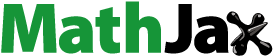
ABSTRACT
The study investigated the change in protein structure upon thermo-induced gelation of myofibrillar protein (MP, 2%) under different temperatures (30–80°C) at 0.6 M NaCl and pH 6.2. The results showed that heating from 45°C led to changes in physicochemical properties and intermolecular forces involved during MP gelation, which are related to each other. During the heating process, the free sulfhydryl content slowly increased and reached the highest value at 45°C (10.23 ± 0.13 μmol/g protein). When the temperature exceeded 50°C, Ellman’s titration and SDS-PAGE results indicated that MP gel began to form with the formation of disulfide bonds, which contributed to stabilize the gel structure. Gel strength of the heat-induced MP gel formed at 60°C was significantly higher than that at other temperatures (P < .05), reaching 1.12 ± 0.02 N. Strictly speaking, myofibrillar protein gelatinized after 60°C, and water-holding capacity of the MP gel decreased from 60-80°C, and reached a relatively low value at 80°C. Furthermore, Raman spectra showed that the α-helix content decreased with increasing temperature, β-sheets and random coil contents increased, and the content of the twist-twist-trans conformation increased steadily.
Introduction
Thermal processing is the main method of making meat products. During heating, meat products undergo a series of changes affecting protein, fat, and microorganism load, thus resulting in a specific flavor of the product.[1] Due to the high content of meat protein (about 20%) and the texture preference of low-temperature meat products, moderate heating will result in a good gel network structure. Molecular interactions upon myofibrillar protein (MP) heat-gelation are central to understand how these could affect textural properties of these meat products.
MP constitutes about 55% to 60% of the total muscle protein. It is mainly composed of myosin and actin and is an important structural protein group in meat proteins.[Citation2] In response to heat treatment, MP undergoes a gelation reaction consisting of protein unfolding (denaturation), exposure of reactive (hydrophobic) groups and sulfur-containing amino acids, protein association (aggregation) via hydrophobic interactions and new disulfide bonds forming. Then, the formation of a space-filling network (gelation) retains water in pores by capillary forces. Ultimately, this gathers into a three-dimensional network structure and finally forms a stable gel network.[Citation3] Ferry[Citation4] studied the formation of thermo-induced protein gels and reported that during the initial heating, protein molecules were denatured by heating. With increasing temperature, unfolded proteins interacted with each other to form aggregates with high molecular weight. Afterward, these aggregates are continuously depolymerized and extended to expose the reactive groups on molecular sites of proteins, thus continuously enhancing the interaction between protein molecules and re-aggregating the unfolded proteins. Finally, protein macromolecules aggregate and form a three-dimensional network gel.
During the formation of aggregates, interactions within and between protein molecules occur continuously.[Citation5] Associative forces include hydrophobic interactions, hydrogen bonding, electrostatic interactions, and disulfide bonds.[Citation6] Upon heat denaturation and aggregation, new intra- or intermolecular disulfide bonds between unfolded polypeptide chains could be established either by thiol oxidation (R1-S-+R2-S- – > R1-S-S-R2) or sulfhydryl-disulfide bond exchange reactions or (R1-S- + R2-S-S-R3 – > R1-S-S-R2 + R3-S- or R1-S-S-R3 + R2-S-).[Citation7] Accompanied by the formation of disulfide bonds on the side chains of amino acids, cysteine residues on protein polypeptides are converted to cysteine residues.[Citation8] Although disulfide bonds are covalent bonds, they are easily reduced to a thiol group, which can be oxidized again to form a disulfide bond. This shows that thiol and disulfide bonds can be transformed into each other under certain conditions. In other words, the disulfide bond content changes dynamically under certain conditions and only has relative stability.
Studies showed that disulfide bonds play an important role in stabilizing the protein molecular structure. Wedemeyer et al.[Citation9] reported that a decrease in disulfide bond content loosened the protein structure. When a disulfide bond re-forms by a thiol group under certain conditions, part of the biological activity of the protein may recover. Other studies showed that oxidation and reduction of disulfide bonds changed the protein structure and thus modified biological activity or other functions.[Citation10] At the same time, they also affect the distribution characteristics of the fat and moisture inside the protein gel, and thus affect the meat quality.[Citation7] However, the physicochemical effects of disulfide bonds on the protein structure and function have yet to be further studied.
The objective of the present work was to investigate changes in MP structure related to their heat-set gelation properties with gradient heating. Upon heating, the role of sulfhydryl change was also elucidated. The gel strength and water-holding capacity were measured to study physical properties of the gel with a texture analyzer and high-speed centrifugation, respectively. Changes in polypeptide composition of the supernatant were determined by SDS-PAGE. The changes of protein secondary structure during heating were monitored by Raman spectroscopy. The study provides new information toward understanding the mechanism of gradient heating on the effects of MP gel properties.
Materials and methods
Material pretreatment
Fresh pork center loin muscles were purchased from a local market (24 to 48 h postmortem, pH 5.6 to 5.9). Visible external fat and connective tissues were removed. The lean tissue was diced into cubes of approximately 1 cm3. The portions were placed in plastic vacuum-packaged bags, evacuated, and stored at −75°C until use.
