ABSTRACT
The objectives of this study were to investigate the structural characterization, antioxidant activity, and α-amylase inhibitory effect and mechanism of ellagitannin-rich fractions (ETRF) from the mangrove leaves of three Sonneratia species, namely S. apetala, S. caseolaris, and S. alba. It was found that the ETRF from these Sonneratia species characterized by MALDI-TOF-MS and reversed-phase HPLC consisted a complex mixture of oligomeric ellagitannins. All samples possessed similar mass distributions, but different polymer chain length, with the longest for S. caseolaris up to pentamers and the shortest for S. alba up to trimers. Acid hydrolysis of the ETRF resulted in substantial amounts of gallic acid and ellagic acid, which the concentration of ellagic acid was significantly higher than that of gallic acid. The ETRF displayed excellent inhibition against α-amylase activity with IC50 values of 10.12 ± 0.08, 7.96 ± 0.05, and 11.96 ± 0.07 µg/mL for S. apetala, S. caseolaris, and S. alba, respectively. Analysis of fluorescence data revealed that the quenching mechanism of α-amylase fluorescence by ETRE was the static quenching and the complexes between them might be formed. It was also observed that the ETRF possessed stronger DPPH and ABTS radical scavenging activities, as well as ferric reducing powers than that of synthetic antioxidant BHA. Results of this study are valuable not only for elucidating ellagitannin compositions of three Sonneratia species but also for further exploiting them as a potential source of natural antioxidant agents and α-amylase inhibitors.
Introduction
Tannins are a family of phenolic secondary metabolites in many plants. Epidemiological evidence has indicated that dietary consumption of tannins may contribute to lower the risk of some human diseases.[Citation1] Tannins in vascular plants occur as two types, the condensed and the hydrolyzable.[Citation2] The hydrolyzable tannins are further subdivided into gallotannins and ellagitannins. Gallotannins consist of a sugar core acylated partially or wholly with gallic acid. Ellagitannins are biogenetically derived from gallotannins by C-C oxidative coupling between neighboring galloyl groups on the glucose core to form hexahydroxydiphenoyl units. It has been reported that the hydrolyzable tannins are less widely distributed in the plant kingdom than the condensed tannins and many other phenolic compounds.[Citation3-Citation5]
Ellagitannins have received much attention recently in the fields of nutrition, health, and medicine largely due to their potential health-promoting benefits and utilizations as antioxidant, anti-inflammatory, antibacterial, and anti-HIV replication products.[Citation6] The remarkable antitumor-promoting effect of these compounds in various animal models and tumor systems suggested that these polyphenols are universal antitumor agents.[Citation7]
Mangroves are a special kind of forest occurring in tropical and subtropical coastlines. In mangrove species, 20% of their dry weights are usually condensed tannins, which mainly prevent damage from herbivory.[Citation2] Previously, the condensed tannins present in some mangrove species have been confirmed and reported to exhibit significant antioxidant activities.[Citation8-Citation11] In comparison with condensed tannins, however, the studies on the hydrolyzable tannins in mangroves have rarely been reported. Sonneratia species are the mangroves of Sonneratiaceae. The leaves of S. apetala, and the fruits of S. caseolaris and S. alba were traditionally used in the treatment of bleeding, hemorrhages, piles, sprains, and hepatitis.[Citation12]
Matrix-assisted laser desorption/ionization time-of-flight mass spectrometry (MALDI-TOF-MS) is a powerful technique in characterization of both synthetic and natural polymers.[Citation13-Citation15] It has been successfully applied to identify condensed tannins in some mangrove species, including Kandelia candel, Rhizophora mangle, R. apiculata, Aegiceras corniculatum, and Ceriops tagal.[Citation8-Citation11,Citation16,Citation17] To our knowledge, however, few works have been demonstrated on the characterization of hydrolyzable tannin in mangroves by MALDI-TOF-MS.
In this study, the ellagitannin-rich fractions (ETRF) in three Sonneratia species leaves, namely S. apetala, S. caseolaris, and S. alba were isolated by a Sephadex LH-20 column chromatography. Their structural features were for the first time elucidated by MALDI-TOF-MS and reversed-phase high-performance liquid chromatography (HPLC) analyses. In addition, the antioxidant activity and α-amylase inhibitory effect and mechanism of these ETRF were also estimated. The results obtained would not only contribute to a better understanding of the chemical compositions and potential health benefits of Sonneratia species leaves but also further increase the applicability of MALDI-TOF-MS in the analysis of hydrolyzable tannins.
Materials and methods
Sample collection
The third to fifth pairs of developmentally matured leaves of three Sonneratia species, namely S. apetala, S. caseolaris, and S. alba were collected in the Dongzhai Harbor Mangrove National Natural Reserves (Hainan, China). Leaves damaged by insects and disease or mechanical factors were avoided. After collection, the leaves were cleaned with distilled water and freeze dried using a desktop freeze-dryer at −56°C for 72 h. Then, the freeze-dried leaves were ground finely and stored at −20°C prior to extraction.
