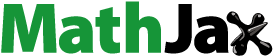
ABSTRACT
This study investigated the influence of pressure, shear, temperature, and their interactions on the inactivation of two bacteria – Lactobacillus brevis and Bacillus cereus spores. Cell suspension of L. brevis (1.6 × 1010 CFU/mL) and spore suspension of B. cereus (3.2 × 108 CFU/mL) were suspended in HEPES buffer (pH 7.3) and subjected to ultra-shear treatment (UST) at 400 MPa, process temperatures of 40 and 70°C, and flow rate of 0.0012 ± 0.0003 kg/s. In separate equipment, thermal (0.1 MPa-70°C-0/5 min) and high-pressure processing (HPP-400 MPa-40°C and 70°C-0/5 min) experiments were carried out to evaluate thermal only, pressure only, and combined pressure-thermal effects. Pressure-only treatment at 400 MPa for 0 min (come-up time) at 70°C resulted in 8.4 and 2.3 log reductions of L. brevis cells and B. cereus spores, respectively. After UST treatment at 70°C, L. brevis and B. cereus spores were reduced by 7.1 and 1.6 logs, respectively. Both strains showed different sensitivities to thermal, pressure, shear, and their combinations. B. cereus spores were relatively more resistant to HPP and UST treatments. Three valve geometries, namely, ultra-shear valve, needle valve, and tubular valve, were used to compare UST treatment effect on L. brevis and B. cereus spores at 40 and 70°C. UST treatment using the ultra-shear valve produced inactivation of 2.0 and 7.1 log cycles for L. brevis during 40 and 70°C process temperatures, respectively, which was relatively higher than needle valve and tubular valve. This higher inactivation could be attributed to the smaller valve gap and higher shear rate in the ultra-shear valve. Further, the comparatively higher inactivation at 70°C UST (7.1 log) emphasized the role of temperature on inactivation during UST. For both L. brevis and B. cereus, the inactivation was least in the tubular valve with relatively lesser shear rate, which indicated the desirable contribution of high shear rate for microbial inactivation. The information from this study contributes to the development of safe harbor UST process conditions for liquid foods.
Introduction
Ultra-shear technology (UST), also referred as high-pressure homogenization (HPH), is a continuous high-pressure processing method to preserve liquid beverages while retaining the health-promoting nutrients.[Citation1,Citation2] During a typical UST treatment, the liquid food is pressurized up to 400 MPa and then depressurized by passing through a shear valve. The pressure drop across the valve facilitates the conversion of pressure energy to kinetic energy. The kinetic energy is subsequently expended as temperature increase in the product, energy spent on modification of properties of liquid food matrix, as well as heat loss to the surrounding environment.[Citation3,Citation4] Moreover, when the microbial cells pass through a shear valve, it may experience shear, turbulence, cavitation, and impingement, which induce breakdown or alteration of microbial cell walls and cause cell injury or death.[Citation5–7] Thus, the microbes in the fluid are subjected to lethal factors such as high pressure, temperature, shear, and their interactions.[Citation8] Many researchers have evaluated the inactivation efficacy of UST treatment on different microorganisms.[Citation1,Citation7,Citation9–11] These studies considered the cumulative effect of various process variables during UST treatments on the microorganisms. Systematic studies to understand the relative effect of individual process variables, such as pressure, temperature, shear, and their interactions on microbial inactivation are lacking.
The main cause for microbial inactivation during ultra-shear treatment is the disintegration of the microbial cells in the fluid has been attributed to shear, turbulence, or cavitation experienced by the food matrix.[Citation8,Citation12] The key to understand the mechanism of microbial inactivation is to know the magnitude of these phenomena in the shear valve and the microbial cell wall resistance to these phenomena. The dominating mechanism for cell droplet breakage may be influenced by factors such as fluid flow, fluid characteristics, and cell structure. Further, the cell wall disruption at a certain pressure is significantly influenced by the shear valve geometry.[Citation13] UST treatment is accomplished by creating a fluid flow restriction using different shear valve geometries including ball, needle, or tubular valve configurations. These different geometries alter the energy dissipated in fluid and the degree of shear on the suspended microbial cells. Considering the small dimension and intense pressure in the shear valve, in situ measurement of velocity in these different geometries is very challenging.[Citation14] Hence, researchers employ dimensionless numbers such as Reynolds number, Weber number, capillary number, and cavitation number that take into consideration valve size, flow regime, and fluid dynamic behavior during shear discharge. These calculations were subsequently validated using experimental data to better understand the mechanism of microbial inactivation by UST.
Lactobacillus brevis is a microorganism responsible for spoilage of juices,[Citation15] salads,[Citation16] sauces,[Citation17] milk,[Citation18] and beer.[Citation19] This microorganism demonstrates a relatively high resistance to pressure (e.g., D-values range from 11 to 1160 s in phosphate buffer at pressure range of 530–400 MPa, process temperatures of 34.3–38.5°C).[Citation16,Citation20,Citation21] Bacillus cereus, a facultatively anaerobic spore former, is responsible for many foodborne illnesses and spoilage of several foods such as poultry, milk, and dairy products.[Citation18,Citation22]
The objective of the present study was to evaluate the contributions of various process parameters (pressure, shear, temperature, holding time, and their interactions) and different shear valve geometries during UST treatment on the inactivation of the two representative microorganisms, L. brevis and B. cereus spores.
Materials and methods
Culture preparation
L. brevis was obtained from the Department of Food Science and Technology, The Ohio State University, and stored at – 80°C in trypticase soy broth (TSB) with 20% (v/v) glycerol. A loop of bacteria was streak plated on Lactobacilli MRS agar from the stock culture and incubated at 37°C for 24 h. A single colony was selected from the agar plate and transferred to 100 mL MRS broth with shaking incubation at 200 rpm for 24 h at 37°C. A portion (25 mL) of bacterial culture was centrifuged at 8000 × g for 10 min at 4°C and the supernatant was discarded. Then, bacteria pellet was suspended with HEPES buffer (pH 7.3) to reach a final concentration of 1.6 × 1010 CFU/mL.
Freeze-dried B. cereus culture ATCC9818 was purchased from ATCC (Manassas, VA, USA). B. cereus spores were prepared as per procedure described by Daryaei et al.[Citation23] with modifications. In brief, a small aliquot of freeze-dried powder was added into nutrient broth and incubated at 30°C for 48 h. A loop of previous culture was streak plated on nutrient agar (NA) and incubated at 30°C for another 48 h. A single colony was transferred to TSBYE and incubated at 30°C for 48 h. Then, 100 µL of the previous culture was spread plated on sporulation media (NA with 10 mg/L MnSO4) and incubated aerobically at 30°C for 7 days. Light phase-contrast microscopy was used to check the formation progress of spores on the last day. Once > 90% of the viewed cells became spores, the bacteria lawn was harvested by flooding the plates with cold and sterile dd H2O, followed by centrifugation at 14000 × g for 10 min at 4°C to discard the supernatant. The bacterial pellet was washed for five times with a down-speed centrifuge at 4°C from 14000 × g to 10000 × g for 10 min. To get rid of the remaining vegetative cells, a heat shock treatment was performed between the 1st and 2nd washing by heating the bacterial culture in a water bath for 15 min at 80°C. Finally, washed spores were suspended in a small amount of cold and sterile dd H2O and stored at – 20°C before usage. The spores were mixed with HEPES buffer right before treatment with a final concentration of 3.2 × 108 CFU/mL.