Preparation of myofibrillar protein
Myofibrillar protein was extracted from pork muscle samples thawed at 2–4°C using an isolation buffer consisting of 0.1 M NaCl, 10 mM sodium phosphate, 2 mM MgCl2, and 1 mM EGTA at pH 7.0 as previously described.[Citation11] The slurry was centrifuged at 2000 × g for 15 min and the supernatant was discarded. The above operation was repeated twice with the same phosphate buffer. Thereafter, the pellet was washed twice using the same homogenization and centrifugation condition as above except that 0.1 M NaCl was used as washing solution. The purified MP pellet was stored at 4°C and utilized within 24–48 h of isolation.[Citation12] Protein concentration was determined by the Biuret method using bovine serum albumin (0–10 mg/ml) as standard.
Preparation of myofibrillar protein heat-induced gel
MP sols (10 g each) were transferred to 16.5 (inside diameter) × 50 (length) mm glass vials covered with aluminum foil and stored at 4°C for 12 h.[Citation11] One sample was not heated and the other samples were heated at 30°C, 35°C, 40°C, 45°C, 50°C, 55°C, 60°C, 65°C, 70°C, 75°C, and 80°C for 30 min each. After heating, the samples were cooled and stored on crushed ice for 3 h. Prior to gel strength measurements, the gel samples were allowed to equilibrate at room temperature (25 ± 1°C) for 30 min.
Gel strength and water-holding capacity
Texture profile analysis of MP gel samples were performed using a Texture Analyzer (TMS-PRO, Food Technology Corporation, USA) fitted with a cylindrical probe (flat cylindrical probe, diameter 10 mm, P/10). The samples were subjected to a double compression cycle and compressed to 50% of their original height using a pretest speed of 2.0 mm/s, a test speed of 1.0 mm/s, and a posttest speed of 2.0 mm/s. Hardness was the test strength of the gel.[Citation13]
The gel samples were transferred to 50-mL centrifuge tubes and centrifuged at 10000 × g for 15 min at 4°C; the supernatant solution was discarded.[Citation14] The water-holding capacity was calculated via EquationEquation 1(1)
(1) :
where W0 represents the total weight of the centrifuge tube and gel after discarding the supernatant solution, W represents the weight of the centrifuge tube, and W1 represents the total weight of the centrifuge tube and the gel before centrifugation.
Total and free sulfhydryl contents
The determination of total and reactive sulfhydryl contents is basically inspired by the Ellman’s titration. The measurements of thiol and disulfide bond contents were performed as described by Yongsawatdigul et al.[Citation15] with slight modifications. The samples were centrifuged at 10000 × g for 15 min. Bovine serum albumin was used as standard protein and the Lorry method was used to determine the protein content in the supernatant solution. The protein concentration was adjusted to the same concentration. One milliliter of phosphate buffer (0.6 M NaCl, 20 mM sodium phosphate, 10 mM EDTA, pH 7.0) and 400 μL of 5,5ʹ-Dithiobis-(2-nitrobenzoic acid (DTNB, 10 mM pH 7.0) were added to 100 μL samples. After fully mixing for 30 min at 37°C, the samples were centrifuged at 20000 × g for 5 min. Deionized water was used for blank samples and the absorbance was measured at 412 nm.
The measurement methods of free sulfhydryl group content were generally consistent with the above except that 8 M urea were added in 1 ml buffer. The free sulfhydryl group content is calculated via EquationEquation 2(2)
(2) :
where C0 represents the concentration of – SH (μmol/g protein), A represents the absorbance at 412 nm, e represents the absorbance coefficient (13600 M−1·cm−1),[Citation16] and D represents the dilution times before the water bath.
SDS-PAGE
The samples were centrifuged at 15000 × g for 15 min. The protein concentration of the supernatant solution was adjusted to the same concentration (6 mg/ml). The stacking (pH 8.8) and resolving gels (pH 6.8) contained 5% and 12% acrylamide, respectively, and the thickness of the gel was 1.5 mm. The protein load (weight) deposited on each well was 10 μL,[Citation17] and β-mercaptoethanol was used as reducing agent. The staining solution (0.025% Coomassie Brilliant Blue, 10% glacial acetic acid) was poured into a Petri dish, stained for about 1 h, and then decolorized with decoloring solution (10% methanol, 10% glacial acetic acid) until the protein band was clear. Standard proteins with a molecular mass ranging from 14.4 to 116 kDa were used for calibration.[Citation11]
Raman spectroscopic analysis
Raman spectroscopic analysis was performed as described by Liu et al.[Citation18] with slight modifications. Raman spectra of samples were measured at 0–25°C using a Renishaw Via micro-Raman spectrometer (British Renishaw Company) equipped with an argon laser (514.5 nm) under the following conditions: laser power, 180 mW; spectral resolution, 2.0 cm−1; the spectral signal was dispersed by 600 g·mm−1; data acquisition speed was 120 cm−1 min−1; aperture, 200 μm; scan, 3 times; integration time, 60 s; frequency correction with single-crystal silicon. The Raman spectra curve obtained at 400–3600 cm−1 was smoothly processed using the Labspec function module. Baseline correction and normalization were performed with phenylalanine (1003 cm−1) to facilitate subsequent analysis.
