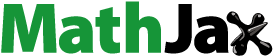
Abstract
The BABY BOOM (BBM) subfamily of the AP2/ERF transcription factor family is the main regulator of totipotency in plant cells and plays an important role in regulating cell proliferation and plant growth and development. Previously, we identified GmBBM7, a key gene of the soybean BBM gene subfamily (GmBBM family). In this study, we aimed to analyze its molecular characteristics and role in somatic embryogenesis in soybean. First, we identified 17 members of GmBBM family. Phylogenetic and collinear analyses revealed that the GmBBM family genes mainly exhibited a strong genetic relationship with the those of PvBBM family, the BBM family of common bean (Phaseolus vulgaris). Analysis of the promoters revealed that GmBBM family genes were mainly regulated by plant hormones. The expression of GmBBM7 varied in various tissues of soybean. Particularly, it was higher in the roots, immature embryos, grains and callus. Subcellular localization analysis indicated that GmBBM7 encodes a nuclear protein. Furthermore, overexpressed GmBBM7 could significantly enhance callus formation by regulating the levels of gibberellin A1, A3 and A7; abscisic acid; and salicylic acid and could promote root growth and development. In summary, GmBBM7 is an important regulatory gene for somatic embryogenesis and root elongation in soybean. GmBBM7 overexpression in soybean could increase the callus formation rate and density through hormonal pathways and could increase root growth. This study provided theoretical basis for efficient breeding of soybean via gene editing, transgenic and other techniques.
Introduction
It is believed that every plant cell has the potential of dedifferentiation. Steward et al. [Citation1] obtained completely new plants through somatic embryogenesis using isolated phloem cells of carrot roots. This indicates that highly differentiated somatic cells can also be dedifferentiated. Similar conclusions were reported by studies on Arabidopsis thaliana, Tilia amurensis and other crops [Citation2–4]. Environmental stress and hormonal induction are the important factors for somatic dedifferentiation in somatic embryogenesis [Citation5, Citation6]. Under specific environmental stress or hormonal induction, somatic cells can be directly dedifferentiated into somatic embryos, or they can be first dedifferentiated into embryogenic callus, followed by the induction of somatic embryo formation [Citation5]. The process of somatic embryogenesis is controlled by various hormones. The hormonal levels in embryo cells and somatic cells in plants are different; somatic cells have low level of abscisic acid (ABA) and high level of gibberellin, whereas embryo cells exhibit an opposite trend [Citation7–13]. Therefore, in the process of somatic embryogenesis to form callus, the level of intracellular ABA should be increased and that of gibberellin should be decreased. In addition, auxin is an important hormone that promotes somatic embryogenesis. In the medium for inducing embryogenic callus, high concentration of an auxin analog, namely, 2,4-D (2,4-dichlorophenoxyacetic acid), is usually added to promote the division of somatic cells and induce dedifferentiation to form embryogenic callus [Citation14–16]. Accumulating evidence indicates that salicylic acid (SA) can promote somatic embryogenesis [Citation17]. SA increased the endogenous H2O2 level; however, H2O2 strongly inhibited somatic embryogenesis in cucumber, which indicated that H2O2 was involved in the signaling pathway mediated by SA [Citation18].
As one of the largest transcription factor families in plants, APETALA2/ETHYLENE RESPONSE FACTOR (AP2/ERF) transcription factors are widely present in plants and play important functions in response to adversity and stress and in growth and development [Citation19]. The BABY BOOM (BBM) gene belongs to the AP2 subfamily of the AP2/ERF transcription factor family and is proven to be one of the key genes for promoting plant regeneration [Citation20, Citation21]. BBM encodes an AINTEGUMENTA-LIKE (AIL) APETALA2/ethylene-responsive element-binding factor. In Arabidopsis, the AIL gene belongs to a subfamily of 8 gene members in the AP2/ERF gene family, which includes AINTEGUMENTA (ANT), AIL1, PLETHORA1 (PLT1), PLT2, AIL6/PLT3, CHOTTO1 in addition to BBM (CHO1)/EMBRYOMAKER (EMK)/AIL5/PLT5 and PLT7 [Citation22]. BBM is expressed in the meristem of immature embryo and root tip meristem and works with other AIL gene family members to maintain the functioning of meristem [Citation23, Citation24].
BBM plays a major role in the formation of somatic and zygotic embryos, and a significantly increased transcript level of BBM can be used as a biomarker for the formation of somatic embryos in cocoa trees [Citation25]. Moreover, BBM can induce organogenesis and callus formation and is involved in regulating the growth and development of flowers and seeds and controlling seed size [Citation21]. BBM plays an important regulatory role in root development. In Arabidopsis roots, BBM is highly expressed in the root-tip stem cells and presents a gradient distribution around them; its effects vary with varying expression levels. For example, high BBM expression promotes the establishment and maintenance of stem cells; low expression promotes the mitosis of daughter cells of stem cells, and even lower expression promotes the differentiation of cells [Citation24]. Heterologous overexpression of the GmBBM gene in A. thaliana produced ectopic somatic embryos from the cotyledons, stems and hypocotyls [Citation26, Citation27]. Homologous or heterologous overexpression of BBM can improve the regeneration ability of other species (Zea mays L. and Sorghum bicolor (L.) Moench); therefore, BBM overexpression is applied to optimize the genetic transformation system [Citation26, Citation28–31]. BBM regulates the expression of YUCCA3 (YUC3) and YUCCA8 (YUC8) during somatic embryogenesis, which in turn regulates the biosynthesis of auxin; moreover, BBM regulates the ectopic expression of YUC3 and YUC8 in plants [Citation32]. In addition to the above pathways, BBM activates the LEC1-ABI3-FUS3-LEC2 network to induce somatic embryogenesis [Citation33].
Soybean, originated in China, provides high-quality plant protein to humans and is an important oil and food crop. The regeneration of soybean is limited by genotypes, which seriously hinders the application efficiency of transgenic, gene editing, and other techniques [Citation34]. The related functions of BBM and its molecular mechanism of promotion of plant regeneration are relatively well studied in model plants but not in soybean. In a previous study, we identified a key candidate gene GmBBM7 (Glyma.09G248200) responsible for soybean regeneration [Citation35]. In this study, we aimed to analyze its relationship with gene family members, molecular characteristics, and function. This study provided reference to solve the bottleneck problems of soybean regeneration and efficiency of transformation from the root limited by genotype. Furthermore, it provided insights into the molecular mechanism of soybean regeneration and provided theoretical support and technical guidance for the application of gene editing, transgenic, and other techniques in breeding of soybean.
Materials and methods
Identification of soybean GmBBM gene subfamily
Based on the results of a previous study [Citation35], using the full-length CDS sequence of GmBBM (Glyma.09G248200) gene as a reference, the BLASTP was performed using the Phytozome database (https://phytozome-next.jgi.doe.gov/), and the members of the GmBBM gene subfamily were screened with reference to the AP2 domain (PF00847). Information on the genetic characteristics of GmBBM, including coding sequence length, chromosome location and protein length, was obtained using the Phytozome database (https://phytozome-next.jgi.doe.gov/). The isoelectric point and molecular mass were determined using the ExPASy server (http://expasy.org/). Transit peptides and subcellular localization were predicted using TargetP 2.0 (https://services.healthtech.dtu.dk/service.php?TargetP-2.0) and Cello 2.56 (http://www.csbio.sjtu.edu.cn/bioinf/plant-multi/) [Citation36].