Chemicals and reagents
Porcine pancreatic α-amylase (A3176), corn starch (S4126), Sephadex LH-20, cesium chloride, 2,5-dihydroxybenzoic acid (DHB), 2,2-diphenyl-1-picrylhydrazyl (DPPH), 2,2ʹ-azinobis(3-ethylbenzothiazoline-6-sulfonic acid) (ABTS), 2,4,6-tripyridyl-S-triazine (TPTZ), butylated hydroxyanisole (BHA), ascorbic acid, gallic acid, and ellagic acid were obtained from Sigma-Aldrich (St. Louis, MO, USA). Acetonitrile (HPLC grade) was purchased from Macklin (Shanghai, China). Acarbose was obtained from J&K Scientific Ltd. (Beijing, China). All other chemicals and reagents used were of analytical grade and obtained from Sinopharm Chemical Reagent Co., Ltd. (Shanghai, China).
Preparation of the ellagitannin-rich fractions (ETRF)
The ETRF from the leaves of three Sonneratia species were prepared according to our previous procedures.[Citation18] The finely ground leaf powders (10 g of each) were extracted thrice with 70% aqueous acetone (100 mL) at room temperature. The extracts were filtered and pooled, and the acetone was eliminated by evaporation under vacuum at 38°C. The aqueous phase was recovered and partitioned with hexane in order to remove chlorophyll and lipophilic compounds. The remaining aqueous phase was applied to a Sephadex LH-20 column (30 × 2.5 cm i.d.) and eluted successively with 50% aqueous methanol and 70% aqueous acetone. The resulting acetone eluates were rotary-evaporated and lyophilized to obtain respective ETRF stored at 4°C until use.
MALDI-TOF-MS analysis
The mass spectra of ETRF were recorded on a Bruker Reflex III MALDI-TOF-MS spectrometer (Bruker, Germany) equipped with a pulsed nitrogen laser (337 nm). An accelerating voltage of 20.0 kV and a reflectron voltage of 23.0 kV were used in the positive reflectron mode. The DHB and Cs+ was selected as the matrix and the cationization reagent, respectively. The sample solution, the cesium chloride solution, and the matrix solution were mixed at a volumetric ratio of 1:1:3. The mixture was deposited onto a MALDI target and crystallized at room temperature before analysis.[Citation19]
Acid hydrolysis coupled with reversed-phase HPLC analysis
The acid hydrolysis reaction was accomplished depending on a method reported by Oszmianski et al.[Citation20] The ETRF (25 mg of each) was hydrolyzed with 2 mL of 2 mol/L HCl in a boiling water bath for 1 h. After cooling, a mix of 2 mL of 2 mol/L NaOH and 6 mL methanol were added to the vial. The slurry was sonicated for 20 min with occasional shaking, following by centrifugation at 12000g. The supernatant was collected and membrane-filtered (0.45 µm) prior to HPLC analysis.
The Agilent 1200 series liquid chromatograph equipped with a diode array detector and a quaternary pump was used. Analysis was performed on a Hypersil ODS column (Elite, China) with dimensions of 250 × 4.6 mm and a particle size of 5 µm. The elution was done using a linear gradient solvent system consisting of water-0.1% (v/v) trifluoroacetic acid (A) and acetonitrile-0.1% (v/v) trifluoroacetic acid (B) as follows: 0–2 min 100% A, 2–6 min 0–5% B, 6–10 min 5% B, 10–15 min 5–10% B, 15–20 min 10% B, 20–30 min 10–20% B, 30–35 min 20% B, and 35–40 min 20–30% B. The flow rate was set at 1 mL/min and the absorption wavelength was performed at 254 nm. The chromatographic peaks were identified by comparison of their relative retention times against those of pure standards. Ellagic acid and gallic acid were quantified by referring to the well-established calibration curve of standards.
DPPH radical scavenging activity assay
The method described by Delfanian et al.[Citation21] with minor modifications was used to evaluate the DPPH radical scavenging activity of ETRF. Sample solution of 0.1 mL was added to 3.9 mL of a 25 mg/L methanol DPPH radical solution. The resulting sample mixtures were allowed to stand at ambient temperature for 30 min and their absorbance was measured at 517 nm. The capability to scavenge the DPPH radical by the sample was calculated according to the following equation: DPPH scavenging effect (%) = [(A1–A2)/A1] × 100, where A1 is the absorbance of control and A2 is the absorbance in the presence of the sample. The IC50 value is the concentration of the sample that could scavenge 50% of the DPPH radicals. The synthetic antioxidant BHA was used as the reference compound.