Statistical analysis
Each experiment was repeated at least three times, and data were statistically analyzed with one-way ANOVA and post-hoc Test (Duncan’s test; P< .05) using the program SPSS 22.0.
Results and discussion
Textural of MP gel with increased temperature
The effect of heating on the gel strength (hardness) of MP gel with increasing temperature is shown in . The results show that when the heating temperature remained below 40°C, the increase in hardness (gel strength) was small and there was no significant difference. This was due to the lower degree of coagulation of MP denaturation at this low temperature (<40°C) and the looser structure[Citation2]; therefore, the hardness at this time was smaller than that of high temperatures. Palka et al.[Citation19] reported that myosin molecules gradually aggregate at 40–60°C. When the temperature reached 60°C, the hardness increased significantly (P < .05), reaching 1.12 ± 0.02 N. At the same time, protein molecules have the highest degree of polymerization and form a more stable three-dimensional network structure.
Figure 1. Hardness of myofibrillar protein gel heated at different temperatures
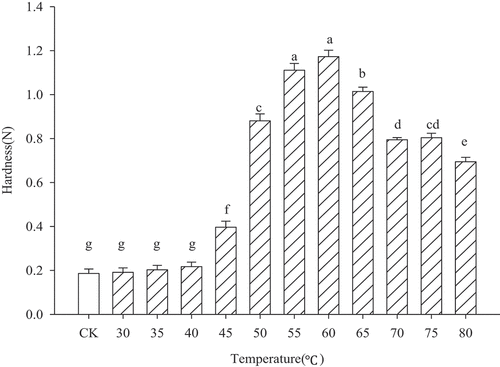
When the temperature exceeded 60°C, the gel strength decreased significantly (P < .05); furthermore, the three-dimension gel network became unstable due to the high temperature. However, when the temperature reached 75°C, MP gel strength increased slightly (P > .05) and the same results were obtained in multiple repetitions. Ko et al.[Citation20] suggested that this may be due to the formation of larger molecules with higher molecular weight than the myosin heavy chain, resulting in the exposure and conversion of part of the free sulfhydryl groups that are embedded in the MP gel network into disulfide bonds. The increased involvement of new disulfide bonds related to the level of protein unfolding/aggregation at increasing heating temperatures enhanced the obtained gel strength (more covalent cross-links than at lower temperature).
Water-holding capacity of MP gel with increased temperature
The water-holding capacity is also one of the important characteristics of protein heat-induced gels, which also affects the quality of meat products to a great extent.[Citation21] shows that when the heating temperature remained below 45°C, the sample water-holding capacity decreased slowly and the difference was not significant (P > .05). The reason for this was that the heating temperature was relatively low. MP did not denature, and centrifugation did not precipitate the water in the cold gel (or sol). Therefore, the water-holding capacity at this temperature does not reflect the water-holding properties of the MP gel. With increasing temperature (50–80°C), the MP started to gelation and formed a three-dimensional network structure. However, at 70–80°C, the water-holding capacity of the gel reached a relatively low value. During temperature increase, protein aggregates may be more tightly associated (coarse aggregation in a random fashion) than at lower temperature, since the number of cross-links increased. The formation of disulfide bonds could prevent optimal rearrangements via non-covalent forces (hydrophobic), since polypeptide chains are less flexible. More water within the gel is retained by capillary forces in small pores. Increasing gel inhomogeneities/pore size results in a decreased water-holding capacity.
Content of sulfhydryl changes in MP gels
According to traditional studies, heating can change the content of disulfide bonds. Sulfhydryl and disulfide bonds can be converted into each other. The effects of heating with increasing temperatures on the total sulfhydryl and free sulfhydryl contents in myofibrillar protein samples are shown in . Sharp et al.[Citation22] suggested that heating can expose hidden thiol groups, disulfide bonds, and hydrophobic groups inside protein molecules, thus enhancing the interaction between molecules and providing a driving force for gel formation. While increasing heating temperature, the total sulfhydryl content decreased. However, the change in free sulfhydryl content was inconsistent with the total sulfhydryl groups. When the heating temperature increased to 45°C, the free thiol content slowly increased and reached the highest measured value at 45°C (10.23 ± 0.13 μmol/g protein). Yongsawatdigul et al.[Citation15] studied the effect of heat treatment on the free sulfhydryl content of squid actin and also reached a similar conclusion. They pointed out that the sulfhydryl content increased with increasing temperature at the low-temperature stage. It has been speculated that the increase of free sulfhydryl content may be because heating causes a heavy chain unfolding of the protein molecule, so that the originally existing disulfide bond is cleaved or the thiol group embedded in the molecule is released.[Citation12] At low temperatures, the incomplete “sol-to-gel” state of MP can be considered as a protein solution dispersion, in which most of the protein molecules form disulfide bonds and stabilize the dispersed phase structure.[Citation15] Therefore, the disulfide bond content is higher at this time. When heated, disulfide bonds are cleaved into free sulfhydryl groups, resulting in a continuous reduction of disulfide bonds.