Evolution, gene structure and homology analysis of GmBBM
The full-length protein and genome sequences of soybean (Glycine max Wm82.a2.v1) GmBBM, maize (Zea mays RefGen_V4) ZmBBM, rice (Oryza sativa v7.0) OsBBM, Arabidopsis (A. thaliana TAIR10) AtBBM and common bean (Phaseolus vulgaris v2.1) PvBBM genes were obtained from the Phytozome database. The phylogenetic tree was constructed using the maximum likelihood method in MEGA software (https://www.megasoftware.net/) [Citation37]. Gene structure analysis was performed using GSDS 2.0 (http://gsds.gao-lab.org/) [Citation38]. Collinearity analysis was performed using the RCircos code [Citation39]. The BBM gene IDs and basic information used in this study are given in Supplemental Table S1.
GmBBM promoter analysis
To study the key cis-acting elements in the promoters of GmBBM genes, the upstream 1.5-kb sequences of these genes were obtained from the Phytozome database. Cis-acting regulatory DNA elements were predicted from the PlantCARE database (http://bioinformatics.psb.ugent.be/webtools/plantcare/html/) and visualized using IBS 2.0 (https://ibs.renlab.org/#/home) [Citation40].
Analysis of expression patterns of GmBBM gene subfamily members
The data of expression of GmBBM gene subfamily members in soybean flowers, leaves, nodules, pod, root, root hairs, seed, shoot apical meristem, and stem were downloaded from the Phytozome database (Glycine max Wm82.a2.v1) [Citation41]. A heat map of the expression patterns of GmBBM gene subfamily members was plotted using TBtools (https://github.com/CJ-Chen/TBtools).
Gene cloning and vector construction
Soybean variety SN14 was maintained in the Soybean germplasm resources collection in the Soybean Genetic and Improvement Lab (Northeast Agricultural University, Harbin, China). Immature embryos with a diameter of 3–5 mm were used. Explant material was sterilized in 20% sodium hypochlorite solution for 20 min. The adaxial ends of immature embryos were inoculated in D40 medium. D40 medium was prepared as follows: Murashige & Skoog Basal Salt Mixture (PhytoTech LABs, M524) 4.33 g, Sucrose 30 g, 2,4-D 40 mg and 3 g Agar were added to each litre of D40 medium. Calli cultured for 40 days were used as experimental materials.
The RNA of the sample was extracted using the Trizol RNA extraction Kit (Invitrogen, 15596026), HiScript III 1st Strand cDNA Synthesis Kit (Vazyme, R312-01) was used to reverse transcribe RNA into cDNA by PCR instrument (Applied Biosystems, 2720 Thermal Cycler). XbaI and BamHI cleavage sites were selected for Fu28 vector, and GmBBM7 gene (Glyma.09G248200) cloning primers were designed according to the above cleavage sites. The sequence of gene cloning primers is shown in . Using the reverse-transcribed cDNA as a template, the GmBBM7 gene was cloned using 2 × KeyPo Master Mix (Vazyme, PK511-01). GmBBM7 was ligated to the Fu28 vector by homologous cloning using ClonExpress® II One Step Cloning Kit (Vazyme, C115-01). Using the constructed Fu28-GmBBM7 vector, Gateway recombinant technology was used to construct pSoy1-GmBBM7 overexpression vector for subcellular localization and soybean genetic transformation.
Table 1. Primers used in this study.
RNA extraction and tissue-specific analysis of GmBBM7
Soybean variety SN14 was used for tissue specificity analysis of GmBBM7 gene. Roots, stems, leaves, immature embryo, callus induced by immature embryo and seeds at mature stage were selected as experimental materials. Three biological replicates were used for each sample. Samples were frozen in liquid nitrogen immediately after sampling and stored at −80 °C. The total RNA was extracted from various tissues of SN14 using TRIzol reagent. Reverse transcription was performed using HiScript III 1st Strand cDNA Synthesis Kit (Vazyme, R312-01). The transcript levels of GmBBM7 in various tissues were analyzed using qRT-PCR with ChamQTM Universal SYBR®qPCR Master Mix Kit (Vazyme, Q711-02) as per the manufacturer’s instructions on a Roche Light Cycler 480 (Roche, United States) instrument. Actin4 (GenBank accession no. AF049106; Phytozome database, Glycine max Wm82.a2.v1, Glyma.12G063400), an endogenous gene of soybean, was used as the qRT-PCR control. The primers of GmBBM7 and Actin4 are given in . The experiment was repeated 3 times for each tissue, and the results were analyzed using the 2−ΔΔCt method [Citation42].
Subcellular localization
To study the subcellular localization of GmBBM7, chemically competent Agrobacterium cells (Agrobacterium tumefaciens EHA105; WEIDI, AC1010; 1 mL) containing the overexpression vector pSoy1-GmBBM7 were inoculated into YEP medium containing corresponding antibiotics (Spectinomycin, 75 μg/mL) and cultured at 28 °C and 220 rpm until the OD600 of the bacterial suspension was approximately 1. pSoy1-GmBBM7 contains a green fluorescence protein (GFP) tag, which makes the GmBBM7 protein emit green fluorescence under a confocal microscope. The bacterial suspension was centrifuged at 4800 g for 10 min, and the cell pellet was resuspended with resuspension solution (200 mmol L−1 MES, 200 μmol L−1 acetosyringone, 150 mmol L−1 MgCl2, ddH2O) to obtain an OD600 of 0.6–0.8. A plasmid expressing mRFP: AHL22 protein was used as a nuclear localization marker [Citation43]. Equal amounts of bacterial suspensions of Agrobacterium tumefaciens EHA105 containing pSoy1-GmBBM7 and Agrobacterium tumefaciens EHA105 containing mRFP: AHL22 were mixed, then placed at room temperature for 3 h, and then injected into a fourth fully unfolded leaf of Nicotiana benthamiana [Citation44, Citation45]. The leaves were protected from light for 12 h and observed under a confocal microscope (FV3000, OLYMPUS) after 3 days. Detection was performed on GFP channel, YFP channel, Chlorophyl channel, Brignt Field channel and Merged channel.
Construction and phenotypic identification of transgenic hairy roots overexpressing GmBBM7
Hairy root transformation was used as one of the best systems to study gene function and expression [Citation46–53]. For this purpose, chemically competent Agrobacterium cells (Agrobacterium rhizogenes K599; WEIDI, AC1080; 1 mL) containing the overexpression vector pSoy1-GmBBM7 were inoculated into TY medium (TRYPTONE 5.0 g/L, YEAST EXTRACT 3.0 g/L, CaCl2.6H2O 1.3 g/L, pH = 7.0) containing corresponding antibiotics (Spectinomycin, 75 μg/mL) and cultured at 28 °C and 220 rpm until the OD600 of the bacterial suspension was approximately 0.7, and soybean hairy roots were transformed [Citation54]. The hairy roots overexpressing GmBBM7 were detected using a portable excitation light source and bar strips. The initial weight of such hairy roots was noted using an analytical balance (ten thousandth). At the same time, qRT-PCR was performed to assess the expression level of GmBBM7 in the hairy roots. The transgenic hairy roots were cultured in hairy root induction medium at 26 °C in dark. From the 2nd day when the explants started exhibiting hairy roots till the 8th day, the length of the tap root of hairy roots was measured and the number of lateral roots was counted every alternate day. Therefore, in total, five measurements were taken. On the 8th day, the hairy roots were weighed to obtain their final weight. The average daily growth rate of hairy roots was calculated according to the following formula:
[Citation55] where a and b are the initial and final weights of hairy roots (g), respectively; n corresponds to the days of culture (d); RGR is the daily average growth rate of hairy roots (g·g−1 d−1).