Experimental design
To study the influence of tested lethal factors on L. brevis cells and B. cereus spores, bacterial suspensions in HEPES buffer were subjected to three treatments: thermal processing (TP), high pressure processing (HPP), and ultra-shear technology (UST). At process temperatures ≤ 40°C, the contribution of heat to microbial lethality is considered negligible or minimal.[Citation24] Thus, HPP (400 MPa-0/5 min) and UST (400 MPa, 0 min) experiments carried out at 40°C help to study the influence of pressure only and pressure + shear effects on bacteria inactivation, respectively. At 70°C process temperature, TP (0.1 MPa, 0/5 min), HPP (400 MPa-0/5 min) or UST (400 MPa-0 min) experiments were carried out to evaluate thermal only, pressure + thermal, and pressure + thermal + shear lethal effects on bacteria, respectively. The summary of the experiments performed is shown in .
Thermal processing
Thermal processing experiments were carried out using capillary tube method.[Citation25,Citation26] Bacterial suspension (40 µL) was injected into a sterile capillary tube (100 mm long, 0.25–0.4 mm wall thickness, 1 mm inner diameter; Kimbel Chase, Vineland, NJ) and sealed with hot flame. Then, capillary tubes were immersed into a 35 L circulating bath (NESLAB EX-35 Digital One, Thermo Fisher Scientific, Inc., Waltham, MA) filled with mineral oil preheated to 70°C. The come-up time (CUT), i.e., the time taken by bacterial suspension in the capillary tube to reach the target temperature, was 10s (0 min). The capillary tube was fully immersed into the oil bath for 10s (0 min) or 5 min, and then cooled down in an ice-water bath immediately to stop the thermal treatment. Then, the capillary tube was transferred into another tube with 70% ethanol and kept for 30s. Finally, the capillary tube was moved to another tube filled with sterile dd H2O to rinse off the ethanol on the surface. Treatments were completed within 2 h before enumeration.
High-pressure processing
High-pressure processing experiments were performed in a laboratory scale high pressure tester (PT-1, Avure Technologies, Inc., Kent, WA) as described by Xu et al.[Citation27] First, 500 µL of inoculated sample was packaged in a UV pre-sterilized plastic pouch (0.04 m × 0.04 m) and heat sealed with attention to avoid air bubbles. The pouch was then loaded inside a 10 mL-polypropylene syringe (model 309604, BD) and the remaining volume of the syringe was filled with water. The syringe with the microbial pouch was then held inside a water bath (Isotemp 928, Fisher Scientific) to equilibrate to target initial temperature before pressure treatment. The initial sample temperatures, determined based on heat of compression of fluid, were 20 and 50°C for HPP treatment at process temperatures of 40 and 70°C, respectively. Upon initial temperature equilibration, the syringe was immediately loaded in the pressure chamber, and pressurized to the target pressure of 400 MPa. After reaching the target pressure and temperature (40°C or 70°C), the pressure was held for 0 min (CUT) or 5 min and depressurized immediately. After depressurization, pouches with sample were removed quickly and cooled in an ice-water bath to stop any thermal inactivation.
Ultra-shear treatment
Ultra-shear treatment was carried out at a pressure of 400 MPa and process temperatures of 40 and 70°C in a laboratory scale UST tester (PBI, Easton, MA, USA) using the procedure described by Janahar et al.[Citation28] The bench scale UST equipment involves feed reservoir (a syringe), pressure generating system, pressure chamber, temperature-controlled shear valve, holding tube, cooling heat exchanger, and processed product reservoir. In the UST tester, the liquid suspension is pressurized up to 400 MPa and passed through a tiny gap in a shear valve. For 70°C UST treatment, the shear valve was electrically heated to desired process temperature (70°C) to prevent heat loss from fluid to the valve body. During 40°C UST treatment, a cooling pad was wrapped around the shear valve in order to instantaneously remove the heat generated by shear. Tests were carried out at a flow rate of 0.0012 ± 0.0003 kg/s. Hold time of 0.5 s (0 min) at process temperatures was realized by a 5 ft (1.524 m) long holding tube (0.03 inches/0.762 mm inner diameter) at the exit of the shear valve. Temperature of the holding tube was controlled at 40 or 70°C by suspending the hold tube inside a water bath maintained at target process temperature. A cooling heat exchanger is placed at the exit of the holding tube to instantly cool down the treated samples under 5°C. Pressure and temperature at various locations were measured by pressure transducer and thermocouples, respectively, and the data were recorded by a data acquisition system (PBI, Easton, MA, USA).
For UST treatment, bacterial suspension was fed into the UST system for treatment involving 5 UST cycles. One cycle typically consists of sample pressurization followed by depressurization (discharge) of ≈2.5 to 3 mL of fluid sample through the UST system. Then, the equipment is designed to carry out subsequent UST cycles in manual or automatic mode. Two independent treatments were performed with duplicate samples collected for each treatment. The treated samples were collected in sterile 15 mL tubes and placed in ice-water bath until enumeration.
To eliminate cross-contamination, the UST equipment was sanitized between treatments using a sanitation protocol developed for the purpose. The sanitation procedure involves circulation of hot water (80°C), 1% (w/v) Tergazyme solution (Alconox, White Plains, NY, USA), and 0.05% (v/v) peracetic acid (Sigma–Aldrich, St Louis, MO, USA) in sequential order through the benchtop UST unit, with each step lasting for 2 min. The final total plate count from the outlet water after the sanitation process was less than 100 CFU/mL.
Pressure and thermal dosage during come-up time for HPP and UST treatments
To determine the influence of pressure-temperature rise rates on microbial inactivation during HPP and UST treatments, the areas under the CUT for pressure-time and temperature-time graphs were computed. The areas under the curve of pressure-time and temperature-time represent the pressure and thermal dosage on the microbes during the CUT. The areas were calculated by numerical integration on the data plot by trapezoidal rule using OriginPro 2019b software version 9.6.5.169 (Origin-Lab Corporation, USA). The area under the curve between the endpoints of the CUT interval a and b, for pressure and temperature history can be approximated by the given formula,
Where x0 = a, x1 through xn are the equally spaced x-coordinates of the right edges of the trapezoids 1 through n, i.e. incremental times in the graph and n is the number or trapezoids.
Recovery and enumeration
All the treated samples were held in the ice-water bath and the enumeration of survived bacteria were done within 2 h after each treatment. For TP, the rinsed capillary tube with treated sample (40 µL) was placed into a sterile 15 mL centrifuge tube with 360 µL 0.1% (v/v) peptone water. The capillary tube was crushed using a sterile blunt-end metal stick and its contents were vortexed sufficiently to mix the treated sample with diluted buffer. For HPP, 500 µL of sample was transferred into a sterile 1.5 mL centrifuge tube. The aliquot (500 µL) of sample was mixed with 4.5 mL 0.1% (w/v) peptone water separately to establish a 10-fold dilution. For UST, 1 mL of treated samples were transferred into 9 mL 0.1% (w/v) peptone water to obtain 10-fold dilution. To enumerate L. brevis, 100 µL of suitable diluted sample was pour plated on lactobacilli MRS agar and incubated at 37°C for 72 h. Before enumeration of B. cereus spores, treated samples were heat shocked to kill any pressure-induced germinated cells by immersing the HPP- or UST-treated sample into a water bath at 80°C for 15 min, then serial 10-fold dilutions were prepared. For enumeration, 100 µL of suitable diluted sample was pour plated using nutrient agar and inoculated plates were incubated at 30°C for 24 h.