Figure 3. Reactive and total sulfhydryl (SH) groups of myofibrillar protein gel heated at different temperatures
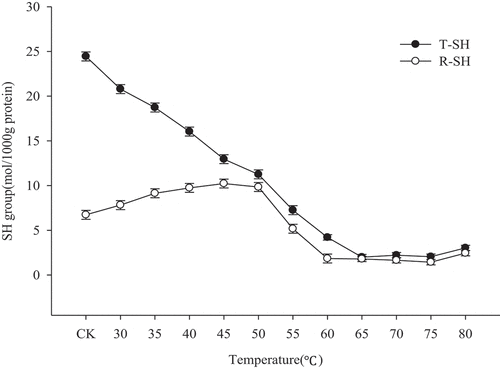
As the temperature continued to increase, the total sulfhydryl content and free sulfhydryl content decreased slowly, indicating that disulfide bonds were continuously formed, which is consistent with the results of Liu et al.[Citation18] When the temperature exceeded 50°C, the increase of disulfide bond content can promote cross-linking in myosin gels.[Citation23] When the temperature continued to increase (65–70°C), the total sulfhydryl content and free sulfhydryl content also increased; however, it slightly decreased at 75°C and the disulfide bond content increased. This may be due to the conversion of sulfhydryl groups to disulfide bonds, which led to a slight increase in gel strength and a small recovery in water retention.[Citation20] Presumably, the gel structure is most stable at this point, which is consistent with the change in gel strength.
SDS-PAGE analysis of protein from supernatants of MP gel heated at different temperatures
The internal protein structure changes of the protein gel were characterized by SDS-PAGE. shows the electrophoretic analysis of supernatants after centrifugation of myofibrillar protein gels heated at different temperatures. Obviously, the band at 30°C is the same as the unheated band at room temperature. The changes in actin bands in are in agreement with the results of Sharp et al.[Citation22], which were found using transmission electron microscopy. They pointed out that at 30–40°C and pH 6.5; the myosin structure did not change at the beginning, followed by the aggregation of a number of molecules in the head to form dimer. Subsequently, the dimers were further gathered together. At this time, as can be seen from , the myosin heavy chain gradually blurred from clarity, indicating that the head or tail of the myosin heavy chain unfolded gradually and participated in intermolecular and intramolecular cross-linking; however, it was still not fully involved in the gel formation.[Citation17] At 40–60°C, myosin further aggregated until the protein monomer disappeared in the supernatant. However, when the temperature exceeded 50°C, the myosin heavy chain band disappeared, indicating that it had been involved in the formation of a gel and did not exist in the supernatant. In addition, changes in the α-actinin (95 kDa) band are consistent with the myosin heavy chain. Changes in the above bands also confirm the analysis and results of , , and .
Figure 4. SDS-PAGE of supernatants protein in myofibrillar protein gel heated at different temperatures
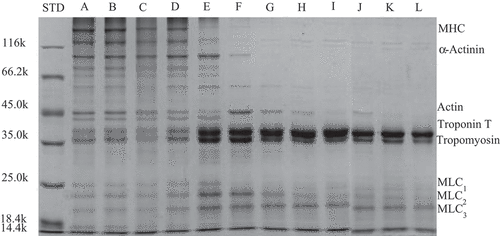
From 30°C to 45°C, the actin bands became deeper and shallower, which may explain the unfolding of the actin molecule. This indicates that actin gradually participated in the formation of the myofibrillar protein gel network and no longer exited independently (). At 50°C, the actin band suddenly deepened. Ko et al.[Citation20] analyzed the effects of heat treatment on actin in tilapia and found similar results. They suggested that the actomyosin that initially aggregates at this temperature to form a macromolecular gel may exist independently at a temperature of 50–60°C due to depolymerization of the gel. When the temperatures exceeded 55°C, actin unfolded[Citation17] and the tropomyosin and troponin T bands progressively darkened, likely due to water loss in the gel after formation. Troponin T and tropomyosin were not involved in the network of the heat-set gel and these are released in non-retained water (supernatant).[Citation20]
Raman spectroscopy of MP gel
The effect of heating at different temperature gradients on the Raman bands (400–2000 cm−1) of MP gels is shown in . The amide I band mainly includes stretching vibrations of C = O, a small portion of C-N, and N-H within the peptide group, which are usually around 1600–1700 cm−1. The specific frequency represents different protein secondary structures. For example, 1650–1660 cm−1, 1665–1690 cm−1, and 1650–1665 cm−1 represent the amide I bands of α-helices, β-sheets, and random coils, respectively.[Citation24] The amide III band mainly includes stretching vibrations of N-H within the C-N extension and small molecule peptide groups and is typically located in the vicinity of 1200–1350 cm−1. The wavenumber depends on the geometry of the protein backbone.[Citation25]
Figure 5. Raman spectra in 400–2000 cm−1 region of myofibrillar protein gels heated at different temperatures
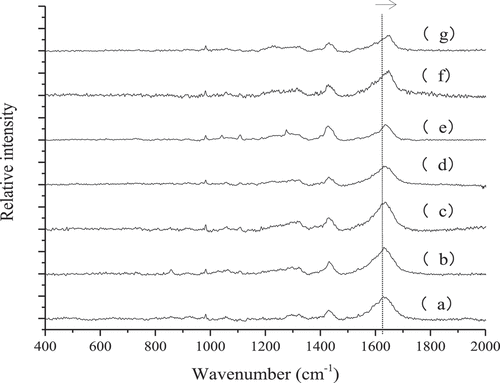
Theoretically, the main conformational information of the amide I band is identical to that of amide III bands. Considering the contradiction of the spectral peak assignment of the amide III band, only the amide I band of the heated gel at different temperatures was quantitatively analyzed. In addition, the movement of characteristic peaks of Raman spectra can be divided into “blue shift” and “red shift”. Blue shift refers to the increase of wavenumber and vibration energy, which may be due to the introduction of new groups or related group activation, leading to a more stable structure. The red shift is contrary to the blue shift, which may be due to structural changes and exposure of the related groups.[Citation26] As shown in , as the temperature increased, the Raman spectral peak of the MP gel shifted to blue, and the peak intensity gradually decreased. This indicated that heating led to the introduction of new groups or certain groups of activation in the myofibrillar protein gel, and the gel structure became more stable.
