Bran, Rice Bran and Oat Fiber for Ca, Mg, Cu, and Zn Alone and in Different Combinations. J. Agric. Food Chem. 1996, 44, 2067–2072. DOI: 10.1021/jf960151e.
- Qi, J.; Yokoyama, W.; Masamba, K. G.; Majeed, H.; Zhong, F.; Li, Y. Structural and Physico-chemical Properties of Insoluble Rice Bran Fiber: Effect of Acid-base Induced Modifications. RSC Adv. 2015, 5, 79915–79923. DOI: 10.1039/C4RA14244F.
- Montanher, S. F.; Oliveira, E. A.; Rollemberg, M. C. Removal of Metal Ions from Aqueous Solutions by Sorption onto Rice Bran. J. Hazard. Mater. 2005, B117, 207–211. DOI: 10.1016/j.jhazmat.2004.09.015.
- Oliveira, E. A.; Montanher, S. F.; Andrade, A. D.; Nóbrega, J. A.; Rollemberg, M. C. Equilibrium Studies for the Sorption of Chromium and Nickel from Aqueous Solutions Using Raw Rice Bran. Process Biochem. 2005, 40, 3485–3490. DOI: 10.1016/j.procbio.2005.02.026.
- Sun, X. F.; Sun, R. C.; Tomkinson, J.; Baird, M. S. Preparation of Sugarcane Bagasse Hemicellulosic Succinates Using NBS as a Catalyst. Carbohydr. Polym. 2003, 53, 483–495. DOI: 10.1016/S0144-8617(03)00150-4.
- Egüés, I.; Sanchez, C.; Mondragon, I.; Labidi, J. Effect of Alkaline and Autohydrolysis Processes on the Purity of Obtained Hemicelluloses from Corn Stalks. Bioresour. Technol. 2012, 103, 239–248. DOI: 10.1016/j.biortech.2011.09.139.
- Ren, H.; Jiang, J.; Wu, D.; Gao, Z.; Sun, Y.; Luo, C. Selective Adsorption of Pb(II) and Cr(VI) by Surfactant-Modified and Unmodified Natural Zeolites: A Comparative Study on Kinetics, Equilibrium, and Mechanism. Water, Air, Soil Pollut. 2016, 227, 101. DOI: 10.1007/s11270-016-2790-6.
- Özcan, A.; Sahin, M.; Özcan, A. S. Adsorption of Nitrate Ions onto Sepiolite and Surfactant-omdified Sepiolite. Adsorpt. Sci. Technol. 2005, 23, 323–333. DOI: 10.1260/0263617054769987.
- Benguella, B.; Benaissa, H. Cadmium Removal from Aqueous Solution by Chitin: Kinetic and Equilibrium Studies. Water Res. 2002, 36, 2463–2474. DOI: 10.1016/S0043-1354(01)00459-6.
- Ho, Y. S.; Wang, C. C. Pseudo-isotherms for the Sorption of Cadmiumion onto Tree Fern. Process Biochem. 2004, 39, 761–765. DOI: 10.1016/S0032-9592(03)00184-5.
- Khezami, L.; Capart, R. Removal of Chromium (VI) from Aqueous Solution by Activated Carbons: Kinetic and Equilibrium Studies. J. Hazard. Mater. 2005, 123, 223–231. DOI: 10.1016/j.jhazmat.2005.04.012.
- Freundlich, H.;. Über die Adsorption in Lösungen. Zeitschrift für Physikalische Chemie. 1907, 57U(1), 385–470.
- Rezgui, A.; Guibal, E.; Boubaker, T. Sorption of Hg (II) and Zn (II) Ions Using Lignocellulosic Sorbent (Date Pits). Can. J. Chem. Eng. 2017. DOI: 10.1002/cjce.22728.
- Hobson, J. P.;. Physical Adsorption Isotherms Extending from Ultrahigh Vacuum to Vapor Pressure. J. Phys. Chem. 1969, 73(8), 2720–2727. DOI: 10.1021/j100842a045.
- Mahramanlioglu, M.; Kizilcikli, I.; Bicer, I. O. Adsorption of Fluoride from Aqueous Solution by Acid Treated Spent Bleaching Earth. J. Fluorine Chem. 2002, 115, 41–47. DOI: 10.1016/S0022-1139(02)00003-9.
- El-Kamash, A. M.; Zaki, A. A.; Abed El Geleel, M. Modeling Batch Kinetics and Thermodynamics of Zinc and Cadmium Ions Removal from Waste Solutions Using Synthetic Zeolite-A. J. Hazard. Mater. 2005, 127, 211–220. DOI: 10.1016/j.jhazmat.2005.07.021.
- Arslanoglu, H.; Altundogan, H. S.; Tumen, F. Heavy Metals Binding Properties of Esterified Lemon. J. Hazard. Mater. 2009, 164, 1406–1413. DOI: 10.1016/j.jhazmat.2008.09.054.
- Reddad, Z.; Gérente, C.; Andrès, Y.; Ralet, M. C.; Thibault, J. F.; Cloirec, P. L. Ni(II) and Cu(II) Binding Properties of Native and Modified Sugar Beet Pulp. Carbohydr. Polym. 2002, 49, 23–31. DOI: 10.1016/S0144-8617(01)00301-0.