The hairy roots overexpressing GmBBM7 with good growth were selected, and 2 intact roots were placed on the callus induction medium to induce callus. Each root was numbered and cultivated in light culture at 26 °C for 18 h [Citation56]. From the 5th day, the number of the hairy roots that grew callus from the site other than the incision was recorded. The recording was stopped when no new hairy roots formed callus for 5 consecutive days. The callus was subcultured using D40 medium to determine the callus growth rate. When the callus was cultured for 15 days, the total weight of callus was used as the final weight of one subculture cycle, and three consecutive cycles were conducted. The callus growth rate was calculated as follows:
[Citation55]
[Citation55]
Detection of phytohormones in transgenic soybean callus overexpressing GmBBM7
Overall, two groups of tissue samples (Wild-type hairy root and hairy root overexpressing the GmBBM7 gene) were tested, each with three biological replicates. At the same time, all samples to be measured were mixed in equal quantities to prepare QC (quality control) samples. In total, 50 mg of each tissue sample was ground in liquid nitrogen. To the tissue powder, 200 μL of pre-cooled ultrapure water was added. After mixing the samples, 800 μL methanol: acetonitrile (1:1, v/v) was added to the samples, and then the samples were vortex mixed. The mixture was sonicated in an ice bath for 60 min using an ultrasonic homogenizer (JY92-IIDN, SCIENTZ, China), incubated at −20 °C for 1 h to precipitate the proteins, and centrifuged at 14,000 g at 4 °C for 20 min. The supernatant was vacuum-dried. The precipitates were redissolved in 200 μL of 50% acetonitrile solution and centrifuged at 20,000 g for 10 min. The supernatant was collected for mass spectrometry (BIOPROFILE, China). Chromatographic peak areas and retention times were calculated using MultiQuant software. Metabolites were identified based on the retention times using phytohormone standards [Citation57]. The abbreviations of the tested hormones are given in Supplementary Table S2.
Statistical analysis
SPSS Statistics 24 software was used for statistical analysis. Differences between two groups were analyzed by student’s t-test. GraphPad Prism 8, Microsoft Excel 2016 and PowerPoint 2016 were used to generate graphs. Differences were considered statistically significant at the p < 0.05 level.
Results
Identification and classification of soybean GmBBM gene subfamily
Considering the full-length protein sequence and structural domain of the soybean GmBBM gene (Glyma.09G248200) as the reference [Citation35], the full-length coding sequence of GmBBM1–17 was 1320–2124 bp, encoding 439–707 amino acids. The isoelectric points and relative molecular masses of the protein products of 17 GmBBM genes were 6–8.61 and 48.38–77.34 kDa, respectively (). Subcellular localization prediction analysis suggested that GmBBM family members were mainly localized in the nucleus and cytoplasm. To study the classification and evolution of GmBBM, a phylogenetic tree was constructed based on the BBM gene subfamily members of G. max, A. thaliana, Z. mays, O. sativa and P. vulgaris. The BBM genes of various plants can be divided into three clusters (). GmBBM7 (Glyma.09G248200), the target gene of this study, was located in cluster I; ZmBBM (Zm00001d042492) and AtBBM (AT5G17430), which are reported to regulate the embryonic development of maize and Arabidopsis, were located in the same cluster [Citation20, Citation33]. This indicated that the members of BBM gene families located in this cluster may play important functions in plant growth and development. The phylogenetic tree revealed that despite different clusters, the soybean BBM gene and common bean BBM gene shared closer genetic relationship.
Figure 1. Phylogenetic analyses of soybean BBM gene subfamily members and prediction of BBM protein structure. (A) Phylogenetic analysis of Glycine max, Arabidopsis thaliana, Zea mays, Oryza sativa and Phaseolus vulgaris (phylogenetic tree analysis by maximum likelihood method using MEGA software); (B) Domain analysis of soybean and Arabidopsis BBM proteins, soybean and Arabidopsis BBM protein length and AP2 domain information were derived from the Phytozome database and plotted using IBS 2.0 and PowerPoint 2016. The predicted AP2 domain (PF00847) is depicted as a teal square.
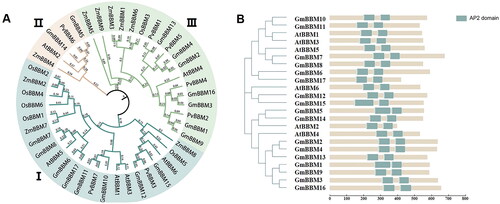
Table 2. Basic information of soybean BBM gene family.
The domain structure of GmBBM1-17 protein was analyzed. GmBBM had protein domains similar to those reported in the BBM proteins of maize and Arabidopsis, both of which had two AP2 domains (PF00847). The protein domains of soybean and Arabidopsis BBM gene families are highly conserved ().
Collinear relationship and structure analysis of GmBBM gene subfamily members
To understand the potential functions of GmBBM genes, we performed a synteny analysis of members of the BBM gene subfamily in G. max, A. thaliana, Z. mays, O. sativa and P. vulgaris. In soybean, 17 GmBBM genes are distributed on 15 chromosomes (; Supplemental Table S1). In addition, 69 pairs of genes homologous with GmBBM were observed in these five species. Among them, 25, 2, 24, 6 and 1 homologous gene pairs were reported in soybean–soybean, soybean–maize, soybean–common bean, soybean–Arabidopsis and soybean–rice, respectively.
Figure 2. Gene collinearity and exon–intron structure analysis of members of the GmBBM gene subfamily. (A) Collinearity analysis of BBM genes of G. max, A. thaliana, Z. mays, O. sativa, and P. vulgaris. Collinearity analysis between different species was performed using the R circos code, and the chromosomes of the above species are depicted in a circular form for ease of presentation. Coloured curves indicate collinear regions of BBM; (B) Exon–intron schematic diagram of AtBBM and GmBBM. Exons and introns of BBM genes in soybean and Arabidopsis were obtained from the Phytozome database, and the gene structure was analyzed using the online tool GSDS 2.0. Untranslated region (UTR) sequences are represented by green squares. Exons and introns are indicated as pink boxes and dashed lines, respectively.
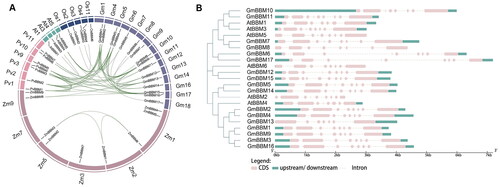
To further understand the structural diversity of GmBBM genes, we compared the exon–intron positioning and size of GmBBM and AtBBM genes. BBM genes belonging to the same subgroup have similar exon and intron structures, especially in the number and relative positions of exons ().