Analysis of amide I bands via protein gel Raman spectroscopy
Protein secondary structure refers to the partial peptide backbone structure in the peptide chain, mainly including α-helix, β-sheet, β-turn, and random coil. Since multiple vibration peaks of the secondary structure of the protein amide I band overlapped easily, a curve-fitting method was used to distinguish the different overlapping bands to obtain more accurate information. As shown in , unheated MP had the highest α-helix content of 93.27%. As the temperature increased, the α-helix content in the secondary structure of MP gradually decreased. Especially when the temperature was between 55°C and 65°C, the α-helix content decreased significantly. It has been suggested that this was due to the expansion of α-helices in actin and myosin.[Citation15] At the same time, as the temperature increased, the β-sheet content and random curl content increased, which was similar to the findings of Liu et al.[Citation18] Therefore, it can be speculated that β-sheeting was also critical for myofibrillar protein gelation.
S-S stretching
The formation of disulfide bonds and the inter-transformation of disulfide bonds play important roles in MP thermo-induced gelation.[Citation18] In the wavenumber range of 500–550 cm−1 the typical band corresponding to disulfide bonds could be detected (). 510 cm−1 is a characteristic band of proteins and peptides containing cysteine residues, which represents the full-twist conformation in disulfide bonds.[Citation27] The characteristic band at 516–530 cm−1 represents the twisted-twisted-trans conformation in the disulfide bond, while the band at 535–545 cm−1 represents the trans-twist-trans conformation in the disulfide bond, or the trihedral conformation with C-C-S-S.[Citation28] These conformations are formed by the S-S stretching vibration caused by internal changes of C-C and C-S.[Citation29] When not heated, the relative intensity of the band at 510 cm−1 and 525 cm−1 was relatively high, and the relative intensity of the band at 540 cm−1 was relatively low. It has been speculated that the disulfide bonds showed a full-twist conformation and a twist-twist-trans conformation. Tu[Citation25] also suggested that the conformation of the disulfide bonds in native proteins was mainly in the form of a full twisted conformation. At 30°C, the relative intensity of the band at 525 cm−1 was reduced; and there was no significant difference in the remaining bands. It has been speculated that the twisted-twisted-trans conformation in the gel may be converted to other conformations.
Figure 7. S-S stretch in 450–600 cm−1 region of myofibrillar protein gel heated at different temperatures
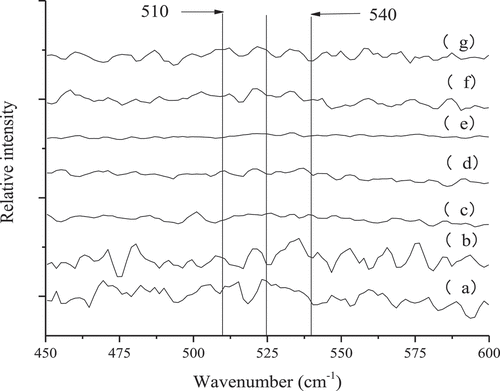
With increasing temperature (30–60°C), the relative intensity of the band at 510 cm−1 gradually decreased, and the relative intensity of bands at 525 cm−1 and 540 cm−1 gradually increased. This is presumably because the gel full-twist conformation transforms into a twist-twist-trans conformation and a trans-trans-distortive conformation. In addition, tilapias MPs also show similar phenomenon. As the temperature continues to increase (>60°C), the full-twist, twist-twist-trans, and trans-twist-trans conformations reappear in the gel, and blue shifts occur. Further analysis of shows that only the content of the twist-twist-trans conformation was steadily increasing. Therefore, this could be related to MP gel formation. Ogawa et al.[Citation30] also concluded that the gel formation of fish AMs in setting required twist-twist-trans conformation of disulfide bonds as well as a slow unfolding of the α-helix.