ABTS radical scavenging activity assay
ABTS radical scavenging activity of ETRF was carried out according to the method described by Zhou et al.[Citation22] with minor modifications. ABTS radical cation was prepared by mixing an ABTS stock solution (7 mM) with 2.45 mM potassium persulfate at a ratio of 1:1 (v/v) and diluted to obtain an absorbance of 0.700 ± 0.050 at 734 nm. Sample solution of 0.1 mL was added to the ABTS working solution and mixed thoroughly. The reaction mixture was allowed to stand at room temperature for 6 min and the absorbance was immediately recorded at 734 nm. The inhibition of the ABTS radical by the sample was calculated by the following equation: ABTS scavenging effect (%) = [(A1–A2)/A1] ×100, where A1 is the absorbance of control and A2 is the absorbance in the presence of the sample. The IC50 value is the concentration of the sample that could scavenge 50% of the ABTS radicals. The synthetic antioxidant BHA was used as the reference compound.
Ferric reducing antioxidant power (FRAP) assay
The FRAP of ETRF was determined as previously described by Wei et al.[Citation23] The FRAP working solution was composed of 0.3 M acetate buffer (pH 3.6), 10 mM TPTZ solution in 40 mM HCl and 20 mM ferric chloride at a ratio of 25:2.5:2.5 (v/v/v). Sample solution of 0.1 mL was allowed to react with 3 mL of freshly made FRAP working solution for 10 min at 25°C, and then the absorbance was measured at 593 nm. The FRAP value was expressed as millimole ascorbic acid equivalents (mmol AAE/g dried samples). The synthetic antioxidant BHA was used as the reference compound.
α-Amylase inhibitory activity assay
The inhibitory activity of ETRF against α-amylase was determined using the method reported by Chen et al.[Citation24] Sample solution of 0.2 mL at different concentrations was mixed with 0.2 mL of 0.02 M sodium phosphate buffer (PH 6.9 with 6.7 mM NaCl) containing 0.125 mg/mL of α-amylase solution. After pre-incubation at 37°C for 10 min, 0.6 mL of 1% (w/w) starch solution in 0.02 M sodium phosphate buffer (pH 6.9 with 6.7 mM NaCl) was added to the mixtures. The reaction mixture was carried out at 37°C for 3 min and stopped with 1.0 mL of dinitrosalicylic acid color reagent. After that, the test tubes were placed in a water bath at boiling point for 8 min and later cooled down to room temperature. The reaction mixture was then diluted with 3 mL of distilled water and the absorbance was recorded at 540 nm. The inhibitory activity of the sample against α-amylase was calculated as follows: α-amylase inhibitory activity (%) = [(A1–A2)/A1] × 100, where A1 is the absorbance of control (buffer in place of the sample) and A2 is the absorbance in the presence of the sample. The IC50 value is the concentration of the sample that could inhibit 50% of the α-amylase activity. Acarbose was used as the reference compound.
Intrinsic fluorescence analysis
The intrinsic fluorescence spectra were measured using an F-4600 fluorescence spectrophotometer (HITACHI, Tokyo, Japan). The sample (3 mL) was excited at 280 nm with slit widths for excitation and emission being both 5 nm, and spectra were recorded from 300 to 450 nm. A stock solution of α-amylase (5 mg/mL) and the quenchers of ETRF (1 mg/mL) were prepared by dissolving in 0.02 M sodium phosphate buffer (pH 6.9 with 6.7 mM NaCl). The fluorescence intensities were obtained at different concentrations of ETRF and plotted according to the Stern–Volmer equation.[Citation25]
Statistical analysis
Statistical analyses were performed using the SPSS version of 16.0 (Chicago, IL, USA). Values were expressed as mean ± standard deviation of triplicate measurements. Statistical evaluation of the data was carried out by one-way analysis of variance (ANOVA). Differences were considered to be significant when P < 0.05.
Results and discussion
Identification of ETRF by MALDI-TOF-MS
MALDI-TOF-MS is very sensitive to molecular weight, and nowadays is considered a method of choice for identifying the polydisperse hydrolyzable tannins in plants.[Citation26,Citation27] However, a previous study reported by Xiang et al.[Citation28] revealed that MS spectra of the same analyte contain different peak profiles in the presence of different cations when application of MALDI. In addition to the accurate molecular mass determination, the series of fragment ions and complex adducts were also observed in these spectra. The complex spectra of hydrolyzable tannins caused by inappropriate cationization reagent for MALDI might make it difficult to interpret structural properties of complicated hydrolyzable tannins. The effect of cationization reagent on MALDI-TOF-MS of hydrolyzable tannins therefore must be considered. When Cs+ was employed as the cationization reagent for MALDI, Chinese gallotannins gave a relatively simple MALDI-TOF spectrum.[Citation28]
shows the MALDI-TOF mass spectra of ETRF from the leaves of three Sonneratia species recorded as Cs+ adducts in the positive ion reflectron mode. It was clear that the mass spectra had a series of peaks exhibiting a mass distance of 152 Da. On the basis of the report by Reed et al.[Citation14] and Zhang et al.[Citation29], the increment of 152 Da was in accordance with the mass value of one galloyl group unit, and the ETRF were composed essentially of oligomeric ellagitannins, in which two or more core glucose units were cross-linked via dehydrodigalloyl unit. shows the calculated and observed values for Cs+ adducts of ellagitannins and possible monomeric compositions present in ETRF. As can be seen in , the ETRF had similar mass distributions, but different polymer chain length, with the longest for S. caseolaris up to pentamers and the shortest for S. alba up to trimers. In addition, each major peak was always followed by mass signals at a distance of 16 Da ( inset). This gained mass might derive from replacement of two galloyl groups (2 × 152 Da) by one chebuloyl (320 Da). The series of compounds with 2 Da multiples lower than those major peaks were also detected ( inset), which might be explained by substitution of two galloyl groups (2 × 152 Da) by one hexahydroxydiphenoyl group (302 Da). Based on the literatures available, this was the first report on the successful structure elucidation of ellagitannins in three Sonneratia species leaves by MALDI- TOF-MS.