Regulatory elements in the promoters of GmBBM gene subfamily members
To identify cis-elements involved in the transcriptional regulation of GmBBM genes, the upstream 1.5-kb promoter region of each GmBBM gene was analyzed. Except GmBBM13 and GmBBM17, all other members of the GmBBM gene subfamily have hormone-responsive elements (). Members of the GmBBM gene subfamily mainly respond to hormones such as ABRE (Abscisic acid-responsive element), P-box (Gibberellin-responsive element), TGA (Auxin-responsive element) and CGTCA (MeJA-responsive element). In addition to their regulation by hormones, GmBBM4, GmBBM9, GmBBM10, GmBBM11 and GmBBM14 have CAT (Meristem expression element). In addition, GmBBM2, GmBBM15 and GmBBM16 have GCN4 (Endosperm expression elements). In the candidate gene GmBBM7, no meristem expression response elements and endosperm expression elements are present; however, it has a large number of ABA response elements.
Figure 3. Prediction of cis-elements in the 1.5-kb promoter region upstream from the start codon of GmBBMs. The upstream 1.5-kb sequences of GmBBMs were obtained from the Phytozome database, the key cis-elements were predicted using PlantCARE database, and the visualization was done using IBS 2.0. The relative positions of cis-elements in each GmBBM gene are marked by boxes of different colours.
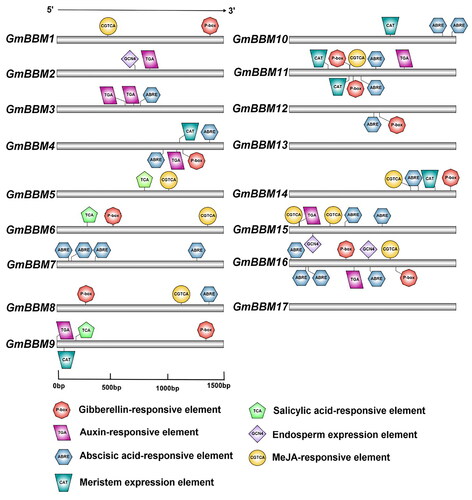
Expression patterns of GmBBM gene subfamily members
Using high-throughput sequencing data from the Phytozome database, we evaluated the expression patterns of GmBBM gene subfamily members in various tissues, including flowers, leaves, nodules, pod, root, hairy roots, seed, shoot apical meristem and stem (). GmBBM gene subfamily members could be divided into 4 clusters according to their expression patterns. GmBBM7, 10 and 11 were highly expressed in the nodules, roots, hairy roots and seeds. The expression of GmBBM1, 8 and 9 did not follow any particular pattern in different tissues. GmBBM5, 6, 14 and 17 were mainly highly expressed in the seeds, whereas GmBBM2, 3, 4, 12, 13, 15 and 16 were highly expressed in the stem and shoot apical meristem.
Figure 4. Analysis of the expression patterns of GmBBM gene subfamily members. The expression data of GmBBM gene subfamily in different tissues were obtained from the Phytozome database. TBtools software was used to map the expression patterns of GmBBM gene subfamily members. The abscissa represents the flowers, leaves, nodules, pod, root, roots hairs, seed, shoot apical meristem, and stems. The ordinate represents the members of the GmBBM gene subfamily. The color scale represents the expression value of log2.
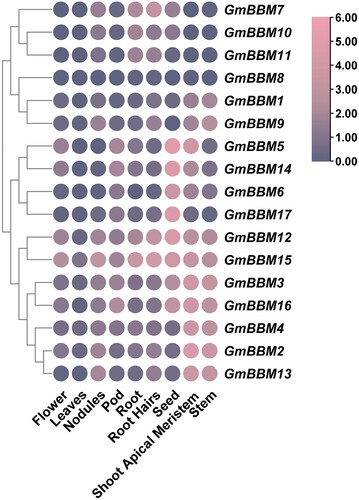
Molecular characterization analysis of GmBBM7
GmBBM7 was expressed in various parts of soybean (). The expression levels were higher in the roots and immature embryos, followed by the grains and callus. As expected, the expression levels in the stems and leaves were lower. GmBBM7 was highly expressed at the growth and differentiation sites. This indicated that GmBBM7 played an important role in differentiation, embryonic growth and callus formation in soybean. In addition, the expression level of GmBBM7 was the highest in the roots, which indicated to a certain extent that this gene may be involved in the differentiation of root primordium and played a role in root growth and development.
Figure 5. Molecular characterization analysis of GmBBM7. (A) Tissue-specific expression analysis of GmBBM7. The abscissa indicates various tissues (root, stem, leaf, callus, embryo and grain) of soybean variety SN14, and the ordinate represents the expression level relative to the housekeeping gene Actin4; (B) Subcellular localization analysis of GmBBM7. Confocal microscopy showing GmBBM7-GFP fluorescence (1), nuclear protein marker-AHL22 (2), chloroplast autofluorescence (3), corresponding bright field (4), and accordingly merged pictures (5).
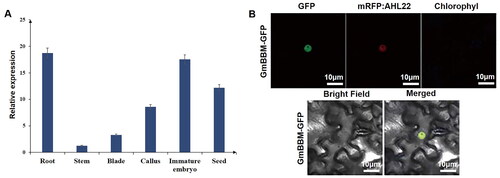
The expression of GmBBM and GFP-tag fusion protein was observed under a confocal microscope. The co-localization with a nuclear marker (AHL22) was studied, which indicated that GmBBM7 was localized in the nucleus. Therefore, GmBBM7 encodes a nuclear protein, which is basically consistent with the bioinformatics predictions ().
Effect of GmBBM7 on the regeneration ability of soybean
In a previous study, we reported that GmBBM7 plays a role in soybean callus formation [Citation35]. However, the exact role and underlying mechanism are still unclear. To verify whether the overexpression of GmBBM7 (OE) affects soybean regeneration, transgenic hairy roots overexpressing GmBBM7 were prepared (; Supplemental Figure S1). The expression level of GmBBM7 in overexpressing transgenic hairy roots was significantly higher than that in wild-type (WT) hairy roots (). Considering the same genetic background, all phenotypic differences between transgenic and WT hairy roots were due to the overexpression of GmBBM7.
Figure 6. Effects of GmBBM7 overexpression on the growth and development of soybean callus and root. (A) The left panels are the roots and calli of hairy roots overexpressing GmBBM7 (OE), and the right panels are the roots and calli of wild-type (WT) hairy roots; (B) the left panel shows hairy roots overexpressing GmBBM7 and the right panel shows WT hairy roots, both cultured for 8 days from the second day of rooting; (C) the relative expression levels of GmBBM7 in OE and WT hairy roots (hairy roots cultured to 8d from OE and WT materials); (D) callus density in OE and WT hairy roots (Calli were cultured with OE and WT materials for 45 days). the ordinate is callus density (g cm−3); (E) Callus formation rate in OE and WT hairy roots (Calli were cultured with OE and WT materials for 45 days). the ordinate is the callus formation rate (%); (F) growth rate of OE and WT hairy roots (hairy roots cultured to 8d from OE and WT materials). the ordinate is the growth rate of hairy roots (g·g−1 d−1); (G) the growth rate of the tap root of OE and WT hairy roots. The abscissa is the number of days (0–2, 2–4, 4–6, and 6–8 days). the vertical axis is the growth rate of tap root of hairy roots (cm cm−1 d−1) (**p < 0.01).