Changes of peaks on 760 cm−1, 850 cm−1, and 830 cm−1 in aromatic amino acid side chains
Aromatic amino acid side chains have multiple Raman spectra characteristic absorption peaks, which can effectively characterize the polarity of the microenvironment around hydrogen bonds.[Citation29] The characteristic peak at 760 cm−1 is due to the vibration of the ring of tryptophan (Trp) residues.[Citation31] shows that as the temperature increased, I-760 continuously decreased because heating changed the tryptophan residue from the embedded state to the exposed state.[Citation29] Furthermore, hydrophilic (polar) groups were embedded and hydrophobic (nonpolar) groups were exposed, which facilitated the interaction between protein molecules, formed a stable three-dimensional structure, and maintained moisture well.[Citation32] Tryptophan residues are extremely sensitive to the degree of polarity of the environment and exposed residues are more sensitive than embedded residues.[Citation33] However, Brownlow et al.[Citation34] introduced the opposite view and suggested that heating caused an increase in the characteristic peak area at 760 cm−1.
Table 1. Normalized intensity of the 760 cm−1 band, the 1450 cm−1 band, and normalized ratio of I850/I830 doublet bands
The tyrosine doublet bands, located at 830 and 850 cm−1, are particularly useful to monitor the microenvironment around the tyrosine residue, and the degree to which the residue is buried. A high ratio I850/830 of 0.9–2.5 indicates that the tyrosine residue is exposed, while a low ratio indicates strong hydrogen bonding.[Citation35,Citation36] shows that the I-850/830 ratio of the blank group can reach 0.94, and when the ratio was between 0.5 and 1.25, this indicated that the tyrosine residue may be buried or exposed to a hydrophobic environment.[Citation37] However, heating to 30°C resulted in a significant decrease of I-850/830. With the gradual increase of temperature, I-850/830 also gradually increased. An I-850/830 ≥ 1.25 indicated that tyrosine residues were exposed on the surface of protein molecules where they can be used as hydrogen bond acceptors or hydrogen bond donors.[Citation38] When the temperature reached 70°C, the I-850/830 can be as high as 3.18, indicating that tyrosine residues were exposed to more polar environments and formed strong hydrogen bond receptors on the protein surface.[Citation34] Thereafter, I-850/830 decreased again and Tu[Citation38] suggested that the hydrogen bonds in the tyrosine residues may be involved in the intermolecular interaction and returned to the buried state. Wang et al.[Citation39] showed that the formation of hydrogen bonds in β-sheets is extremely important to stabilize gel networks. Changes in the state of these amino acids are conducive to the formation of heat-set gels.
The band at 1450 cm−1 is related to the bending vibrations of CH3, CH2, and CH.[Citation40] shows that as the temperature increased, I-1450 gradually decreased, and compared to the blank group, the gel I-1450 after heating decreased significantly (P < .05). This may be due to the increase of hydrophobic interactions between the aliphatic amino acid side chains in response to heating, which promotes the cross-linking of proteins.[Citation41]
Correlation analysis
As shown in , the total sulfhydryl content and the free sulfhydryl content in the gel correlated significantly with the water-holding capacity of the gel, the disulfide bond content in the gel, the β-sheet content, and I-760 (P < .01), while they significantly positively correlated with gel strength (P < .05). The results of Liu et al.[Citation42] showed a significant negative correlation between the gel water-holding capacity and β-sheet content caused by heating (P < .05), which is consistent with . Because the decrease of I-760 led to an increase of hydrophobic interactions between protein molecules, the hydrophobic groups within the molecule were exposed and the hydrophilic groups were embedded; then, the hydrophilicity of the protein molecules decreased, which led to the decrease of the gel water retention. Therefore, I-760 was significantly positively correlated with water-holding capacity (P < .05). In addition, the reduction of I-760 also led to an increase in the intermolecular interaction of proteins, which facilitated the aggregation of protein molecules and the formation of gels, thus leading to an increase in the content of β-sheets. Therefore, a significant negative correlation was found between I-760 and β-sheet content (P < .05). During heat-induced gel formation by MPs, heating changes the intermolecular forces such as sulfhydryl groups and disulfide bonds, hydrophobic interactions, and hydrogen bonds. This affects the molecular structure and status of the protein, ultimately affecting the properties of the gel.
Table 2. Pearson correlation of gel properties and secondary structure of myofibrillar protein gels heated at different temperatures
Conclusion
MP heat-induced gels were formed by gradient heating and changes in protein structure and their sol-to-gel properties during gel formation were investigated. Different temperature heat treatment could result in different textural properties during sol-to-gel process. MP gel formed at 60°C and hardness of the gel was significantly higher than that at other temperatures. Strictly speaking, water-holding capacity of MP gel could be shown only when temperature increased to 50°C. While increasing heating temperature of MP at 2% protein concentration, pH 6 at 0.6 M NaCl, the total sulfhydryl content and free sulfhydryl content decreased slowly, indicating that disulfide bonds were continuously formed. The gel strength increased and then decreased, and the water-holding capacity decreased gradually. A series of physical properties changed during gel formation, including texture, water retention, and secondary structure. It was significantly correlated with changes in intermolecular forces. Among these, a decrease of α-helix content, an increase of β-sheet content, and the formation of the twist-twist-trans conformation in disulfide bond conformation were closely related to the formation of the gel. MP formed a gel above 50°C, while myosin and actin molecules were not present in the gel and the increased disulfide bond content can promote the cross-linking of the gel. This study also shows that the gel structure formed at 60°C was the most stable and the content of disulfide bonds in the gel was relatively high.
