Abstract
Lavender (Lavandula angustifolia Mill.) essential oil is of high medicinal and economic importance. The preliminary results of this study found that lavender tissue culture seedlings contained 2.1% essential oil, which was 21 times that of callus tissue. However, the mechanisms involved in its synthesis are unclear. This study used transcriptome sequencing of L. angustifolia callus and tissue culture seedlings to compare changes in differentially expressed genes (DEGs) and metabolic pathways. Transcriptome analysis revealed 2394 DEGs in callus and tissue culture seedlings. Several DEGs were enriched in plant hormone signal transduction, as well as the biosynthesis of terpenoids and other secondary metabolites. In the terpenoid biosynthesis pathway, 93 genes were differentially expressed, and four receptors protein genes (BRI1) of brassinosteroid (BR) hormone were significantly differentially expressed. This study provides deeper insights into regulatory genes involved in terpenoid biosynthesis and BR signal transduction in L. angustifolia, contributing to the genetic improvement of L. angustifolia.
Introduction
Lavender (Lavandula angustifolia Mill.) is a perennial aromatic ornamental plant, belonging to the family Labiatae. Lavender produces various secondary metabolites, including linalyl acetate, linalool, eucalyptol and pinene, which have antioxidant, antibacterial and anticholinesterase activities, as well as analgesic, disinfectant and bactericidal effects. These secondary metabolites are the main components of lavender essential oil. Lavender essential oil is widely used in food, medicine, aromatherapy, etc [Citation1,Citation2]. to promote sleep, reduce anxiety and treat dementia [Citation3–5].
Terpenoids in lavender play an important role as hormones, membrane fluidity regulators (e.g. cholesterol) and insect repellents [Citation6]. The unique fragrance of plants is partly due to terpenoids, which also play a crucial role in plant defense against pests [Citation7,Citation8]. Terpenoids have high medicinal value due to their anticancer, antimalarial and antimicrobial activities [Citation9]. In lavender, terpenoid biosynthesis involves the mevalonate (MVA) pathway and the 2-C-methyl-D-erythritol-4-phosphate (MEP) pathway, which is also a carbon skeleton biosynthesis pathway for chlorophyll, carotene, pentadiene, cytokinin, ABA, BR, etc [Citation10]. The differences between the two synthetic pathways include the mechanism of terpenoid precursor synthesis, metabolites formed and location in plant cells [Citation11].
Previous studies have indicated significant differences in the content of essential oil between callus and tissue culture seedlings. Utilizing callus and tissue culture seedlings for extracting secondary metabolites such as essential oil can potentially overcome the limitations of field production and enable factory bioreactor production of target products. Bushra et al. [Citation12] conducted a study comparing the essential oil content between lavender callus and leaves. The results demonstrated that when the components extracted from lavender callus and leaves were the same, the callus extract contained a higher proportion of components. For instance, the linoleic acid content in the lavender callus extract was 57.84%, whereas it was 47.63% in the leaf extract [Citation12].
In this study, transcriptome sequencing was performed to analyze and compare differentially expressed genes (DEGs) in callus tissue and tissue culture seedlings of L. angustifolia. The synthesis and regulatory pathways of terpenoids and related phytohormones were predicted and the regulatory mechanism of terpenoid synthesis in L. angustifolia was predicted in silico. The findings of this study provide a reference for studying the metabolic regulation of terpenoid synthesis in L. angustifolia.