Valve geometry
To investigate the influence of shear valve geometry on the microbial inactivation, three types of valves were used: ultra-shear valve, needle valve, and tubular valve (). Ultra-shear valve consists of a spherical ceramic ball placed over a circular valve seat. A valve pin adjusted by a micrometer setting is used to alter the force of the ball over the valve seat. When the pressure on the fluid overcomes the force on the ball, the fluid flows between the ball and valve seat. Needle valve consists of a wedge-shaped ceramic needle placed over a circular and conical valve seat. A revolving handle is used to adjust the ceramic needle to alter the valve gap. Tubular valve involves a small and long tubular hole and an internal insert made of a tight-fitting ceramic rod pushed into the tubular hole. This setup creates a very restrictive flow path in the annular space. The different geometries of valves could expose the bacterial cells to different shear magnitudes when the fluid passed through the valve.
Figure 2. Schematics of the valve geometries tested (a) Ultra-shear valve, (b) Needle valve, (c) Tubular valve. (Arrows show the fluid direction through the valve).
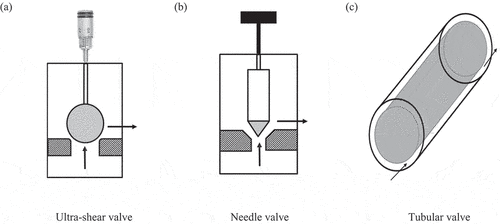
To evaluate the valve geometry’s effects on the microbial inactivation, UST treatment was performed using the three valves mentioned above at a pressure of 400 MPa at temperatures of 40 and 70°C with no holding tube. The flow rates during UST treatment using ultra shear valve, needle valve, and tubular valve were 0.0012 ± 0.0003 kg/s, 0.0013 ± 0.0006 kg/s, and 0.0010 ± 0.0005 kg/s, respectively.
Influence of fluid dynamic characteristics in different valve geometries on microbial inactivation
To determine the influence of valve geometry on microbial inactivation, it is essential to understand the mechanism of cell disruption facilitated by the different shear valve geometries. Differences between valve geometry were characterized in terms of the dimensions of the valves, i.e., diameter of ball/needle in shear valve, diameter of valve seat, cross sectional area of fluid flow, length of fluid acceleration, etc. The maximum theoretical exit velocity ( of fluid through the valve is given by the following equation relating the pressure (P) and density (ρ) of the fluid.
For the pressure of 400 MPa and assumed density of 1060.4 kg/m3 for the fluid, the maximum velocity was calculated as 868.58 m/s. Assuming the average velocity is , the average velocity was determined as 434.29 m/s. The average velocity and flow rate (measured for each valve) were used to determine the cross-sectional area and valve gap of ultra-shear valve and needle valve. The valve gap of tubular valve is pre-determined. The average velocity was then used to determine the shear rate of fluid through the shear valve. The energy applied by the UST process on the microorganisms to effect inactivation is represented by the energy density Ev, as given by the relation below.[Citation13]
where, ∆P is the total pressure drop (MJ/m3) and n is the number of passes. The number of passes used for all the valves is one, since the product exiting the shear valve is not passed again through the valve. The energy distributed, Ed, on fluid volume passing through the valve gap (MJ) is determined as below.
when the volume of fluid passing through the shear valve is lesser, the energy distributed on the fluid is lesser and vice versa. During shear treatment, the microbial suspension is subjected to high pressure energy, which is converted to kinetic energy due to the sudden pressure drop across the shear valve. The kinetic energy is dissipated as fluid-dynamic stresses, which take the form of turbulence, elongational stress, shear stress, and cavitation. The degree of disruption and inactivation of the microbial cells depends on the physical resistance of the cells to the fluid-dynamic characteristics under shear treatment. These fluid dynamic parameters can be estimated by the dimensionless parameters discussed below.
Reynolds number (Re): In low viscosity fluids such as microbial suspensions, one of the important mechanisms of microbial inactivation is the turbulence of fluid in shear valve and inertial forces in the highly turbulent region.[Citation14,Citation29] Turbulence in the fluid flow region of shear valve can be expressed by the Reynolds number.
where, ρ is the fluid density (kg/m3), uvalve is the mean velocity (m/s) in the valve gap, dvalve is the valve gap size (m), and η is the fluid viscosity (kg/m.s).
Weber number (We): When the microbial suspension flows through the tiny gap of shear valve, the microbial cells can be subjected to extensional stress. The resistance of microbial cells to the stress depends on its physical strength. In viscous fluids, the extensional shear stress in the laminar flow is the primary cell disruption mechanism and it is estimated by the Weber number.[Citation30]
Here, α is the fluid acceleration (s−1), which is calculated by dividing the average fluid velocity in valve by the length over which fluid acceleration happens.[Citation14] Donsì et al.[Citation13] assumed the length of acceleration as ten times the valve gap size. In the present study, for the ultra-shear valve, since the length of acceleration is not measurable, it is assumed as ten times the valve gap size. The length of acceleration for needle valve and tubular valve were 0.001 m and 0.0114 m, respectively, as measured by valve dimensions. dcell is the average diameter of cell, assumed as 50 nm for calculations based on particle size measurements. σ is the cell resistance to stress tension and it is assumed to be 8 N/m based on the value for S. cerevisiae as proposed by Kleinig and Middelberg.[Citation30]
Capillary number (Ca): Shear stress is another factor that contributes to microbial disruption and it becomes dominant mechanism in viscous microbial suspensions.[Citation13,Citation31] The shear stress is represented by capillary number which is the ratio of the shear stress and the cell wall resistance.[Citation32]
Cavitation number (K): The fluid velocity and pressure drop in the shear valve favor cavitation effect, due to the formation and collapse of vapor bubbles in the turbulent regions.[Citation33] Thus, cavitation can also present an important mechanism of microbial inactivation. Cavitation is expressed by cavitation number, which is the ratio of the difference between local absolute pressure downstream of the shear valve (P2) and the vapor pressure (Pv) at the exit of shear valve to the specific kinetic energy, depending on mean valve velocity (uvalve).[Citation30]
For estimation of the above parameters, the test fluid was assumed as water. The properties of water (in the shear valve gap at average pressure of 200 MPa and average temperature of 50°C) were estimated using National Institute of Standards and Technology (NIST) standard reference database – REFPROP program version 10.0.[Citation34]
Scanning electron microscopy (SEM)
A small aliquot of untreated, HPP-, or UST-treated sample was fixed into 2.5% (v/v) paraformaldehyde/glutaraldehyde/0.1 M phosphate buffer for 72 h. Fixed samples were kept at room temperature (23°C) for the first 2 h and then put at 4°C for rest of the time. After fixation, the biosamples were rinsed with 0.1 M phosphate buffer two times (10 min, each time), followed by centrifuge at 8000 × g at 4°C for 10 min. Dehydration of samples were performed in a graded ethanol series (30%, 50%, 75%, 95%, 100%) and Hexamethyldisilazane for 10 min. Dehydrated samples were spread in a thin layer on double coated carbon conductive tabs and gold coated to a thickness of 6 nm in a vacuum evaporator. The microstructures of treated samples were examined using scanning electron microscopy at the Center for Electron Microscopy and Analysis (CEMAS), The Ohio State University. At least 5 representative images were captured for each sample at 20,000× magnification.
Statistical analysis
Survivors were enumerated from triplicate samples for each treatment. The significance of average survivors expressed as log CFU/mL with respect to different treatments, process temperature, holding time, and their interactions was investigated by general linear model (GLM) univariate ANOVA at a significance level of 0.05 using SPSS version 28.0.1.1 (IBM, Armonk, NY). Similarly, the significance of UST temperature and valve geometries on average survivors were evaluated as well.