Acknowledgments
This study was supported by the National Natural Science Foundation of China (31371792), the Jiangsu Agriculture Science and Technology Innovation Fund (JASTIF) (CX(16)1007), the Sci-Technology program of Jiangsu Province (BN2016191), and the Cultivating Targets of Young and Middle-aged Academic Leaders in Yangzhou University (201606) and Jiangsu Government Scholarship for Overseas Studies.
References
- Pisula, A.; Tyburcy, A. Hot Processing of Meat. Meat Sci. 1996, 43, 125–134.
- Tornberg, E. Effects of Heat on Meat proteins–Implications on Structure and Quality of Meat Products. Meat Sci. 2005, 70, 493–508.
- Sanchez-Gonzalez, I.; Carmona, P.; Moreno, P.; Borderias, J.; Sanchez-Alonso, I.; Rodriguez-Casado, A.; Careche, M. Protein and Water Structural Changes in Fish Surimi during Gelation as Revealed by Isotopic H/D Exchange and Raman Spectroscopy. Food Chem. 2008, 106, 56–64.
- Ferry, J. D. Protein Gels. Adv. Protein Chem. 1948, 4, 1–78.
- Montero, P.; Gomez-Guillen, C. Thermal Aggregation of Sardine Muscle Proteins during Processing. J. Agric. Food Chem. 1996, 44, 3625–3630.
- Perez-Mateos, M.; Lourenco, H.; Montero, P.; Borderias, A. J. Rheological and Biochemical Characteristics of High-pressure-and Heat-induced Gels from Blue Whiting (micromesistius Poutassou) Muscle Proteins. J. Agric. Food Chem. 1997, 45, 44–49.
- Yang, H.; Zhang, W.; Li., T.; Zheng., H.; Khan., M. A.; Xu, X.; Sun, J.; Zhou, G. H. Effect of Protein Structure on Water and Fat Distribution during Meat Gelling. Food Chem. 2016, 204, 239–245.
- Fritz, J. D.; Swartz, D. R.; Greaser, M. L. Factors Affecting Polyacrylamide Gel Electrophoresis and Electroblotting of High-molecular-weight Myofibrillar Proteins. Anal. Biochem. 1989, 180, 205–210.
- Wedemeyer, W. J.; Welker, E.; Mahesh Narayan, A.; Scheraga, H. A. Disulfide Bonds and Protein Folding†. Biochemistry. 2000, 39, 4207–4216.
- Hoffmann, M. A. M.; Mil, P. J. J. M. V. Heat-Induced Aggregation of β-Lactoglobulin: Role of the Free Thiol Group and Disulfide Bonds. J. Agric. Food Chem. 1997, 45, 2942–2948.
- Wu, M.; Xiong, Y. L.; Chen, J.; Tang, X.; Zhou, G. Rheological and Microstructural Properties of Porcine Myofibrillar Protein–Lipid Emulsion Composite Gels. J. Food Sci. 2009, 74, E207–E217.
- Xiong, Y. L.; Brekke, C. J. Gelation Properties of Chicken Myofibrils Treated with Calcium and Magnesium Chlorides1. J. Muscle Foods. 2007, 2, 21–36.
- Montejano, J. G.; Hamann, D. D.; Lanier, T. C. Comparison of Two Instrumental Methods with Sensory Texture of Protein Gels. J. Texture Stud. 1985, 16, 403–424.
- Tang, C. H.; Wang, X. Y.; Yang, X. Q.; Lin, L. Formation of Soluble Aggregates from Insoluble Commercial Soy Protein Isolate by Means of Ultrasonic Treatment and Their Gelling Properties. J. Food Eng. 2009, 92, 432–437.
- Yongsawatdigul, J.; Park, J. W. Thermal Denaturation and Aggregation of Threadfin Bream Actomyosin. Food Chem. 2003, 83, 409–416.
- Ellman, G. L. Tissue Sulfhydryl Groups. Arch Biochem Biophys. 1959, 82, 70–77.
- Ramirez-Suarez, J. C.; Addo, K.; Xiong, Y. L. Gelation of Mixed Myofibrillar/wheat Gluten Proteins Treated with Microbial Transglutaminase. Food Res. Int. 2005, 38, 1143–1149.
- Liu, R.; Zhao, S. M.; Xie, B. J.; Xiong, S. B. Contribution of Protein Conformation and Intermolecular Bonds to Fish and Pork Gelation Properties. Food Hydrocoll. 2011, 25, 898–906.
- Palka, K.; Daun, H. Changes in Texture, Cooking Losses, and Myofibrillar Structure of Bovine M. Semitendinosus during Heating. Meat Sci. 1999, 51, 237–243.
- Ko, W. C.; Yu, C. C.; Hsu, K. C. Changes in Conformation and Sulfhydryl Groups of Tilapia Actomyosin by Thermal Treatment. LWT - Food Sci. Technol. 2007, 40, 1316–1320.