Table 1. Calculated and observed masses for ETRF form the leaves of three Sonneratia species and possible monomeric composition
Identification of acidic hydrolyzates of ETRF by reversed-phase HPLC
The chromatograms for acidic hydrolyzates of ETRF from the leaves of three Sonneratia species analyzed by reversed-phase HPLC are given in –c. By direct comparison of retention times with authentic standards, gallic acid (peak 1) and ellagic acid (peak 2) were found as the major hydrolytic products. Gallic acid and ellagic acid are of particular interest from a dietary viewpoint as it has been reported to have many health-promoting properties.[Citation30] However, published data on their concentrations of various plants are limited. As presented in , the amounts of gallic acid and ellagic acid in the hydrolyzed ETRE ranged from 46.19 ± 1.45 to 102.97 ± 2.09 mg/g and 100.99 ± 2.13 to 253.22 ± 4.87 mg/g, respectively, which the concentration of ellagic acid was significantly higher than that of gallic acid. It suggested that the leaves of three Sonneratia species especially S. apetala and S. caseolaris were probable a potential source of gallic acid and ellagic acid that might find use as food additives or functional food products.
Figure 2. Reversed-phase HPLC chromatograms of ETRE from the leaves of three Sonneratia species after hydrolysis: S. apetala (a), S. caseolaris (b), and S. alba (c); Concentrations of total (d) and free (e) gallic acid and ellagic acid in hydrolyzed and unhydrolyzed ETRE from the leaves of three Sonneratia species. Peaks: 1, gallic acid; 2, ellagic acid; 3 and 4, unidentified compounds
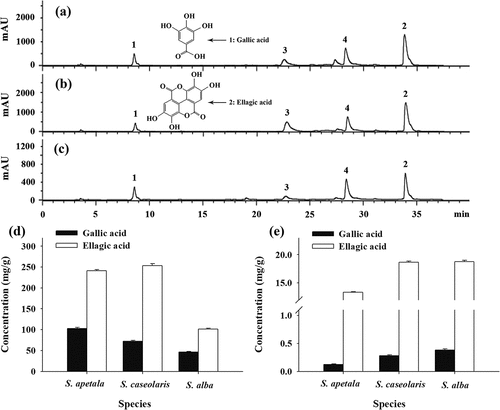
In addition, according to Onodera et al.[Citation31] and Kusuda et al.[Citation32], ellagic acid is mainly in the form of ellagitannins and is bound to glucose in plants. Acid hydrolysis can transform glucosylated and esterified ellagic acid into their aglycones and liberate the parent compounds ellagic acid and gallic acid.[Citation33] In this study, the concentrations of ellagic acid and gallic acid in the unhydrolyzed ETRE from the leaves of three Sonneratia species were also determined and results are displayed in . It was found that the levels of free ellagic acid and gallic acid of the ETRE before acid hydrolysis varied from 13.34 ± 0.11 to 18.75 ± 0.26 mg/g and 0.12 ± 0.01 to 0.38 ± 0.02 mg/g, respectively. Apparently, the free ellagic acid comprised only a minor part of the total ellagic acid pool, indicating that ellagic acid existed primarily as the high molecular weight form of ellagitannins in the ETRF. The chromatograms after acid hydrolysis demonstrated again polymeric ellagitannins were the major constituents for ETRF from the leaves of S. apetala, S. caseolaris, and S. alba.