Results and discussion
Pressure- temperature history
shows the pressure-temperature history during HPP treatments at 400 MPa for 0 min. The CUT for HPP to reach 40 and 70°C at 400 MPa were 45.50 s and 48.50 s, respectively (). The average process temperature during HPP treatment was 40 ± 0.73°C () and 70 ± 0.87°C ().
Figure 3. Pressure and temperature histories during different processing treatments (a) HPP-400 MPa-40°C-0 min, (b) HPP-400 MPa-70°C-0 min, (c) UST-400 MPa-40°C-0 min, (d) UST-400 MPa-70°C-0 min.
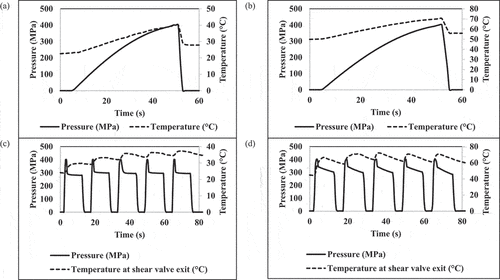
Table 1. Pressure and thermal dosage1 during come-up time for high pressure processing and ultra-shear technology treatments.
The pressure-thermal histories for UST treatments at 40 and 70°C were shown in . During UST treatment, during pressure discharge, the pressure drop from 400 MPa to 0.1 MPa across the valve. Pressure energy subsequently converted into kinetic energy. Part of the energy is expended in the form intense shear, turbulence, impingement, or cavitation, while portion of the energy contributes to temperature rise in the fluid[Citation4] and the remainder energy may be wasted in the form of heat loss to the environment. The temperature increase during UST is primarily due to temperature rise by instant pressure discharge in shear valve (~15 to 20°C/100 MPa).[Citation4,Citation28] Transient temperature increase due to isostatic pressure generated heat of compression (~3°C/100 MPa) is considered to be negligible as the influence is transient. As a result, the samples were exposed to combined pressure, shear, and thermal effects during UST treatment. For both 40 and 70°C UST treatments, the samples were collected from the 3rd through 5th cycle to ensure that equipment components conditioned to appropriate target thermal conditions.
Different lethal factors on the inactivation of L. brevis and B. cereus spores
The changes in populations of L. brevis and B. cereus spores treated by different processes for 0 min treatment time (time required to achieve target process conditions) are shown in . The inactivation of L. brevis and B. cereus spores under different process times, temperatures, and the different sensitivities to thermal, pressure, and shear stress are discussed in following sections.
Thermal effect on the inactivation of L. brevis and B. cereus spores
Thermal processing at 70°C and 0.1 MPa for 5 min decreased the population of L. brevis, from 10 ± 0.1 log CFU/mL to 6.4 ± 0.7 log CFU/mL. The same treatment condition caused ~1-log reduction of B. cereus spores. In the CUT (10 s) during the thermal processing, a comparable inactivation (less than 1 log) was observed for both strains (data not shown).
Pressure effect on the inactivation of L. brevis and B. cereus spores
The pressure-only effect (HPP-400 MPa-40°C-0 min) caused significant (p < 0.05) population reduction of L. brevis. The survival count of L. brevis was 8.8 ± 0.3 log CFU/mL (1.5 log reduction from untreated) during the pressure CUT () and 7.2 ± 0.1 log CFU/mL (3 log reduction from untreated) after pressure holding for 5 min. This reduction can be attributed to the pressure resistance of L. brevis.[Citation16,Citation21] For B. cereus spores, pressure-only treatment resulted in 1.8 log reduction in population during pressure CUT and 2.6-log reduction during pressure holding for 5 min. B. cereus spores showed an intermediate sensitivity to pressure since the population reductions under high pressure for 0 and 5 min (1.8 log and 2.6 log) were much higher than those under thermal-only treatment at 0 min and 5 min, respectively (0.6 log and 1.1 log).
Increasing temperature under pressure increased lethality significantly (p < .05) in L. brevis. Pressure treatment at 400 MPa and 70°C for 0 min and 5 min resulted in 1.8 ± 0.7 log CFU/mL (8 log reduction) and no detectable bacteria counts (<10 CFU/mL), respectively. With the combined effect of pressure and temperature, the viable counts of B. cereus spores reduced by 2.1 log and 3.5 log during 0 and 5 min, respectively. Considerable population reductions were reported during CUT under pressure treatment at 600 MPa and 60°C in earlier studies.[Citation23,Citation35,Citation36] The highest population decrease was observed under HPP treatment at 400 MPa at 70°C for 5 min on both strains, indicating a synergistic effect of pressure and temperature on the inactivation of microorganisms. Within the conditions of the study, we did not observe any spore germination (data not shown). Similar observations were noted in earlier research.[Citation23,Citation37–40]
Researchers have used synergetic effects combined with heat, including pressure, shear stress, or sonication, to eliminate bacteria.[Citation1,Citation6,Citation40–43] However, relative contribution of pressure, shear, and temperature and their interactions on lethal effects were not well understood.[Citation43,Citation44] Valencia-Flores et al.[Citation45] found high pressure homogenization (HPH) at 200 MPa and a temperature above 114°C could achieve 3-log reduction of B. cereus, while only 0.11-log reduction after conventional pasteurization (90°C for 90 s) was achieved. Pathanibul et al.[Citation10] compared the inactivation of P. fluorescens between a short-time thermal treatment (∼4 s, 53–87°C) and a single-pass UHPH processing (100–290 MPa) and reported that when the fluid temperature at the exit of the shear valve reached 80°C, the microbial inactivation could mainly be caused by thermal effects. However, the separate lethal effect of pressure has not been evaluated, and the cell analysis on the microstructure after different treatment conditions was not provided. The understanding of different lethal factors on the microstructure and survivability of bacterial cells is needed.
Shear effect on the inactivation of L. brevis and B. cereus spores
During UST treatment, combined effects of pressure and shear was realized at 40°C process temperature. In L. brevis, pressure, and shear in 40°C UST treatment contributed less than 2.6-log reduction (). However, the combination of pressure, shear, and temperature in 70°C UST treatment enabled sufficient inactivation by attaining ~7 log reduction of L. brevis. On the other hand, 70°C UST treatment inactivated only ~2 log population in B. cereus spores, which indicated the high pressure, shear, and heat tolerance of the spores.
The shear-only effect was estimated by the difference in the survivors between UST and HPP at similar process temperatures with 0 min holding time. The number of survivors of both strains under 40°C UST treatment at 0 min showed no significant difference. Thus, it could be inferred that shear stress as a sole factor (i.e., no pressure association) does not play a significant role on the inactivation of L. brevis and B. cereus spores. For both strains, HPP treatment facilitated relatively higher log-reduction than UST treatment under similar pressure and temperature levels. This higher reduction can be attributed to the time involved in sample loading and unloading in the batch HPP equipment and the longer pressure CUT during HPP treatment (). shows the area under the curve for the CUT portion of pressure-time and temperature-time graphs. These areas are defined as pressure and thermal dosages. The dosages during the come up time for HPP treatments were significantly higher than that for UST treatment. The lesser rate of pressurization in HPP treatment increased the area under the curve and correspondingly increased lethality, in accordance with earlier observation by Ratphitagsanti et al.[Citation46] In addition, the thermal dosage for 70°C HPP treatment was higher than 40°C HPP treatment. Thus, the higher pressure and thermal dosages during CUT of HPP treatment as compared to UST treatment helped the microbial reduction. Future experiments can attempt increasing the pressure and thermal dosages for UST treatment through suitable means to increase lethality.