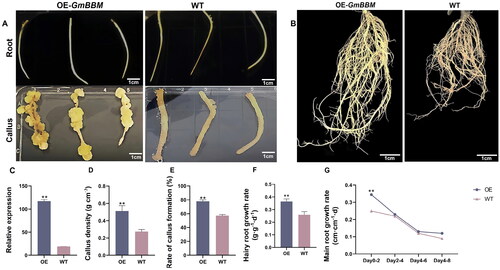
First, the callus-associated phenotypes of GmBBM7-overexpressing transgenic and WT hairy roots were identified. The callus density after continuous culture for 15 days is shown in . The callus density of transgenic hairy roots was significantly higher (approximately twice) than that of WT hairy roots (p = 0.003). The callus formation rate of the transgenic hairy roots was 77.84%, which was considerably higher than that of WT (57.20%; ). These results indicated that the overexpression of GmBBM7 could significantly improve the callus formation rate and callus quality.
Further, we investigated the effect of GmBBM7 overexpression on the root growth and development. GmBBM7 overexpression could significantly promote the growth of hairy roots (), exhibiting an increase by 1.4 times compared with WT. The taproot elongation of hairy roots is shown in . Growth rate of the tap root of transgenic and WT samples decreased with time. During days 0–2, when the tap root grew most vigorously, the growth rate of the tap root of the transgenic samples was significantly higher than that of WT. The overexpression of GmBBM7 could effectively promote the growth and development of soybean callus and root.
Effect of GmBBM7 on the phytohormonal levels in hairy root callus
Studies have reported that phytohormonal levels are an important factor in the formation and growth of callus [Citation58–62]. To explore whether GmBBM7 is involved in the formation and growth of soybean callus by affecting phytohormonal levels, we assessed the changes in the phytohormonal levels in the callus of GmBBM7-overexpressing transgenic hairy roots and WT hairy roots. A total of 18 phytohormones were detected in the tested samples. The standard curve and the quantitative values of various hormones are shown in Supplemental Figure S2 and Supplemental Table S2, respectively. Significant differences were observed in the levels of phytohormones such as 2iP Riboside (IPR), Abscisic Acid (ABA), Dihydrozeatin (DZ), gibberellin A1 (GA1), gibberellin A3 (GA3), gibberellin A7 (GA7), Indole-3-butyric Acid (IBA), and Salicylic acid (SA) between WT and GmBBM7-overexpressing transgenic hairy roots (). The levels of GA1, GA3 and GA7 in hairy roots overexpressing GmBBM7 were significantly lower than those in WT hairy roots. Since GmBBM7 overexpression can promote the formation and growth of callus, the reduction in endogenous gibberellins appears to be beneficial for callus formation. The levels of ABA and SA in the hairy roots overexpressing GmBBM7 were significantly increased. This indicated that GmBBM7 overexpression affected the metabolism of ABA and SA, thereby promoting the growth of callus and hairy roots.
Figure 7. Determination of hormonal levels in WT and GmBBM7-overexpressing transgenic hairy root calli (Calli were cultured with OE and WT materials for 45 days). (A) One-way analysis of variance of OE and WT calli. horizontal and vertical scales are the names of the tested hormones. vertical axis indicates the hormonal content (ng g−1). IPR (Isopentenyladenine riboside), ABA (Abscisic acid), BR (brassinolide), DZ (Dihydrozeatin), GA1 (gibberellin A1), GA3 (gibberellin A3), GA7 (gibberellin A7), IAA (Indole-3-acetic acid), IBA (3-Indolebutyric acid), Ica (Indole-3-carboxaldehyde), IP (N6-Isopentenyladenine), JA-ILE (Jasmonoyl-L-Isoleucine), MeIAA (methyl indole-3-acetate), Melatonin (Melatonin), MESA (methylsalicylate), SA (Salicylic acid), TZ (trans-Zeatin), ZR (trans-Zeatin-riboside); (B) Hierarchical clustering analysis of the heat map of hormonal content corresponding to ; the first three columns are the 3 repetitions of the OE test sample, and the last three columns are the 3 repetitions of the WT test sample, each box in the heat map represents the relative abundance of the corresponding hormone in the corresponding sample, red represents the increase of the relative hormone content, blue represents the decrease of the relative hormone content, and the depth of the color represents the magnitude of the difference. (*p < 0.05, **p < 0.01), the hierarchical clustering analysis was plotted using TBtools software.
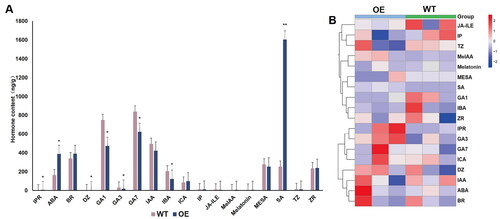
Discussion
Soybean is an important economic crop and one of the main sources of protein and oil for humans [Citation63]. With the publication of reference genomes such as Williams82, Enrei and Zhonghuang13, it is estimated that soybean has a total of 46,430 protein-coding genes (70% more than Arabidopsis) [Citation64–66]. Soybean is an ancient tetraploid plant, and nearly 75% of its genes exist in multiple copies. It is important to identify the key genes for soybean regeneration, verify the functions of related genes, and understand the mechanism of soybean cell regeneration [Citation67]. The transcription factor BBM is a master regulator of plant embryogenesis. It belongs to the AP2 subfamily of the AP2/ERF transcription factor family and has multiple functions in cell proliferation, growth and development [Citation26]. BBM encodes the AIL protein and directly regulates all LAFI genes, which activate auxin synthesis and transport to regulate somatic embryogenesis [Citation33]. In addition, BBM is involved in regulating the expression of YUC3 and YUC8, thereby regulating the biosynthesis of auxin [Citation32]. The function of BBM is studied in detail in Arabidopsis and other crops but not in soybean.
In this study, 17 members of the GmBBM gene subfamily (GmBBM1–17) were identified from soybean genome (). Similar to BBM in other crops, in GmBBM, two AP2 domains (PF00847) are connected in a series, the synteny analysis of BBM subfamily members in different species showed that there were more homologous genes in soybean - soybean and soybean - common bean. Moreover, BBM genes belonging to the same subfamily have similar exon and intron structures. ( and ). Combined with the analysis of promoter cis-acting elements, it can be observed that except for GmBBM13 and GmBBM17, other GmBBM family members contain a large number of hormone-responsive induction elements (). This indicated that the members of the GmBBM gene subfamily are mainly regulated by hormones. The expression patterns of GmBBM subfamily members in different tissues in Phytozome database were analyzed. According to the expression patterns, the GmBBM subfamily members can be divided into 4 clusters. Similar to the results of tissue-specific analysis of GmBBM7 in this study, GmBBM7 was expressed higher in roots, immature embryos, callus and other parts where cell division and proliferation are vigorous ( and ). In addition, GmBBM7 encodes a nuclear protein, suggesting that the protein it encodes may perform corresponding functions in the nucleus (), which is consistent with previous studies [Citation23, Citation24].