Antioxidant activity of ETRF
The antioxidant activities of ETRF from the leaves of three Sonneratia species were determined using DPPH and ABTS radical scavenging assays, together with FRAP assay (). Synthetic antioxidant BHA was also examined for comparative purposes. The results obtained from and b clearly demonstrated that all the ETRF could scavenge the DPPH and ABTS radicals in a concentration-dependent manner. The antioxidant activities of ETRF were further evaluated using IC50 values. A lower value of IC50 indicates a higher radical scavenging activity. As can be seen in and e, the IC50 values of ETRF from S. apetala (69.10 ± 0.36 and 47.57 ± 0.99 µg/mL), S. caseolaris (69.39 ± 0.29 and 45.11 ± 0.49 µg/mL), and S. alba (78.89 ± 0.52 and 59.42 ± 0.48 µg/mL) for the DPPH and ABTS assays were all significantly lower than that of BHA (116.52 ± 0.95 and 88.46 ± 0.11 µg/mL). It could be concluded, therefore, that the examined ETRF possessed higher antiradical activities in comparison to the reference compound BHA.
Figure 3. Antioxidant activities of ETRE from the leaves of three Sonneratia species determined by DPPH, ABTS, and FRAP assays. Different letters (a–c) above bars represent significant differences from each other at P < 0.05 level. Butylated hydroxyanisole (BHA) was used as a positive control
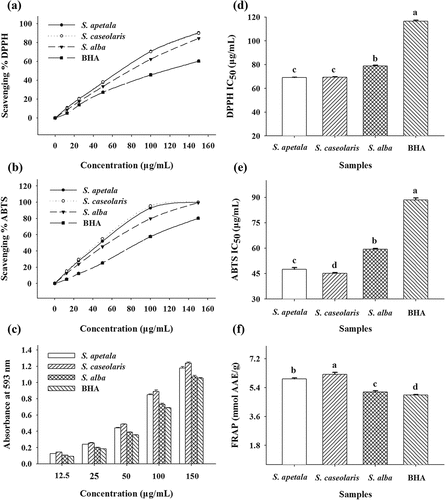
shows the ferric reducing capacity of ETRF from the leaves of three Sonneratia species. It was apparent that the absorbance of the reaction system was highly correlated with the ETRF concentrations. The antioxidant capacity of ETRE was also further estimated by FRAP values expressed as the ascorbic acid equivalents. Similar to the findings of DPPH and ABTS radical scavenging assays, ETRF from S. apetala (5.95 ± 0.06 mmol AAE/g), S. caseolaris (6.22 ± 0.13 mmol AAE/g), and S. alba (5.13 ± 0.07 mmol AAE/g) exhibited higher ferric ion-reducing activities than that of the reference compound BHA (4.95 ± 0.02 mmol AAE/g) (). The results obtained suggested that ETRF from the leaves of three Sonneratia species might be good candidates for further development as antioxidant agents.
α-Amylase inhibitory activity of ETRF
α-Amylase is a key enzyme involved in the digestion of carbohydrates.[Citation34] Inhibition of α-amylase is considered to be an effective strategy for the prevention or treatment of diabetes mellitus.[Citation24,Citation34] The inhibitory effects of ETRF from the leaves of three Sonneratia species on the activity of α-amylase were thus investigated and results are listed in . As observation, all the ETRF significantly inhibited the activity of α-amylase in a concentration-dependent manner (). The ETRF concentrations leading to 50% enzyme activity loss (IC50 values) were estimated to be 10.12 ± 0.08, 7.96 ± 0.05, and 11.96 ± 0.07 µg/mL for S. apetala, S. caseolaris, and S. alba, respectively (). A lower IC50 value is associated with a higher α-amylase inhibitory activity. Compared with acarbose (IC50 = 24.89 ± 1.49 µg/mL) the positive control which is a known α-amylase inhibitor, the examined ETRF exhibited better inhibitory effects. It suggested that the ETRF from the leaves of three Sonneratia species have the potential to be exploited as antidiabetic agents in the future.
Fluorescence quenching of α-amylase by ETRF
For further elucidating the interaction mechanism between α-amylase and ETRF from the leaves of three Sonneratia species, the fluorescence quenching of α-amylase by ETRF was investigated. As can be seen from –c, a fluorescence emission peak of α-amylase (curve a) at near 340 nm was observed after being excited at a wavelength of 280 nm. According to the report by Lakowicz,[Citation25] it belongs to tryptophan (Trp) residues located at protein interior. The addition of increasing concentrations of the ETRF caused a gradual and significant reduction of the fluorescence intensity of α-amylase, indicating that the fluorescence of Trps was quenched by the interaction of ETRF with α-amylase. In addition, a slight blue shift of the maximum emission wavelength from 340 to 337 nm of three fluorescence emission spectra was also found. It suggested that the addition of ETRF resulted in the polarity variation around Trp residues and conformational change of α-amylase.