Mechanical shear stress will cause cell wall collapse, cell membrane damage, and the leakage of cytoplasmic materials, resulting in cell injury or death.[Citation6,Citation7] Ghandi et al.[Citation47] used spray drying of L. lactis subsp. cremoris and observed shear-related cell damage possibly caused by cell disruption and protein denaturation.[Citation48,Citation49] When combined with high temperature, the inactivation efficiency increased significantly for L. brevis (p < .05), but not for spores (). From the results, B. cereus spores showed a higher resistance toward multiple lethal factors compared with L. brevis, showing less than 2 log reduction after UST treatment at 70°C.
Several studies have reported resistance of bacterial spores during shear treatment by HPH at process temperatures below 100°C.[Citation43,Citation50] However, the inactivation significantly increased when using a higher process temperature, indicating that temperature is a dominant parameter causing bacterial spore inactivation. Espejo et al.[Citation51] found UHPH treatment at 300 MPa and 130°C could produce a ∼5 log CFU/mL reduction of Bacillus spores. Georget et al.[Citation40] evaluated the performance of using UHPH (>300 MPa) to inactivate B. subtilis PS832 and Geobacillus stearothermophilus ATCC7953 spores, and a significant population reduction was noted when the temperature was above 145°C. Thus, it appears, both pressure and temperature provide lethal effect, while shear provided minimal or modest contribution to spore lethality under the tested conditions.[Citation40,Citation51,Citation52] Therefore, UST treatment at a higher process temperature (>100°C) may lead to relatively higher inactivation of B. cereus spores. More systematic studies should be carried out to compare the lethal effects of UST at higher temperature levels with traditional isothermal treatment and HPP for spores.
Influence of valve geometry on inactivation performance
shows the results of survivors (log CFU/mL) after treatment using different valves. For L. brevis, the shear valve geometry, temperature, and interaction of temperature and valve geometry had significant (p < .05) influence on microbial inactivation (). The number of L. brevis survivors with UST treatment at 70°C was significantly (p < .05) lower than that at 40°C. For B. cereus spores, the higher temperature (70°C) did not significantly reduce the survivor counts as compared to 40°C treatment, and this result was comparable with the previous observations described in the earlier section.
Figure 6. Influence of valve geometry on the survivors of (a) Lactobacillus brevis cells and (b) Bacillus cereus spores during ultra-shear technology (UST) treatment.
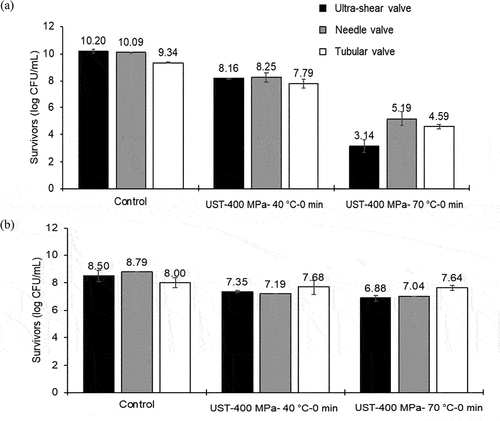
Shear valve configuration has been reported to influence cell disruption. A knife-edge valve performs better inactivation compared with other shapes.[Citation5] The efficiency of the valve type also depends on microorganism species, including its shape and cell wall structure.[Citation5,Citation53] Donsì et al.[Citation29] reported that shear treatment at 250 MPa and ~30°C could achieve 6 log CFU/mL population reduction of E. coli, while only 1 log CFU/mL reduction for L. delbrueckii, indicating a different sensitivity of microbes to shear. Further, the fluid dynamic characteristics in different valve geometries can influence the microbial inactivation, which is discussed in the following section.
Influence of fluid dynamic characteristics in different valve geometries on microbial inactivation
The variations in the energy distributed on fluid flowing through the different valve geometries, gap estimates for different valves, and resulting shear rates are presented in . Since a pressure of 400 MPa was used for all treatments, the energy density was 400 MJ/m3 for all the valve geometries. The energy distributed over fluid in the shear valve was the lowest for the ultra-shear valve due to lesser volume of fluid passing through the shear valve. The valve gap was smallest at 0.75 µm in the ultra-shear valve and largest at 8.89 µm in the tubular valve. Further, the fluid shear rate was maximum at 1.16 × 109 1/s in the ultra-shear valve (). These data highlighted the role of valve configuration and valve gap in varying the shear intensities, which in turn have variable effects on the microbial inactivation through size and structural modification of cells. The Reynolds number of the ultra-shear valve and needle valve indicated laminar flow. Similar laminar flow regime was reported on lab-scale homogenizer[Citation29] and high-pressure homogenizer.[Citation13]
Table 2. The influence of valve parameters and fluid dynamic non-dimensional numbers in different valve geometries on microbial inactivation.
The Weber number of the ultra-shear valve (1.40 × 10−5) was greater than that of other valves tested, thus indicating higher elongational stress exhibited on microbial cells in this valve. The tubular valve exhibited the lowest elongational stress among all the valves, as evident by the lowest Weber number. In the ultra-shear valve, the sudden pressure drop through the tiny valve gap creates high inertial stresses on the microbial cells. Further, the density difference causes a wall stress in the cells that eventually results in cell breakage.[Citation29]
Like Weber number, the capillary number in the ultra-shear valve was higher than the other valves. The higher capillary number indicates the dominance of shear stress in causing rupture of microbial cells. The capillary number is a function of fluid acceleration in valve gap, valve dimensions, and valve gap size.[Citation29] The smaller valve gap and resulting fluid acceleration causes high-shear stresses in the ultra-shear valve, as expressed by the capillary number. The capillary number was lowest for the needle valve, which might be due to the larger dimension of the valve.
The cavitation number indicates the formation of vapor bubbles in the fluid flowing through the shear valve when the pressure of fluid falls below its vapor pressure and the bubbles collapse, particularly, in turbulent flow.[Citation34] Cavitation could produce a shock wave that can be another major mechanism for microbial inactivation. The cavitation number of fluid for all the valves were 8.76 × 10−16, which could be attributed to the similar fluid velocity through the valve gap.[Citation29]
Since B. cereus spores were relatively more resistant to modest UST process conditions employed in the present study, inactivation data of L. brevis was compared against different valve geometries (). For L. brevis, the smaller valve gap and higher shear rate in the ultra-shear valve might be responsible for the higher reductions of 2.0 and 7.1 logs in 40 and 70°C UST treatments, respectively. The inactivation of L. brevis was lowest at 1.6 and 4.8 logs after 40 and 70°C UST treatments, respectively, in the tubular valve, which might be due to the larger valve gap and lower shear rate in this valve. This finding is in accordance with an observation by Ghandi et al.[Citation47] on survival of Lactococcus lactis subsp. cremoris during spray drying. During the atomization in spray drying with air, the authors reported high level of bacterial survival (45.19%) at the lower characteristic shear rate (229 × 103 s−1) and low survival (39.38%) at the higher characteristic shear rate (559 × 103 s−1). The relatively higher inactivation at 70°C UST treatment indicates the synergistic effect of temperature with pressure-associated shear in inactivation of L. brevis. The comparatively lesser inactivation for L. brevis in tubular valve reveals the ineffectiveness of turbulence in the inactivation of L. brevis. The higher Weber number in ultra-shear valve treatment highlights the role of higher elongational stresses on L. brevis inactivation. In addition, the capillary number of the ultra-shear valve was higher than the other valves, which points out the dominating influence of shear stress in L. brevis inactivation. Earlier researchers have reported that in high-pressure homogenizer valves with laminar flow, the cell deformation occurs due to shear forces.[Citation13,Citation29,Citation32,Citation54] Since, the cavitation number of all valves were similar, the effect of cavitation on L. brevis inactivation could not be verified in the present study. In addition to the valve geometry and fluid dynamic effects in the shear valve, other factors such as microbial concentration, pH, aw, and oxygen might play roles in microbial inactivation.[Citation30] These factors were not considered in the present analysis.