Materials and methods
Callus and tissue-cultured seedlings
The leaves of fresh seedlings of Lavandula angustifolia Mill were used as explants. The three-year-old lavender seedlings utilized in this research were sourced from Yili Lavender Company. The explants were poured into a triangular flask containing 0.2% detergent, shaken for 10 min in a shaker and washed with running water for 15 min until there was no foam. After 75% alcohol disinfection for 30 s and 0.1% mercuric chloride sterilization for 8 min, the explants were rinsed with sterile water eight times, inoculated in MS (MURASHIGE and SKOOG) medium with 2,4-D (2,4-dichlorophenoxyacetic acid) 1.0 mg/L and placed in a light incubator. The culture conditions were 25 ± 2 °C, 12 h light and 12 h dark every day, with the light intensity being 40 μmol·m−2·s−1. The callus was sub-cultured in MS medium supplemented with 1.0 mg/L 6-BA (6-benzylaminopurine) and 0.1 mg/L NAA (naphthalene acetic acid) to induce buds () [Citation13]. The tissue culture seedlings were subcultured for one month. Each sample had three seedlings, excluding roots. The experiment was repeated thrice. Samples (Callus and tissue culture seedlings) were quickly frozen in liquid nitrogen. Transcriptome sequencing was performed at Qingdao Baimaike Biotechnology Co., Ltd. (Qingdao City, Shangdong Province, China; http://www.biomarker.com.cn/about-us/contact-us). Each sample had three biological replicates and the determination of chemical composition content was carried out at the College of Agriculture Science and Engineering of Liaocheng University (Liaocheng City, Shangdong Province, China).
Essential oil (EO) extraction and gas chromatography-mass spectrometry (GC-MS) analysis
EO was extracted from lavender callus and tissue-cultured seedlings by micro distillation and ultrasonic treatment. The lavender callus and tissue-cultured seedlings were dried at 45 °C and 0.5 g samples were extracted by micro distillation using the Eppendorf micro distillatory (Germany) system. The sample bottle was heated to 108 °C at a rate of 20 °C/min for 90 min, during which ultrasonication was repeated thrice for 30 min each time and heated to 112 °C at a rate of 20 °C/min for 30 min. An Agilent 5975 GC–MSD system was applied to the GC–MS analyses investigated. Helium served as the carrier gas for the Innowax FSC column, measuring 60 meters by 0.25 mm with a film thickness of 0.25 mm [Citation14]. The organic layer entered the collection bottle for separation and was analyzed by GC-MS. Oil yield was calculated by dividing the oil content by dry weight (DW) [Citation3,Citation15,Citation16].
RNA extraction and transcriptome sequencing
Callus and seedlings of over 500 mg were collected for RNA extraction (cat. no. Z3351; Promega, Beijing, China). The NEBNext SuperTM RNA Library Preparation Kit (cat. no. E3330S; New England Biolabs, Ipswich, MA, USA) was used and a total of 1 μg RNA was used for each sample as input material for library generation. RNA-Seq libraries were successfully constructed from both callus and tissue culture seedling samples. For each treatment condition, three biological replicates were included to enhance the reliability and statistical power of the data. The quality of the library was evaluated by Agilent Bioanalyzer 2100 (Agilent, USA). Illumina sequencing of the purified cDNA library was performed at Biomark Technologies (Beijing, China, http://www.biomarker.com.cn/about-us/contact-us).
Transcriptome data assembly
Following the removal of sequencing adaptors and primer sequences in Reads, the filtration of inferior data, and maintaining data integrity, Trinity software segmented the sequencing Reads into shorter segments (K-mer), which were subsequently elongated into longer segments (Contig), utilizing the intersection of these segments to compile the fragment set (Component). Ultimately, the sequence of transcripts in each set of fragments was pinpointed through the application of the De Bruijn diagram technique and sequencing data [Citation17]. Trinity software’s specifications included a minimum assembly length of 200 bp for contigs and insert sizes of 500 bp.
Quality evaluation and differential gene expression identification
Clean readings, including reads, adapters and reads containing poly-N generated after the removal of low-quality data and Q30 were used to evaluate the quality of clean reads. The HISAT2 software (https://daehwankimlab.github.io/hisat2/) was used [Citation18]. Gene function analysis was performed using the following databases: NCBI non-redundant protein sequence (Nr), NCBI non-redundant nucleotide sequence (Nt), protein family (Pfam), homologous protein cluster (KOG/COG), Swiss-Prot, Kyoto Encyclopedia of Genes and Genomes (KEGG) homologous database and gene ontology (GO). DEG sequences were compared and analyzed using the KEGG database. With q < 0.05 as the standard [Citation19], each pathway in KEGG was analyzed to identify significantly enriched pathways in DEGs. Gene function annotation analysis was performed on the pathways.