- Betancur-Ancona, D.; Peraza-Mercado, G.; Moguel-Ordoñez, Y.; Fuertes-Blanco, S. Physicochemical Characterization of Lima Bean (Phaseolus lunatus) and Jack Bean (Canavalia ensiformis) Fibrous Residues. Food Chem. 2004, 84, 287–295. DOI: 10.1016/S0308-8146(03)00213-9.
- Lodeiro, P.; Cordero, B.; Barriada, J. L.; Herrero, R.; Sastre de Vicente, M. E. Biosorption of Cadmium by Biomass of Brown Marine Microalgae. Bioresour. Technol. 2005, 96, 1796–1803. DOI: 10.1016/j.biortech.2005.01.002.
- Oliveira, W. E.; Franca, A. S.; Oliveira, L. S.; Rocha, S. D. Untreated Coffee Husks as Biosorbents for the Removal of Heavy Metals from Aqueous Solutions. J. Hazard. Mater. 2008, 152, 1073–1081. DOI: 10.1016/j.jhazmat.2007.07.085.
- Low, K. S.; Lee, C. K.; Leo, A. C. Removal of Metals from Electroplating Wastes Using Banana Pith. Bioresour. Technol. 1995, 51, 227–231. DOI: 10.1016/0960-8524(94)00123-I.
- Claye, S. S.; Idouraine, A.; Weber, C. W. In Vitro Binding of Five Fiber Sources and Their Insoluble Components for Magnesium and Calcium. Food Chem. 1998, 61, 333–338. DOI: 10.1016/S0308-8146(97)00059-9.
- Šćiban, M. B.; Klašnja, M. T.; Antov, M. G. Study of the Biosorption of Different Heavy Metal Ions onto Kraft Lignin. Ecol. Eng. 2011, 37, 2092–2095. DOI: 10.1016/j.ecoleng.2011.08.006.
- Gupta, N.; Kushwaha, A. K.; Chattopadhyaya, M. C. Application of Potato (Solanum tuberosum) Plant Wastes for the Removal of Methylene Blue and Malachite Green Dye from Aqueous Solution. Arabian J. Chem. 2016, 9, S707–S716. DOI: 10.1016/j.arabjc.2011.07.021.
- Wan, M. W.; Kan, C. C.; Rogel, B. D.; Dalida, M. L. P. Adsorption of Copper (II) and Lead (II) Ions from Aqueous Solution on Chitosan-coated Sand. Carbohydr. Polym. 2010, 80, 891–899. DOI: 10.1016/j.carbpol.2009.12.048.
- Weber, W. J.; Morris, J. C. Kinetics of Adsorption on Carbon from Solution. J. Sanitary Eng. Div. (Proc. ASCE) 1963, 89, 31–59.
- Wan, S.; Ma, Z.; Xue, Y.; Ma, M.; Xu, S.; Qian, L.; Zhang, Q. Sorption of Lead(II), Cadmium(II), and Copper(II) Ions from Aqueous Solutions Using Tea Waste. Ind. Eng. Chem. Res. 2014, 53(9), 3629–3635. DOI: 10.1021/ie402510s.
- Zhang, D.; Wang, C.; Bao, Q.; Zheng, J.; Deng, D.; Duan, Y.; Shen, L. The Physicochemical Characterization, Equilibrium, and Kinetics of Heavy Metal Ions Adsorption from Aqueous Solution by Arrowhead Plant (Sagittaria trifolia L.) Stalk. J. Food Biochem. 2018, 42, 1. DOI: 10.1111/jfbc.2018.42.issue-1.
- Wang, X.; Qin, Y.; Li, Z. F. Biosorption of Zinc from Aqueous Solutions by Rice Bran: Kinetics and Equilibrium Studies. Sep. Sci. Technol. 2006, 41, 747–756. DOI: 10.1080/01496390500527951.
- Guo, L.; Liang, L.; Wang, Y.; Liu, M. Biosorption of Pb2+ from Aqueous Solution by Rice Straw Modified with Citric Acid. Environ. Prog. 2016, 35(2), 359–367.
- Deng, S.; Wang, P.; Zhang, G.; Dou, Y. Polyacrylonitrile-based Fiber Modified with Thiosemicarbazide by Microwave Irradiation and Its Adsorption Behavior for Cd(II) and Pb(II). J. Hazard. Mater. 2016, 37, 64–72. DOI: 10.1016/j.jhazmat.2016.01.002.
- Xu, D.; Tan, X. L.; Chen, C. L.; Wang, X. K. Adsorption of Pb(II) from Aqueous Solution to MX-80 Bentonite: Effect of pH, Ionic Strength, Foreign Ions and Temperature. Appl. Clay Sci. 2008, 41, 37–46. DOI: 10.1016/j.clay.2007.09.004.
- Mohan, D.; Chander, S. Single, Binary, and Multicomponent Sorption of Iron and Manganese on Lignite. J. Colloid Interface Sci. 2006, 299, 76–87. DOI: 10.1016/j.jcis.2006.02.010.