Morphology changes of L. brevis and B. cereus spores after HPP and UST treatment
SEM micrographs of L. brevis were analyzed for both 40 and 70°C temperature levels (). L. brevis are highly heat sensitive, and HPP treatment at 70°C had more influence on cell structure of L. brevis compared at 40°C HPP (). In fact, high pressure treatment compressed the bacterial cells. When the pressure was released, most of the cells went back to their original shape. In contrast, after being treated by UST (400 MPa, 70°C), the bacteria revealed clear and observable morphology change. The cell debris and broken cells under the SEM were an indication of destructive effect of the high shear force. The mechanical shear during UST treatment damaged the cells, which were characterized with several broken cells and cytoplasmic materials leaked from the cell (). The influence of pressure and shear on the morphology of bacteria can be visualized by SEM, and the inside changes caused by thermal or pressure should be further analyzed by transmission electron microscopy (TEM).
Figure 7. Representative SEM images of Lactobacillus brevis under different high pressure processing (HPP), and ultra-shear technology (UST) treatment conditions. (a) control, (b) HPP-400 MPa-40°C-0 min, (c) UST-400 MPa-40°C-0 min, (d) HPP-400 MPa-70°C-0 min, (e) UST-400 MPa-70°C-0 min. Magnification ×20,000, scale bar = 5 μm.
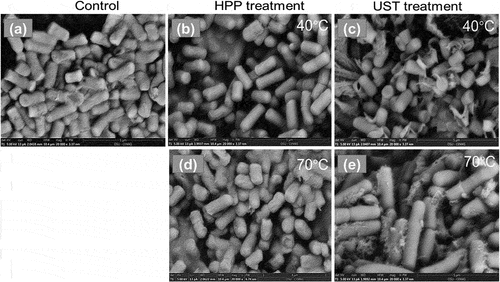
Conclusion
L. brevis cells and B. cereus spores showed different sensitivities to pressure, temperature, shear, and their combinations. The magnitude of inactivation is not only influenced by the process conditions (pressure, temperature, and treatment time), but also by the pretreatment history. For instance, at similar treatment conditions (pressure, temperature, and hold time), the HPP had longer pressure-thermal CUT than UST, which likely resulted in higher microbial inactivation. For both organisms, increases in temperature and holding time under pressure resulted in increased lethality, indicating the importance of thermal and pressure dosages in microbial inactivation. As expected, bacterial vegetative cells are more sensitive to pressure-thermal-shear treatments than spores. The magnitude of shear intensities of fluids as they pass through different valve geometries influences the microbial inactivation. Increasing the shear rate appears to increase inactivation. The higher Weber number and capillary number in the ultra-shear valve as compared to other valves indicate the contribution of elongational stress and shear stress, respectively, to achieve microbial inactivation. Thus, the study demonstrated the importance of pressure, temperature, and shear interactions and valve geometry effects during ultra-shear technology on liquids, thereby showing the potential for liquid food pasteurization or sterilization. These findings provide knowledge to food processors to design UST process conditions for beverages. In addition, the information will be help engineers to design different valve geometries and equipment parts in UST systems according to the processing requirements.
Abbreviations
UST Ultra-shear technology
HPP High-pressure processing
TP Thermal processing
CUT Come up time
Symbols
vmax maximum theoretical exit velocity (m/s)
P pressure (MPa)
ΔP total pressure drop (MPa)
Ev energy density (MJ/m3)
Ed Energy distributed on fluid volume through valve (MJ)
n number of passes
ρ fluid density (kg/m3)
σ cell wall resistance to stress tension (N/m)
α fluid acceleration (s−1)
dcellmean droplet particle size (m)
η fluid viscosity (kg/m∙s)
P2 local absolute pressure downstream of valve (MPa)
Pv vapor pressure at outlet (MPa)
uvalve average valve velocity (m/s)
Credit (CRediT) - author contributions statement
Jerish Joyner Janahar: Data curation, Formal analysis, Methodology, Software, Writing – original draft.
Jie Xu: Data curation, Formal analysis, Methodology, Software, Writing – original draft.
V.M. Balasubramaniam: Conceptualization, Funding acquisition, Methodology, Writing – review & editing, Project administration, Resources, Supervision.
A.E. Yousef: Conceptualization, Funding acquisition, Methodology, Writing – review & editing. Edmund Ting: Conceptualization, Methodology, Writing – review & editing.
Acknowledgments
Research was conducted at The Ohio State University Food Safety Engineering laboratory, Center for Clean Food Process Technology Development (u.osu.edu/foodsafetyeng/). Authors gratefully acknowledge the financial support from USDA NIFA grant 2018-67017-27914. References to commercial products or trade names are made with the understanding that no endorsement or discrimination by The Ohio State University is implied.
Disclosure statement
No potential conflict of interest was reported by the author(s).
Additional information
Funding
References
- Bevilacqua, A.; Campaniello, D.; Speranza, B.; Altieri, C.; Sinigaglia, M.; Corbo, M. R. Two Nonthermal Technologies for Food Safety and Quality—Ultrasound and High Pressure Homogenization: Effects on Microorganisms, Advances, and Possibilities: A Review. J. Food Prot. 2019, 82(12), 2049–2064. DOI: 10.4315/0362-028X.JFP-19-059.
- Georget, E.; Miller, B.; Callanan, M.; Heinz, V.; Mathys, A. (Ultra) High Pressure Homogenization for Continuous High Pressure Sterilization of Pumpable foods–a Review. Front. Nutr. 2014, 1, 15. DOI: 10.3389/fnut.2014.00015.
- Martínez-Monteagudo, S. I.; Yan, B.; Balasubramaniam, V. M. Engineering Process Characterization of high-pressure homogenization—from Laboratory to Industrial Scale. Food Eng. Rev. 2017, 9(3), 143–169. DOI: 10.1007/s12393-016-9151-5.
- Janahar, J. J.; Marciniak, A.; Balasubramaniam, V. M.; Jimenez-Flores, R.; Ting, E. Effects of Pressure, Shear, Temperature, and Their Interactions on Selected Milk Quality Attributes. J. Dairy Sci. 2021, 104(2), 1531–1547. DOI: 10.3168/jds.2020-19081.
- Diels, A. M.; Michiels, C. W. High-pressure Homogenization as a non-thermal Technique for the Inactivation of Microorganisms. Crit. Rev. Microbiol. 2006, 32(4), 201–216. DOI: 10.1080/10408410601023516.
- Tribst, A. A.; Franchi, M. A.; Cristianini, M.; De Massaguer, P. R. Inactivation of Aspergillus Niger in Mango Nectar by High Pressure Homogenization Combined with Heat Shock. J. Food Sci. 2009, 74(9), M509–M514. DOI: 10.1111/j.1750-3841.2009.01370.x.
- Vachon, J.; Kheadr, E. E.; Giasson, J.; Paquin, P.; Fliss, I. Inactivation of Foodborne Pathogens in Milk Using Dynamic High Pressure. J. Food Prot. 2002, 65(2), 345–352. DOI: 10.4315/0362-028x-65.2.345.