- Huff-Lonergan, E.; Lonergan, S. M. Mechanisms of Water-holding Capacity of Meat: The Role of Postmortem Biochemical and Structural Changes. Meat Sci. 2005, 71, 194–204.
- Sharp, A.; Offer, G. The Mechanism of Formation of Gels from Myosin Molecules. J. Sci. Food Agr. 1992, 58, 63–73.
- Foegeding, E. A. Functional Properties of Turkey Salt-Soluble Proteins. J. Food Sci. 1987, 52, 1495–1499.
- Herrero, A. M. Raman Spectroscopy for Monitoring Protein Structure in Muscle Food Systems. Crit. Rev. Food Sci. 2008, 48(6), 512–523.
- Tu, A. T. Raman Spectroscopy in Biology: Principles and Applications, first ed.; Wiley: New York, 1982.
- Ramazan, K.; Joseph, I. Applications of Raman Spectroscopy for Food Quality Measurement. In Nondestructive Testing of Food Quality; Irudayaraj, I., Reh, C., Eds.; Blackwell Publ: Hoboken, 2008; pp pp. 143–163.
- Li-Chan, E.; Nakai, S.; Hirotsuka, M. Raman Spectroscopy as a Probe of Protein Structure in Food Systems. In Protein Structure-function Relationships in Foods; Yada, R. Y., Jackman, R. L., Smith, J. L., Eds.; Blackie Academic & Professional: London, 1994; pp 163–197.
- Ngarize, S.; Herman, H.; Adams, A.; Howell, N. Comparison of Changes in the Secondary Structure of Unheated, Heated, and High-pressure-treated Beta-lactoglobulin and Ovalbumin Proteins Using Fourier Transform Raman Spectroscopy and Self-deconvolution. J. Agric. Food Chem. 2004, 52, 6470–6477.
- Li-Chan, E. C. Y. The Applications of Raman Spectroscopy in Food Science. Trends Food Sci. Technol. 1996, 7, 361–370.
- Ogawa, M.; Nakamura, S.; Horimoto, Y.; An, H.; Tsuchiya, T.; Nakai, S. Raman Spectroscopic Study of Changes in Fish Actomyosin during Setting. J. Agric. Food Chem. 1999, 47, 3309–3318.
- Byler, D. M.; Susi, H.; Farrell, H. M., Jr. Laser-Raman Spectra, Sulfhydryl Groups, and Conformation of the Cystine Linkages of Beta-lactoglobulin. Biopolymers. 1983, 22, 2507–2511.
- Brondum, J.; Munck, L.; Henckel, P.; Karlsson, A.; Tornberg, E.; Engelsen, S. B. Prediction of Water-holding Capacity and Composition of Porcine Meat by Comparative Spectroscopy. Meat Sci. 2000, 55, 177–185.
- Bellocq, A. M.; Lord, R. C.; Mendelsohn, R. Laser-excited Raman Spectroscopy of Biomolecules. 3. Native Bovine Serum Albumin and Beta-lactoglobulin. Biochim. Biophys. Acta. 1972, 257, 280–287.
- Bouraoui, M.; Nakai, S.; Li-Chan, E. In Situ Investigation of Protein Structure in Pacific Whiting Surimi and Gels Using Raman Spectroscopy. Food Res. Int. 1997, 30, 65–72.
- Linlaud, N.; Ferrer, E.; Puppo, M. C.; Ferrero, C. Hydrocolloid Interaction with Water, Protein, and Starch in Wheat Dough. J. Agr. Food Chem. 2011, 59, 713–719.
- Badii, F.; Howell, N. K. Fish Gelatin: Structure, Gelling Properties and Interaction with Egg Albumen Proteins. Food Hydrocolloid. 2006, 20, 630–640.
- Zhang, Z.; Yang, Y.; Tang, X.; Chen, Y.; You, Y. Chemical Forces and Water Holding Capacity Study of Heat-induced Myofibrillar Protein Gel as Affected by High Pressure. Food Chem. 2015, 188, 111–118.
- TU, T. A. Peptide Backbone Conformation and Microenvironment of Protein Side Chains. In Advances in Infrared & Raman Spectroscopy; Clark, R. J. H., Hester, R. E., Eds.; Wiley: Chichester, 1986; pp 47–112.
- Cheng, H. W.; Damodaran, S. Thermal Gelation of Globular Proteins: Influence of Protein Conformation on Gel Strength. J. Agric. Food Chem. 1991, 39, 999–1006.
- Ikeda, S.; Li-Chan, E. C. Y. Raman Spectroscopy of Heat-induced Fine-stranded and Particulate β-lactoglobulin Gels. Food Hydrocoll. 2004, 18, 489–498.
- Herrero, A. M.; Cambero, M. I.; Ordóez, J. A.; Hoz, L. D. L.; Carmona, P. Raman Spectroscopy Study of the Structural Effect of Microbial Transglutaminase on Meat Systems and Its Relationship with Textural Characteristics. Food Chem. 2008, 109, 25–32.
- Liu, R.; Zhao, S. M.; Xiong, S. B.; Xie, B. J.; Qin, L. H. Role of Secondary Structures in the Gelation of Porcine Myosin at Different pH Values. Meat Sci. 2008, 80, 632–639.