In summary, we can predict that during somatic embryogenesis of soybean, GmBBM7 may first respond to external hormonal signals, thereby inducing somatic embryogenesis [Citation20]. When GmBBM7 was overexpressed, the callus formation rate and density increased significantly (), indicating that GmBBM7 positively regulates somatic embryogenesis and accelerates the proliferation of callus cells in soybean. In addition, GmBBM7 overexpression could significantly increase the growth rate of tap root and hairy roots (). This is consistent with BBM gene functions reported in other crops [Citation24, Citation26].
In the process of somatic embryogenesis, various stages can be effectively regulated through the combined use of various exogenous hormones [Citation7]. To understand this in detail, the hormonal levels were determined in the hairy roots overexpressing GmBBM7. The levels of GA1, GA3 and GA7 in hairy roots overexpressing GmBBM7 were significantly lower than those in WT hairy roots (). Studies have shown that RGL3 (RGLLIKE-3) is a negative regulator of gibberellin content in plants, and RGL3 interacts with ABI3. In addition, BBM regulates the expression level of ABI3 gene, so BBM may indirectly regulate gibberellin content in plants. [Citation68]. Therefore, it was suggested that GmBBM7 participates in the gibberellin-regulated pathway of somatic embryogenesis in soybean. In addition to hormones, adversity stress signals are important factors that affect somatic embryogenesis. During the induction of somatic embryos, mechanical damage and ion penetration affect somatic embryogenesis [Citation69–71]. Interestingly, the levels of SA and ABA were significantly increased in the GmBBM7-overexpressing transgenic hairy roots. In the process of somatic embryogenesis, SA is involved in H2O2 accumulation, and ABA is one of the hormones responding to adversity stress [Citation17, Citation72]. This indicated that GmBBM7 is also likely involved in adversity stress response during somatic embryogenesis.
Conclusions
In this study, 17 members of the GmBBM gene subfamily containing two AP2 domains were identified in the soybean genome. Combined phylogenetic and collinear analyses revealed that the GmBBM has the highest homology with common bean PvBBM gene. The members of GmBBM gene subfamily are mainly localized in the nucleus and cytoplasm. The analysis of the cis-acting elements in the upstream 1.5-kb sequence of the promoter revealed that the expression of the members of the GmBBM gene subfamily was mainly regulated by phytohormones. GmBBM7, the target gene in this study, encodes a nuclear protein; its expression greatly varied in various tissues of soybean. GmBBM7 was highly expressed in the roots, callus, embryos and seeds. This indicated that GmBBM7 mainly plays important roles in the parts of active growth and differentiation in soybean. When GmBBM7 was overexpressed, the callus density, callus formation rate, hairy root growth rate, and tap root growth rate were significantly increased. Moreover, the contents of endogenous plant hormones GA1, GA3, and GA7 significantly decreased and those of ABA and SA significantly increased. In summary, GmBBM7 positively regulates the growth and development of soybean callus and root during somatic embryogenesis by regulating the levels of endogenous plant hormones.
Authors’ contributions
Xiaoxia Wu, Qingshan Chen and Sinan Li designed the experiments; Runnan Zhou, Yujing Zhao, Peng Cheng and Sinan Li carried out the experiments; Binshuo Zhang, Zhen Liu, Sihui Wang and Haibo Li analyzed the experimental results. Ying Zhao analyzed the sequence data. Sinan Li provided experimental consumables and related equipment support. Runnan Zhou, Sinan Li and Yujing Zhao wrote the manuscript. Runnan Zhou, Peng Cheng and Yujing Zhao revised the manuscript. All authors have read and agreed to the published version of the manuscript.
Supplemental Material
Download PDF (3 MB)Acknowledgements
The authors express gratitude to BIOPROFILE (Shanghai, China) for providing hormone measurement services for this study. The authors thank Dr. Yongfu Fu ‘s team (MOA Key Lab of Soybean Biology, National Key Facility of Crop Gene Resource and Genetic Improvement, Institute of Crop Sciences, Chinese Academy of Agricultural Sciences, Beijing 100081, China) for providing nuclear marker (mRFP: ALH22) and Nicotiana benthamiana for this study.
Disclosure statement
No potential conflict of interest was reported by the authors.
Data availability statement
All data that support the findings reported in this study are available from the corresponding author upon reasonable request.
Additional information
Funding
References
- Steward FC, Mapes MO, Mears K. Growth and organized development of cultured cells. II. Organization in cultures grown from freely suspended cells. Am J Bot. 1958;45(10):1–14. doi: 10.2307/2439728.
- Ikeuchi M, Iwase A, Rymen B, et al. PRC2 represses dedifferentiation of mature somatic cells in Arabidopsis. Nat Plants. 2015;1:15089. doi: 10.1038/nplants.2015.89.
- Kim TD, Lee BS, Kim TS, et al. Developmental plasticity of glandular trichomes into somatic embryogenesis in Tilia amurensis. Ann Bot. 2007;100(2):177–183. doi: 10.1093/aob/mcm094.
- Yuan G, Shui GL, Xiao FF, et al. Application of somatic embryogenesis in woody plants. Front Plant Sci. 2016;7:938. doi: 10.3389/fpls.2016.00938.
- Fehér A. Somatic embryogenesis—stress-induced remodeling of plant cell fate. Biochim Biophys Acta. 2015;1849(4):385–402. doi: 10.1016/j.bbagrm.2014.07.005.
- Ikeda-Iwai M, Umehara M, Satoh S, et al. Stress-induced somatic embryogenesis in vegetative tissues of Arabidopsis thaliana. Plant J. 2003;34(1):107–114. doi: 10.1046/j.1365-313X.2003.01702.x.
- Hays D, Mandel R, Pharis R. Hormones in zygotic and microspore embryos of Brassica napus. Plant Growth Regul. 2001;35(1):47–58. doi: 10.1023/A:1013831116996.
- White CN, Proebsting WM, Hedden P, et al. Gibberellins and seed development in maize. I. Evidence that gib-berellin/abscisic acid balance governs germination versus maturation pathways. Plant Physiol. 2000;122(4):1081–1088. doi: 10.1104/pp.122.4.1081.
- Ogawa M, Hanada A, Yamauchi Y, et al. Gibberellin biosynthesis and response during Arabidopsis seed germination. Plant Cell. 2003;15(7):1591–1604. doi: 10.1105/tpc.011650.
- Castro RD, Hilhorst HWM. Hormonal control of seed development in GA- and ABA-deficient tomato (Lycopersicon esculentum mill. cv. Moneymaker) mutants. Plant Sci. 2006;170(3):462–470. doi: 10.1016/j.plantsci.2005.09.014.
- Hu J, Mitchum MG, Barnaby N, et al. Potential sites of bioactive gibberellin production during reproductive growth in Arabidopsis. Plant Cell. 2008;20(2):320–336. doi: 10.1105/tpc.107.057752.
- Vahdati K, Bayat S, Ebrahimzadeh H, et al. Effect of exogenous ABA on somatic embryo maturation and germination in Persian walnut (Juglans regia L.). Plant Cell Tiss Organ Cult. 2008;93(2):163–171. doi: 10.1007/s11240-008-9355-3.