Figure 5. (a–c) Fluorescence emission spectra of α-amylase in the absence and presence of ETRE from the leaves of three Sonneratia species at 290 k. The concentrations of ETRE for curves a-e were 0, 1.0, 2.0, 5.0, and 8.0 µg/mL, respectively; (d–f) Stern–Volmer plots for α-amylase quenching by ETRE at different temperatures; (g–i) Plots of log[(F0-F)/F] versus log[Q] for the binding of ETRE with α-amylase at different temperatures. [Q] and T represent the concentrations of ETRE and temperature, respectively
![Figure 5. (a–c) Fluorescence emission spectra of α-amylase in the absence and presence of ETRE from the leaves of three Sonneratia species at 290 k. The concentrations of ETRE for curves a-e were 0, 1.0, 2.0, 5.0, and 8.0 µg/mL, respectively; (d–f) Stern–Volmer plots for α-amylase quenching by ETRE at different temperatures; (g–i) Plots of log[(F0-F)/F] versus log[Q] for the binding of ETRE with α-amylase at different temperatures. [Q] and T represent the concentrations of ETRE and temperature, respectively](/cms/asset/d4cf9f4f-e856-435e-afba-671aa8081728/ljfp_a_1675693_f0005_oc.jpg)
The mechanism of fluorescence quenching can be collisional/dynamic (diffusion-controlled) quenching or static quenching (formation of complex between fluorophore and quencher).[Citation25] In order to speculate the possible quenching mechanism, the fluorescence data were analyzed using the Stern–Volmer equation: F0/F = 1 + Ksv [Q], where F0 and F are fluorescence intensities of α-amylase in the absence and presence of ETRF, [Q] is the ETRF concentration, Ksv is referred to as the Stern–Volmer quenching constant. The Stern–Volmer plots for α-amylase fluorescence quenching by ETRF from the leaves of three Sonneratia species are shown in –f. As observation, plots of F0/F versus [ETRF] at different temperatures all exhibited good linear relationships (R2 > 0.956), implying that there was one quenching type (static or dynamic) involved in the interaction of α-amylase with ETRF. The Stern–Volmer quenching constants at 290 K and 310 K were further calculated from the plot and values are listed in . It was found that the Ksv all decreased with increasing temperature, which is an indication that the probable quenching mechanism of α-amylase by ETRE from the leaves of three Sonneratia species was a static quenching process and the complexes between α-amylase and ETRE might be formed.
Table 2. The quenching parameters of ETRF form the leaves of three Sonneratia species against α-amylase
For static quenching, the binding constants (Ka) and the number of binding sites (n) between α-amylase and ETRE from the leaves of three Sonneratia species can also be assayed and calculated according to the double-logarithm equation: log[(F0-F)/F] = logKa + n log[Q]. As observed in –i, plots of log[(F0-F)/F] versus log[Q] for α-amylase fluorescence quenching by ETRF gave straight lines at different temperatures, whose slope equals to n and the vertical intercept equals to logKa. The calculated values of Ka and n at 290 and 310 K are presented in . It was clearly seen that the binding constants of α-amylase with ETRE all decreased with rising temperature and this indicated that high temperature weakened the stability of the complexes formed between them. The binding site values of n listed in , suggested that there was probably only one binding site in α-amylase for ETRE from the leaves of S. apetala and S. alba, and two binding sites on α-amylase for S. caseolaris leaves ETRE.
Conclusion
This study has shown that MALDI-TOF-MS is a powerful tool for the analysis of ellagitannins in the leaves of S. apetala, S. caseolaris, and S. alba. The ETRE isolated from three Sonneratia species were identified to be a complex mixture of oligomeric ellagitannins. Gallic acid and ellagic acid were confirmed as the major hydrolytic products of ETRE after acid hydrolysis followed by reversed-phase HPLC. The ETRE inhibited the activity of α-amylase strongly and could quench the intrinsic fluorescence of α-amylase by a single static quenching mechanism. Moreover, the ETRF also exhibited greater antioxidant activities, as measured by DPPH, ABTS, and FRAP assays than that of synthetic antioxidant BHA. In conclusion, the results from this study would not only point out that the ETRF from three Sonneratia species leaves might be interesting candidates for the development of antioxidant agents and α-amylase inhibitors but also further increase the applicability of MALDI-TOF-MS in the analysis of hydrolzsable tannins.
Additional information
Funding
References
- Mattei, J.; Malik, V.; Wedick, N. M.; Hu, F. B.; Spiegelman, D.; Willett, W. C.; Campos, H. Reducing the Global Burden of Type 2 Diabetes by Improving the Quality of Staple Foods: The Global Nutrition and Epidemiologic Transition Initiative. Globalization Health 2015, 11, 23. DOI: 10.1186/s12992-015-0109-9.
- Hernes, P. J.; Benner, R.; Cowie, G. L.; Goni, M. A.; Bergamaschi, B. A.; Hedges, J. I. Tannin Diagenesis in Mangrove Leaves from a Tropical Estuary: A Novel Molecular Approach. Geochim. Cosmochim. Acta 2001, 65(18), 3109–3122. DOI: 10.1016/S0016-7037(01)00641-X.