- Engler, C. R.; Robinson, C. W. Disruption of Candida Utilis Cells in High Pressure Flow Devices. Biotechnol. Bioeng. 1981, 23(4), 765–780. DOI: 10.1002/bit.260230408.
- Benjamin, O.; Gamrasni, D. Microbial, Nutritional, and Organoleptic Quality of Pomegranate Juice following High‐pressure Homogenization and Low‐temperature Pasteurization. J. Food. Sci. 2020, 85(3), 592–599. DOI: 10.1111/1750-3841.15032.
- Pathanibul, P.; Taylor, T. M.; Davidson, P. M.; Harte, F. Inactivation of Escherichia Coli and Listeria Innocua in Apple and Carrot Juices Using High Pressure Homogenization and Nisin. Int. J. Food Microbiol. 2009, 129(3), 316–320. DOI: 10.1016/j.ijfoodmicro.2008.12.020.
- Velázquez-Estrada, R. M.; López-Pedemonte, T. J.; Hernández-Herrero, M. M.; Roig-Sagués, A. X. Evaluation of Mycobacterium Smegmatis as Indicator of the Efficacy of High Hydrostatic Pressure and ultra-high Pressure Homogenization Treatments for pasteurization-like Purposes in Milk. J. Dairy Res. 2020, 1–9. DOI: 10.1017/s0022029919001043.
- Save, S.; Pandit, A.; Joshi, J. Microbial Cell Disruption: Role of Cavitation. Chem. Eng. J. Biochem. Eng. J. 1994, 553, B67–B72. DOI:10.1016/0923-0467(94)06062-2.
- Donsì, F.; Annunziata, M.; Ferrari, G. Microbial Inactivation by High Pressure Homogenization: Effect of the Disruption Valve Geometry. J. Food Eng. 2013, 115(3), 362–370. DOI: 10.1016/j.jfoodeng.2012.10.046.
- Floury, J.; Bellettre, J.; Legrand, J.; Desrumaux, A. Analysis of A New Type of High Pressure Homogeniser. A Study of the Flow Pattern. Chem. Eng. Sci. 2004, 59(4), 843–853. DOI: 10.1016/j.ces.2003.11.017.
- Elez-Martinez, P.; Escolà-Hernández, J.; Soliva-Fortuny, R. C.; Martín-Belloso, O. Inactivation of Lactobacillus Brevis in Orange Juice by high-intensity Pulsed Electric Fields. Food Microbiol. 2005, 22(4), 311–319. DOI: 10.1016/j.fm.2004.09.005.
- Mallidis, C.; Galiatsatou, P.; Taoukis, P.; Tassou, C. The Kinetic Evaluation of the Use of High Hydrostatic Pressure to Destroy Lactobacillus Plantarum and Lactobacillus Brevis. Int. J. Food Sci. Technol. 2003, 38(5), 579–585. DOI: 10.1046/j.1365-2621.2003.00692.x.
- Bjorkroth, K. J.; Korkeala, H. J. Lactobacillus Fructivorans Spoilage of Tomato Ketchup. J. Food Prot. 1997, 60(5), 505–509. DOI: 10.4315/0362-028x-60.5.505.
- Kable, M. E.; Srisengfa, Y.; Xue, Z.; Coates, L. C.; Marco, M. L.; Björkroth, J. Viable and Total Bacterial Populations Undergo equipment-and time-dependent Shifts during Milk Processing. Appl. Environ. Microbiol. 2019, 85(13), e00270–19. DOI: 10.1128/aem.00270-19.
- Sakamoto, K.; Konings, W. N. Beer Spoilage Bacteria and Hop Resistance. Int. J. Food Microbiol. 2003, 89(2–3), 105–124. DOI: 10.1016/s0168-1605(03)00153-3.
- Stead, D. The Effect of Chlorogenic, Gallic and Quinic Acids on the Growth of Spoilage Strains of Lactobacillus Collinoides and Lactobacillus Brevis. Lett. Appl. Microbiol. 1994, 182, 112–114. DOI:10.1111/j.1472-765x.1994.tb00819.x.
- Tribst, A. A.; Franchi, M. A.; Cristianini, M. Ultra-high Pressure Homogenization Treatment Combined with Lysozyme for Controlling Lactobacillus Brevis Contamination in Model System. Innov. Food Sci. Emerg. Technol. 2008, 9(3), 265–271. DOI: 10.1016/j.ifset.2007.07.012.
- Turner, B.; Foegeding, P.; Larick, D.; Murphy, A. Control of Bacillus Cereus Spores and Spoilage Microflora in Sous Vide Chicken Breast. J. Food Sci. 1996, 61(1), 217–219. DOI: 10.1111/j.1365-2621.1996.tb14763.x.
- Daryaei, H.; Balasubramaniam, V.; Legan, J. D. Kinetics of Bacillus Cereus Spore Inactivation in Cooked Rice by Combined pressure–heat Treatment. J. Food Prot. 2013, 76(4), 616–623. DOI: 10.4315/0362-028x.jfp-12-44.
- Goel, S. K.; Beckman, E. J. Generation of Microcellular Polymeric Foams Using Supercritical Carbon Dioxide. I: Effect of Pressure and Temperature on Nucleation. Polym. Eng. Sci. 1994, 34(14), 1137–1147. DOI: 10.1002/pen.760341407.
- Adams, D. M. Inactivation of Clostridium Perfringens Type A Spores at Ultrahigh Temperatures. Appl. Microbiol. 1973, 26, 282–287. DOI: 10.1128/am.26.3.282-287.1973.
- Chung, H.-J.; Birla, S.; Tang, J. Performance Evaluation of Aluminum Test Cell Designed for Determining the Heat Resistance of Bacterial Spores in Foods. LWT Food Sci. Technol. 2008, 41(8), 1351–1359. DOI: 10.1016/j.lwt.2007.08.024.
- Xu, J.; Janahar, J. J.; Park, H. W.; Balasubramaniam, V. M.; Yousef, A. E. Influence of Water Activity and Acidity on Bacillus Cereus Spore Inactivation during Combined High pressure-thermal Treatment. LWT. 2021, 146, 111465. DOI: 10.1016/j.lwt.2021.111465.
- Janahar, J. J.; Balasubramaniam, V. M.; Jimenez-Flores, R.; Campanella, O. H.; García-Cano, I.; Chen, D. Pressure, Shear, Thermal, and Interaction Effects on Quality Attributes of pea–dairy Protein Colloidal Dispersions. Food Hydrocoll. 2022, 107811. DOI: 10.1016/j.foodhyd.2022.107811.
- Donsì, F.; Ferrari, G.; Lenza, E.; Maresca, P. Main Factors Regulating Microbial Inactivation by high-pressure Homogenization: Operating Parameters and Scale of Operation. Chem. Eng. Sci. 2009, 64(3), 520–532. DOI: 10.1016/j.ces.2008.10.002.
- Kleinig, A. R.; Middelberg, A. P. On the Mechanism of Microbial Cell Disruption in high-pressure Homogenisation. Chem. Eng. Sci. 1998, 53(5), 891–898. DOI: 10.1016/s0009-2509(97)00414-4.
- Kelly, W. J.; Muske, K. R. Optimal Operation of high-pressure Homogenization for Intracellular Product Recovery. Bioprocess Biosyst. Eng. 2004, 27(1), 25–37. DOI: 10.1007/s00449-004-0378-9.
- Floury, J.; Legrand, J.; Desrumaux, A. Analysis of a New Type of High Pressure Homogeniser. Part B. Study of Droplet break-up and Recoalescence Phenomena. Chem. Eng. Sci. 2004, 59(6), 1285–1294. DOI: 10.1016/j.ces.2003.11.025.