- Braybrook SA, Harada JJ. LECs go crazy in embryo development. Trends Plant Sci. 2008;13(12):624–630. doi: 10.1016/j.tplants.2008.09.008.
- Gaj MD. Factors influencing somatic embryogenesis induction and plant regeneration with particular reference to Arabidopsis thaliana (L.) heynh. Plant Growth Regul. 2004;43(1):27–47. doi: 10.1023/B:GROW.0000038275.29262.fb
- Jiménez VM. Involvement of plant hormones and plant growth regulators on in vitro somatic embryogenesis. Plant Growth Regul. 2005;47(2–3):91–110. doi: 10.1007/s10725-005-3478-x.
- Raghavan V. Role of 2,4-dichlorophenoxyacetic acid (2,4-D) in somatic embryogenesis on cultured zygotic embryos of Arabidopsis: cell expansion, cell cycling, and morphogenesis during continuous exposure of embryos to 2,4-D. Am J Bot. 2004;91(11):1743–1756. doi: 10.3732/ajb.91.11.1743.
- Elhiti M, Stasolla C, Wang A. Molecular regulation of plant somatic embryogenesis. In Vitro Cell Dev Biol-Plant. 2013;49(6):631–642. doi: 10.1007/s11627-013-9547-3.
- Luo JP, Jiang ST, Pan LJ. Enhanced somatic embryogenesis by salicylic acid of Astragalus adsurgens pall.: relationship with H2O2 production and H2O2-metabolizing enzyme activities. Plant Sci. 2001;161(1):125–132. doi: 10.1016/S0168-9452(01)00401-0.
- Chen B, Maas L, Figueiredo D, et al. BABY BOOM regulates early embryo and endosperm development. Proc Natl Acad Sci USA. 2022;119(25):e2201761119. doi: 10.1073/pnas.2201761119.
- Boutilier K, Offringa R, Sharma VK, et al. Ectopic expression of BABY BOOM triggers a conversion from vegetative to embryonic growth. Plant Cell. 2002;14(8):1737–1749. doi: 10.1105/tpc.001941.
- Passarinho P, Ketelaar T, Xing M, et al. BABY BOOM target genes provide diverse entry points into cell proliferation and cell growth pathways. Plant Mol Biol. 2008;68(3):225–237. doi: 10.1007/s11103-008-9364-y.
- Horstman A, Willemsen V, Boutilier K, et al. AINTEGUMENTA-LIKE proteins: hubs in a plethora of networks. Trends Plant Sci. 2014;19(3):146–157. doi: 10.1016/j.tplants.2013.10.010.
- Aida M, Beis D, Heidstra R, et al. The PLETHORA genes mediate patterning of the Arabidopsis root stem cell niche. Cell. 2004;119(1):109–120. doi: 10.1016/j.cell.2004.09.018.
- Galinha C, Hofhuis H, Luijten M, et al. PLETHORA proteins as dose-dependent master regulators of Arabidopsis root development. Nature. 2007;449(7165):1053–1057. doi: 10.1038/nature06206.
- Florez SL, Erwin RL, Maximova SN, et al. Enhanced somatic embryogenesis in Theobroma cacao using the homologous BABY BOOM transcription factor. BMC Plant Biol. 2015;15:121. doi: 10.1186/s12870-015-0479-4.
- El OS, Schnell J, Abdeen A, et al. Control of somatic embryogenesis and embryo development by AP2 transcription factors. Plant Mol Biol. 2010;74(4–5):313–326. doi: 10.1007/s11103-010-9674-8.
- Gordon-Kamm B, Sardesai N, Arling M, et al. Using morphogenic genes to improve recovery and regeneration of transgenic plants. Plants. 2019;8:38. doi: 10.3390/plants8020038.
- Morcillo F, Gallard A, Pillot M, et al. EgAP2-1, an AINTEGUMENTA-like (AIL) gene expressed in meristematic and proliferating tissues of embryos in oil palm. Planta. 2007;226(6):1353–1362. doi: 10.1007/s00425-007-0574-3.
- Deng W, Luo K, Li Z, et al. A novel method for induction of plant regeneration via somatic embryogenesis. Plant Sci. 2009;177(1):43–48. doi: 10.1016/j.plantsci.2009.03.009.
- Heidmann I, Lange B, Lambalk J, et al. Efficient sweet pepper transformation mediated by the BABY BOOM transcription factor. Plant Cell Rep. 2011;30(6):1107–1115. doi: 10.1007/s00299-011-1018-x.
- Lowe K, Wu E, Wang N, et al. Morphogenic regulators baby boom and wuschel improve monocot transformation. Plant Cell. 2016;28(9):1998–2015. doi: 10.1105/tpc.16.00124.
- Li M, Wrobel-Marek J, Heidmann I, et al. Auxin biosynthesis maintains embryo identity and growth during BABY BOOM-induced somatic embryogenesis. Plant Physiol. 2022;188(2):1095–1110. doi: 10.1093/plphys/kiab558.
- Horstman A, Li M, Heidmann I, et al. The BABY BOOM transcription factor activates the LEC1-ABI3-FUS3-LEC2 network to induce somatic embryogenesis. Plant Physiol. 2017;175(2):848–857. doi: 10.1104/pp.17.00232.
- Bao A, Zhang C, Huang Y, et al. Genome editing technology and application in soybean improvement. Oil Crop Sci. 2020;5(1):31–40. doi: 10.1016/j.ocsci.2020.03.001.
- Li SN, Cheng P, Bai YQ, et al. Analysis of soybean somatic embryogenesis using chromosome segment substitution lines and transcriptome sequencing. Genes. 2019;10:943. doi: 10.3390/genes10110943.
- Yu CS, Lin CJ, Hwang JK. Predicting subcellular localization of proteins for gram-negative bacteria by support vector machines based on n-peptide compositions. Protein Sci. 2004;13(5):1402–1406. doi: 10.1110/ps.03479604.
- Tamura K, Peterson D, Peterson N, et al. MEGA5: molecular evolutionary genetics analysis using maximum likelihood, evolutionary distance, and maximum parsimony methods. Mol Biol Evol. 2011;28(10):2731–2739. doi: 10.1093/molbev/msr121.
- Hu B, Jin J, Guo A-Y, et al. GSDS 2.0: an upgraded gene feature visualization server. Bioinformat. 2015;31(8):1296–1297. doi: 10.1093/bioinformatics/btu817.
- Lee TH, Tang H, Wang X, et al. PGDD: a database of gene and genome duplication in plants. Nucleic Acids Res. 2013;41(Database issue):D1152–1158. doi: 10.1093/nar/gks1104.
- Liu W, Xie Y, Ma J, et al. IBS: an illustrator for the presentation and visualization of biological sequences. Bioinformat. 2015;31(20):3359–3361. doi: 10.1093/bioinformatics/btv362.
- Büyük İ, İlhan E, Şener D, et al. Genome-wide identification of CAMTA gene family members in Phaseolus vulgaris L. and their expression profiling during salt stress. Mol Biol Rep. 2019;46(3):2721–2732. doi: 10.1007/s11033-019-04716-8.
- Li Q, Fan CM, Zhang XM, et al. Validation of reference genes for real-time quantitative PCR normalization in soybean developmental and germinating seeds. Plant Cell Rep. 2012;31(10):1789–1798. doi: 10.1007/s00299-012-1282-4.