- Haslam, E.; Cai, Y. Plant Polyphenols (Vegetable Tannins): Gallic Acid Metabolism. Nat. Prod. Rep. 1994, 11(1), 41–66.
- Quideau, S.; Deffieux, D.; Douat-Casassus, C.; Pouysegu, L. Plant Polyphenols: Chemical Properties, Biological Activities, and Synthesis. Angew. Chem. Int. Edit. 2011, 50(3), 586–621. DOI: 10.1002/anie.201000044.
- Clifford, M. N.; Scalbert, A. Ellagitannins-Nature, Occurrence and Dietary Burden. J. Sci. Food Agric. 2000, 80(7), 1118–1125. DOI: 10.1002/(ISSN)1097-0010.
- Quideau, S. Chemistry and Biology of Ellagitannins: An Underestimated Class of Bioactive Plant Polyphenois; World Scientific: Singapore, 2009.
- Gali-Muhtasib, H. U.; Yamout, S. Z.; Sidani, M. M. Plant Tannins as Inhibitors of Hydroperoxide Production and Tumor Promotion Induced by Ultraviolet B Radiation in Mouse Skin in Vivo. Oncol. Rep. 1999, 6(4), 847–853. DOI: 10.3892/or.6.4.847.
- Zhang, L. L.; Lin, Y. M.; Zhou, H. C.; Wei, S. D.; Chen, J. H. Condensed Tannins from Mangrove Species Kandelia candel and Rhizophora mangle and Their Antioxidant Activity. Molecules 2010, 15(1), 420–431. DOI: 10.3390/molecules15010420.
- Wei, S. D.; Lin, Y. M.; Liao, M. M.; Zhou, H. C.; Li, Y. Y. Characterization and Antioxidative Properties of Condensed Tannins from the Mangrove Plant Aegiceras corniculatum. J. Appl. Polym. Sci. 2012, 124(3), 2463–2472. DOI: 10.1002/app.35258.
- Wei, S. D.; Zhou, H. C.; Lin, Y. M. Antioxidant Activities of Extract and Fractions from the Hypocotyls of the Mangrove Plant Kandelia candel. Int. J. Mol. Sci. 2010, 11(10), 4080–4093. DOI: 10.3390/ijms11104080.
- Zhou, H. C.; Tam, N. F. Y.; Lin, Y. M.; Ding, Z. H.; Chai, W. M.; Wei, S. D. Relationships between Degree of Polymerization and Antioxidant Activities: A Study on Proanthocyanidins from the Leaves of a Medicinal Mangrove Plant Ceriops tagal. PloS One 2014, 9(10), e107606. DOI: 10.1371/journal.pone.0107606.
- Bandaranayake, W. M. Traditional and Medicinal Uses of Mangroves. Mangroves Salt Marshes 1998, 2(3), 133–148. DOI: 10.1023/A:1009988607044.
- Pasch, H.; Pizzi, A.; Rode, K. MALDI-TOF Mass Spectrometry of Polyflavonoid Tannins. Polymer 2001, 42(18), 7531–7539. DOI: 10.1016/S0032-3861(01)00216-6.
- Reed, J. D.; Krueger, C. G.; Vestling, M. M. MALDI-TOF Mass Spectrometry of Oligomeric Food Polyphenols. Phytochemistry 2005, 66(18), 2248–2263. DOI: 10.1016/j.phytochem.2005.05.015.
- Monagas, M.; Quintanilla-Lopez, J. E.; Gomez-Cordoves, C.; Bartolome, B.; Lebron-Aguilar, R. MALDI-TOF MS Analysis of Plant Proanthocyanidins. J. Pharm. Biomed. Anal. 2010, 51, 358–372. DOI: 10.1016/j.jpba.2009.03.035.
- Kandil, F. E.; Grace, M. H.; Seigler, D. S.; Cheeseman, J. M. Polyphenolics in Rhizophora mangle L. Leaves and Their Changes during Leaf Development and Senescence. Trees 2004, 18(5), 518–528. DOI: 10.1007/s00468-004-0337-8.
- Oo, C. W.; Pizzi, A.; Pasch, H.; Kassim, M. J. Study on the Structure of Mangrove Polyflavonoid Tannins with MALDI-TOF Mass Spectrometry. J. Appl. Polym. Sci. 2008, 109(2), 963–967. DOI: 10.1002/(ISSN)1097-4628.
- Song, W.; Zhu, X. F.; Ding, X. D.; Yang, H. B.; Qin, S. T.; Chen, H.; Wei, S. D. Structural Features, Antioxidant and Tyrosinase Inhibitory Activities of Proanthocyanidins in Leaves of Two Tea Cultivars. Int. J. Food Prop. 2017, 20(6), 1348–1358. DOI: 10.1080/10942912.2016.1209682.
- Wei, S. D.; Chen, H.; Lin, Y. M. Comparison of Chemical Compositions and Antioxidant Activities of Condensed Tannins from Different Parts of Calliandra haematocephala. J. Wood Chem. Technol. 2015, 35(3), 193–206. DOI: 10.1080/02773813.2014.919596.