- Shirgaonkar, I. Z.; Lothe, R. R.; Pandit, A. B. Comments on the Mechanism of Microbial Cell Disruption in High‐pressure and High‐speed Devices. Biotechnol. Prog. 1998, 14(4), 657–660. DOI: 10.1021/bp980052g.
- Lemmon, E. W.; Bell, I. H.; Huber, M. L.; McLinden, M. O. NIST Standard Reference Database 23: Reference Fluid Thermodynamic and Transport Properties-REFPROP, Version 10.0, National Institute of Standards and Technology; Standard Reference Data Program: Gaithersburg, 2018.
- Ahn, J.; Balasubramaniam, V. M.; Yousef, A. E. Inactivation Kinetics of Selected Aerobic and Anaerobic Bacterial Spores by pressure-assisted Thermal Processing. Int. J. Food. Microbiol. 2007, 113(3), 321–329. DOI: 10.1016/j.ijfoodmicro.2006.08.012.
- Rajan, S.; Pandrangi, S.; Balasubramaniam, V.; Yousef, A. E. Inactivation of Bacillus Stearothermophilus Spores in Egg Patties by pressure-assisted Thermal Processing. LWT Food Sci. Technol. 2006, 39(8), 844–851. DOI: 10.1016/j.lwt.2005.06.008.
- Al-Ghamdi, S.; Sonar, C. R.; Patel, J.; Albahr, Z.; Sablani, S. S. High pressure-assisted Thermal Sterilization of low-acid Fruit and Vegetable Purees: Microbial Safety, Nutrient, Quality, and Packaging Evaluation. Food Control. 2020, 114, 107233. DOI: 10.1016/j.foodcont.2020.107233.
- Brown, P.; Meyer, R.; Cardone, F.; Pocchiari, M. Ultra-high-pressure Inactivation of Prion Infectivity in Processed Meat: A Practical Method to Prevent Human Infection. Proc. Natl. Acad. Sci. 2003, 100(10), 6093–6097. DOI: 10.1073/pnas.1031826100.
- Chen, H.; Hoover, D. G. Modeling the Combined Effect of High Hydrostatic Pressure and Mild Heat on the Inactivation Kinetics of Listeria Monocytogenes Scott A in Whole Milk. Innov. Food Sci. Emerg. Technol. 2003, 4(1), 25–34. DOI: 10.1016/s1466-8564(02)00083-8.
- Georget, E.; Miller, B.; Aganovic, K.; Callanan, M.; Heinz, V.; Mathys, A. Bacterial Spore Inactivation by ultra-high Pressure Homogenization. Innov. Food Sci. Emerg. Technol. 2014, 26, 116–123. DOI: 10.1016/j.ifset.2014.08.004.
- Fan, L.; Hou, F.; Muhammad, A. I.; Ruiling, L.; Watharkar, R. B.; Guo, M.; Ding, T.; Liu, D. Synergistic Inactivation and Mechanism of Thermal and Ultrasound Treatments against Bacillus Subtilis Spores. Food Res. Int. 2019, 1094–1102. DOI: 10.1016/j.foodres.2018.09.052.
- Maresca, P.; Donsì, F.; Ferrari, G. Application of a multi-pass high-pressure Homogenization Treatment for the Pasteurization of Fruit Juices. J. Food Eng. 2011, 104(3), 364–372. DOI: 10.1016/j.jfoodeng.2010.12.030.
- Pinho, C. R.; Franchi, M. A.; Tribst, A. A.; Cristianinia, M. Effect of High Pressure Homogenization Process on Bacillus Stearothermophilus and Clostridium Sporogenes Spores in Skim Milk. Procedia Food Sci. 2011, 1, 869–873. DOI: 10.1016/j.profoo.2011.09.131.
- Chaves-López, C.; Lanciotti, R.; Serio, A.; Paparella, A.; Guerzoni, E.; Suzzi, G. Effect of High Pressure Homogenization Applied Individually or in Combination with Other Mild Physical or Chemical Stresses on Bacillus Cereus and Bacillus Subtilis Spore Viability. Food Control. 2009, 20(8), 691–695. DOI: 10.1016/j.foodcont.2008.09.001.
- Valencia Flores, D. C.; Hernández‐Herrero, M.; Guamis, B.; Ferragut, V. Comparing the Effects of Ultra‐high‐pressure Homogenization and Conventional Thermal Treatments on the Microbiological, Physical, and Chemical Quality of Almond Beverages. J. Food Sci. 2013, 78(2), E199–E205. DOI: 10.1111/1750-3841.12029.
- Ratphitagsanti, W.; Ahn, J.; Balasubramaniam, V. M.; Yousef, A. E. Influence of Pressurization Rate and Pressure Pulsing on the Inactivation of Bacillus Amyloliquefaciens Spores during pressure-assisted Thermal Processing. J. Food Prot. 2009, 72(4), 775–782. DOI: 10.4315/0362-028x-72.4.775.
- Ghandi, A.; Powell, I. B.; Howes, T.; Chen, X. D.; Adhikari, B. Effect of Shear Rate and Oxygen Stresses on the Survival of Lactococcus Lactis during the Atomization and Drying Stages of Spray Drying: A Laboratory and Pilot Scale Study. J. Food Eng. 2012, 113(2), 194–200. DOI: 10.1016/j.jfoodeng.2012.06.005.
- Goldberg, S. Mechanical/physical methods of cell disruption and tissue homogenization. Methods in Molecular Biology, vol. 424: 2D PAGE: Sample Preparation and Fractionation, Posch, A., Ed.; Totowa, NJ: Humana Press, 2008; pp 3–22. doi:10.1007/978-1-60327-064-9_1.
- Bekard, I. B.; Barnham, K. J.; White, L. R.; Dunstan, D. E. α-Helix Unfolding in Simple Shear Flow. Soft Matter. 2011, 7, 203–210. DOI: 10.1039/c0sm00692k.
- Feijoo, S.; Hayes, W.; Watson, C.; Martin, J. Effects of Microfluidizer® Technology on Bacillus Licheniformis Spores in Ice Cream Mix. J. Dairy Sci. 1997, 80(9), 2184–2187. DOI: 10.3168/jds.s0022-0302(97)76166-6.
- Espejo, G. G. A.; Hernßndez-Herrero, M.; Juan, B.; Trujillo, A. Inactivation of Bacillus Spores Inoculated in Milk by Ultra High Pressure Homogenization. Food Microbiol. 2014, 44, 204–210. DOI: 10.1016/j.fm.2014.06.010.
- Poliseli-Scopel, F. H.; Hernández-Herrero, M.; Guamis, B.; Ferragut, V. Sterilization and Aseptic Packaging of Soymilk Treated by Ultra High Pressure Homogenization. Innov. Food Sci. Emerg. Technol. 2014, 22, 81–88. DOI: 10.1016/j.ifset.2014.01.001.
- Popper, L.; Knorr, D. Applications of high-pressure Homogenization for Food Preservation: High-pressure Homogenization Can Be Used Alone or Combined with Lytic Enzyme or Chitosan to Reduce the Microbial Population and Heat Treatment Damage in Foods. Food Technol. 1990, 44(7), 84–89.
- Stang, M.; Schuchmann, H.; Schubert, H. Emulsification in High‐pressure Homogenizers. Eng. Life Sci. 2001, 1(4), 151–157. DOI: 10.1002/1618-2863(200110)1:4<151::AID-ELSC151>3.0.CO;2-D.