- Wang X, Fan C, Zhang X, et al. BioVector, a flexible system for gene specific-expression in plants. BMC Plant Biol. 2013;13:198. doi: 10.1186/1471-2229-13-198.
- Wang J, Wang J, Ma C, et al. QTL mapping and data mining to identify genes associated with the Sinorhizobium fredii HH103 T3SS effector NopD in soybean. Front Plant Sci. 2020;11:453. doi: 10.3389/fpls.2020.00453.
- Guolong Y, Jianan Z, Jinhui W, et al. A soybean NAC homolog contributes to resistance to Phytophthora sojae mediated by dirigent proteins. Crop J. 2022;10(2):332–341. doi: 10.1016/j.cj.2021.08.009.
- Costantino P, Spanò L, Pomponi M, et al. The T-DNA of Agrobacterium rhizogenes is transmitted through meiosis to the progeny of hairy root plants. J Mol Appl Genet. 1984;2(5):465–470.
- David C, Chilton MD, Tempé J. Conservation of T-DNA in plants regenerated from hairy root cultures. Nat Biotechnol. 1984;2(1):73–76. doi: 10.1038/nbt0184-73.
- Collier R, Fuchs B, Walter N, et al. Ex vitro composite plants: an inexpensive, rapid method for root biology. Plant J. 2005;43(3):449–457. doi: 10.1111/j.1365-313X.2005.02454.x.
- Kereszt A, Li D, Indrasumunar A, et al. Agrobacterium rhizogenes-mediated transformation of soybean to study root biology. Nat Protoc. 2007;2(4):948–952. doi: 10.1038/nprot.2007.141.
- Zhen HL, Xue HD, Juan L, et al. The Type-B cytokinin response regulator ARR1 inhibits shoot regeneration in an ARR12-Dependent manner in Arabidopsis. Plant Cell. 2020;32(7):2271–2291. doi: 10.1105/tpc.19.00022.
- Fan M, Xu C, Xu K, et al. Lateral organ boundaries domain transcription factors direct callus formation in Arabidopsis regeneration. Cell Res. 2012;22(7):1169–1180. doi: 10.1038/cr.2012.63.
- Hoque ME, Mansfield JW. Effect of genotype and explant age on callus induction and subsequent plant regeneration from root-derived callus of indica rice genotypes. Plant Cell, Tissue Organ Cult. 2004;78(3):217–223. doi: 10.1023/B:TICU.0000025640.75168.2d
- Franklin G, Sheeba CJ, Lakshmi SG. Regeneration of eggplant (Solanum melongenaL.) from root explants. In Vitro Cell Dev Biol Plant. 2004;40(2):188–191. doi: 10.1079/IVP2003491.
- Tóth K, Batek J, Stacey G. Generation of soybean (Glycine max) transient transgenic roots. 2016;1:1–13. doi: 10.1002/cppb.20017.
- Zhou Y, Liu W, Sun S, et al. Cloning and expression analysis of soybean regeneration-related gene GmWUS. Chinese Journal of Oil Crops Sciences. 2014;6:17–22. doi: 10.7505/j.issn.1007-9084.2014.06.003.
- Cao D, Hou W, Song S, et al. Assessment of conditions affecting agrobacterium rhi-zogenes-mediated transformation of soybean. Plant Cell Tiss Organ Cult. 2009;96(1):45–52. doi: 10.1007/s11240-008-9458-x.
- Wei R, Li G, Seymour AB. High-throughput and multiplexed LC/MS/MRM method for targeted metabolomics. Anal Chem. 2010;82(13):5527–5533. doi: 10.1021/ac100331b.
- Lee HW, Kim NY, Lee DJ, et al. LBD18/ASL20 regulates lateral root formation in combination with LBD16/ASL18 downstream of ARF7 and ARF19 in Arabidopsis. Plant Physiol. 2009;151(3):1377–1389. doi: 10.1104/pp.109.143685.
- Lee K, Park OS, Seo PJ. JMJ30-mediated demethylation of H3K9me3 drives tissue identity changes to promote callus formation in Arabidopsis. Plant J. 2018;95(6):961–975. doi: 10.1111/tpj.14002.
- Méndez-Hernández HA, Ledezma-Rodríguez M, Avilez-Montalvo RN, et al. Signaling overview of plant somatic embryogenesis. Front Plant Sci. 2019;10:77. doi: 10.3389/fpls.2019.00077.
- Neves M, Correia S, Cavaleiro C, et al. Modulation of organogenesis and somatic embryogenesis by ethylene: an overview. Plants. 2021;10:1208. doi: 10.3390/plants10061208.
- Wang L, Liu N, Wang T, et al. The GhmiR157a–GhSPL10 regulatory module controls initial cellular dedifferentiation and callus proliferation in cotton by modulating ethylene-mediated flavonoid biosynthesis. J Exp Bot. 2018;69(5):1081–1093. doi: 10.1093/jxb/erx475.
- Ghosh A, Islam T. Genome-wide analysis and expression profiling of glyoxalase gene families in soybean (Glycine max) indicate their development and abiotic stress specific response. BMC Plant Biol. 2016;16:87. doi: 10.1186/s12870-016-0773-9.
- Schmutz J, Cannon SB, Schlueter J, et al. Genome sequence of the palaeopolyploid soybean. Nature. 2010;463(7278):178–183. doi: 10.1038/nature08670.
- Shimomura M, Kanamori H, Komatsu S, et al. The Glycine max cv. Enrei genome for improvement of Japanese soybean cultivars. Int J Genomics. 2015;2015:358127. doi: 10.1155/2015/358127.
- Shen Y, Liu J, Geng H, et al. De novo assembly of a Chinese soybean genome. Sci China Life Sci. 2018;61(8):871–884. doi: 10.1007/s11427-018-9360-0.
- Xu H, Guo Y, Qiu L, et al. Progress in soybean genetic transformation over the last decade. Front Plant Sci. 2022;13:900318. doi: 10.3389/fpls.2022.900318.
- Hu Y, Zhou L, Yang Y, et al. The gibberellin signaling negative regulator RGA-LIKE3 promotes seed storage protein accumulation. Plant Physiol. 2021;185(4):1697–1707. doi: 10.1093/plphys/kiaa114.
- Langhansová L, Konrádová H, Vanĕk T. Polyethylene glycol and abscisic acid improve maturation and regeneration of panax ginseng somatic embryos. Plant Cell Rep. 2004;22(10):725–730. doi: 10.1007/s00299-003-0750-2.
- Stasolla C, Yeung EC. Recent advances in conifer somatic embryogenesis: improving somatic embryo quality. Plant Cell, Tissue Organ Cult. 2003;74(1):15–35. doi: 10.1023/A:1023345803336.
- Marhava P, Hoermayer L, Yoshida S, et al. Re-activation of stem cell pathways for pattern restoration in plant wound healing. Cell. 2019;177(4):957–969.e13. doi: 10.1016/j.cell.2019.04.015.
- Chen K, Li GJ, Bressan RA, et al. Abscisic acid dynamics, signaling, and functions in plants. J Integr Plant Biol. 2020;62(1):25–54. doi: 10.1111/jipb.12899.