Gene expression levels were calculated using fragments per thousand bases per million mapped reads (FPKM). DESeq2 software was used to screen the DEGs, which were defined as |log2FoldChange| > 1.5, p-adjust < 0.01 [Citation18].
Quantitative real-time PCR analysis
To verify the accuracy of the transcriptome data, we randomly selected six different genes for expression level verification lists the primer sequences used for qPCR validation in this study. The Aidlab reverse transcription kit (cat. no. PC1802; Aidlab Biotechnologies Co., Ltd, Beijing, China)was used for reverse transcription. qRT-PCR was performed using the Bio-Rad CFX96 (Shanghai, China) system. The steps were as follows: After 95 °C for 3 min, it was 95 °C for 10 s and 60 °C for 30 s for 39 cycles. The melt curve analysis was 60 ∼ 90 °C, + 1 °C/cycle.
Table 1. List of primers for real-time fluorescence quantification.
The reaction volume of qRT-PCR was 20 μL, including 2X SYBR Green Supermix (cat. no. F0202-100T; LABLEAD, Bejing, China) 10 μL, forward primer (200 nmol/L) 0.5 μL, reverse primer (200 nmol/L) 0.5 μL, cDNA 1 μL and ddH2O 8 μL. Relative gene expression was calculated using the 2-ΔΔCt method [Citation20].
Data analysis
SPSS 23.0 (https://www.ibm.com/support/pages/spss-statistics-230-fix-pack-3) and Microsoft Excel 2016 were used for statistical analysis. One-way analysis of variance (ANOVA) was applied to assess the differences. Differences were considered statistically significant at the level of p < 0.05.
Results
EO content
The difference of EOs between callus and tissue culture seedlings of L.angustifolia was more accurately determined by gas chromatography-mass spectrometry (GC-MS). The essential oil content in the callus was 0.2%, while in tissue culture seedlings, it was 2.1% (). The main component of essential oil, linalool, was found to be 0.4% in callus and 19.6% in tissue culture seedlings (). Another major component, lavender acetate, was present at 0.3% in callus and 14.3% in tissue culture seedlings (). The results indicate that the content of EO, as well as its main components linalool and lavender acetate, in callus was lower than that in tissue culture seedlings.
Quality assessment of RNA-Seq data
A total of 39.01 Gb clean data (accession number PRJNA929460) was obtained by high-throughput sequencing. The Q30 value of each sample was above 93.18%, representing high quality. More than 68.41% of the clean data were mapped to the genome, of which more than 80.17% were Multi mapped reads (). These results suggested that the transcriptome data was sufficient to identify DEGs further.
Table 2. Quality of sequencing data.
Identification and verification of DEGs
Overall, 2394 DEGs were found between lavender callus and tissue-cultured seedlings, including 1561 up-regulated genes and 833 down-regulated genes. To verify the reliability of RNA-seq data of DEGs, six DEGs (c78212, c78102, c77542, c78257, c76140 and c64631) were randomly selected for qRT-PCR analysis. The results showed that the expression trends of DEGs between RNA-seq and qRT-PCR were similar, indicating that RNA-seq analysis was reliable ().
Figure 3. Validation of the gene expression levels estimated from RNA sequencing (RNA-Seq) data using quantitative real-time PCR (qRT-PCR) and six target genes of lavandula angustifolia. Different letters represent significant differences between different groups (p < 0.05).