- Oszmianski, J.; Wojdylo, A.; Lamer-Zarawska, E.; Swiader, K. Antioxidant Tannins from Rosaceae Plant Roots. Food Chem. 2007, 100(2), 579–583. DOI: 10.1016/j.foodchem.2005.09.086.
- Delfanian, M.; Kenari, R. E.; Sahari, M. A. Antioxidant Activity of Loquat (Eriobotrya japonica Lindl.) Fruit Peel and Pulp Extracts in Stabilization of Soybean Oil during Storage Conditions. Int. J. Food Prop. 2015, 18(12), 2813–2824. DOI: 10.1080/10942912.2015.1013635.
- Zhou, X. L.; Zhou, M.; Liu, Y.; Ye, Q.; Gu, J.; Luo, G. Y. Isolation and Identification of Antioxidant Compounds from Gynura bicolor Stems and Leaves. Int. J. Food Prop. 2016, 19(1), 233–241. DOI: 10.1080/10942912.2014.983607.
- Wei, S. D.; Chen, H.; Yan, T.; Lin, Y. M.; Zhou, H. C. Identification of Antioxidant Components and Fatty Acid Profiles of the Leaves and Fruits from Averrhoa carambola. LWT-Food Sci. Technol. 2014, 55(1), 278–285. DOI: 10.1016/j.lwt.2013.08.013.
- Chen, H.; Sun, K. K.; Yang, Z. N.; Guo, X. H.; Wei, S. D. Identification of Antioxidant and Anti-α-amylase Components in Lotus (Nelumbo nucifera, Gaertn.) Seed Epicarp. Appl. Biochem. Biotechnol. 2019, 187(3), 677–690. DOI: 10.1007/s12010-018-2844-x.
- Lakowicz, R. J. Principles of Fluorescence Spectroscopy, 3rd ed.; Springer: New York, 2006.
- Pasch, H.; Pizzi, A. Considerations on the Macromolecular Structure of Chestnut Ellagitannins by Matrix-assisted Laser Desorption/Ionization-Time-of-Flight Mass Spectrometry. J. Appl. Polym. Sci. 2002, 85(2), 429–437. DOI: 10.1002/(ISSN)1097-4628.
- Radebe, N.; Rode, K.; Pizzi, A.; Giovando, S.; Pasch, H. MALDI-TOF-CID for the Microstructure Elucidation of Polymeric Hydrolysable Tannins. J. Appl. Polym. Sci. 2013, 128(1), 97–107. DOI: 10.1002/app.38156.
- Xiang, P.; Lin, Y. M.; Lin, P.; Xiang, C.; Yang, Z.; Lu, Z. Effect of Cationization Reagents on the Matrix-assisted Laser Desorption/Ionization Time-of-Flight Mass Spectrum of Chinese Gallotannins. J. Appl. Polym. Sci. 2007, 105(2), 859–864. DOI: 10.1002/(ISSN)1097-4628.
- Zhang, L. L.; Lin, Y. M. Antioxidant Tannins from Syzygium cumini Fruit. Afr. J. Biotechnol. 2009, 8(10), 2301–2309.
- Masamune, A.; Satoh, M.; Kikuta, K.; Suzuki, N.; Satoh, K.; Shimosegawa, T. Ellagic Acid Blocks Activation of Pancreatic Stellate Cells. Biochem. Pharmacol. 2005, 70(6), 869–878. DOI: 10.1016/j.bcp.2005.06.008.
- Onodera, K.; Hanashiro, K.; Yasumoto, T. Camellianoside, a Novel Antioxidant Glycoside from the Leaves of Camellia japonica. Biosci. Biotechnol. Biochem. 2006, 70(8), 1995–1998. DOI: 10.1271/bbb.60112.
- Kusuda, M.; Hatano, T.; Yoshida, T. Water-soluble Complexes Formed by Natural Polyphenols and Bovine Serum Albumin: Evidence from Gel Electrophoresis. Biosci. Biotechnol. Biochem. 2006, 70(1), 152–160. DOI: 10.1271/bbb.70.152.
- Daniel, E. M.; Krupnick, A.; Heur, Y. H.; Blinzler, J. A.; Nims, R. W.; Stoner, G. D. Extraction, Stability and Quantification of Ellagic Acid in Various Fruits and Nuts. J. Food Compos. Anal. 1989, 2(4), 338–349. DOI: 10.1016/0889-1575(89)90005-7.
- Tsujita, T.; Shintani, T.; Sato, H. α-Amylase Inhibitory Activity from Nut Seed Skin Polyphenols. 1. Purification and Characterization of Almond Seed Skin Polyphenols. J. Agric. Food Chem. 2013, 61(19), 4570–4576. DOI: 10.1021/jf400691q.