Note: Callus: Callus in tissue culture; Seedling: Tissue Cultured Seedling
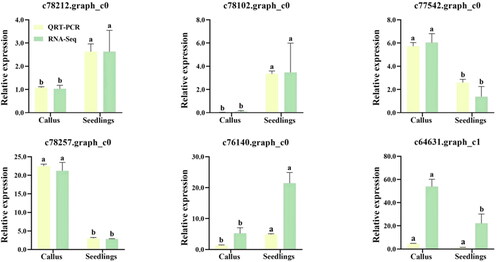
GO classification of DEGs
Using GO annotations, a total of 32,442 unigenes were obtained from Lavender callus and tissue culture seedlings, including 1739 differentially expressed genes. The DEGs can be divided into three categories: cellular composition, molecular function and biological processes. The differentially expressed genes in the items of cellular component were mainly concentrated in membrane, cell and organelle, accounting for 24.52%, 22.81%, and 17.29%, respectively. Among the DEGs in molecular function, the highest number of genes was associated with ‘catalytic activity’, followed by ‘binding’ with 859 and 856 entries, respectively, accounting for 42.32% and 42.17% of the total. Among the DEGs in biological process, ‘metabolic process’ and ‘cellular process’ accounted for the main GO entries, 750 and 625, respectively, followed by 529 single-organism process entries, accounting for 25.30%, 21.09% and 17.85%, respectively. There were 294 differentially expressed genes in biological regulation entries, accounting for 9.92%; these may be related to the development of Lavender callus ().
Figure 4. Gene ontology (GO) analysis of DEGs in callus and tissue culture seedlings of Lavandula angustifolia.
Note: DEG: differentially expressed gene. X-axis shows GO terms. Y-axis on the left shows the percentage of the genes annotated to this term in total number of annotated genes. The Y-axis on the right shows the number of genes annotated to the term.The topGO version 2.28.0 was used to analyze the data. (http://www.bioconductor.org/packages/release/bioc/html/topGO.html).
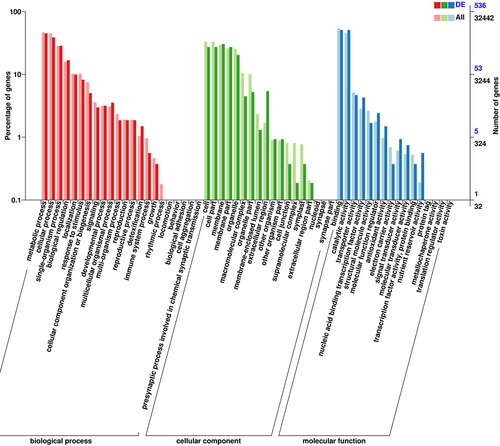
COG functional classification of DEGs
The COG functional classification showed that the DEGs were most abundant in biosynthesis, transport and catabolism (93, 12.42%), followed by the transport and metabolism of carbohydrates (80, 10.68%). Notably, the Signal transduction mechanisms cluster (64, 8.54%), Energy production and conversion cluster (32, 4.27%) and Cell cycle control, cell division, chromosome partitioning cluster (8, 1.07%) may play important roles in the growth and differentiation of Lavender callus and the synthesis of secondary metabolites ().
KEGG pathway annotation of DEGs
In the KEGG pathway, 815 DEGs were found to be abundant and categorized into 123 pathways. Of these, 575 up-regulated genes were annotated to 116 pathways, 240 down-regulated genes were annotated to 97 pathways (). There were 60 regulatory genes assigned to the ‘The plant hormone signal transduction’ pathway, accounting for 10.43% of the up-regulated genes and 17 down-regulated genes, accounting for 7.08% of the down-regulated genes. The ‘Phenylpropanoid biosynthesis’ pathway accounted for up to 25 (10.42%) of the down-regulated genes and 20 (3.48%) of the up-regulated genes in this pathway. In addition, ‘Glycolysis/Gluconeogenesis’ and ‘Porphyrin and chlorophyll metabolism’ were found in both up-regulated and down-regulated genes.
Figure 6. KEGG pathway enrichment analysis of DEGs of Lavandula angustifolia.
A: up-regulated genes; B: down-regulated genes.
Note; Each dot represents a KEGG pathway. Y-axis is -log10 (Q-value) and X-axis is the enrichment factor. The larger the factor is, the higher the enrichment level of the pathway. The color of the dots represents the q-value (adjusted p-value). A smaller q-value indicates a more significant or reliable enrichment. The size of the dots represents the number of DEGs enriched in this pathway. The larger the dot, the more genes it contains.
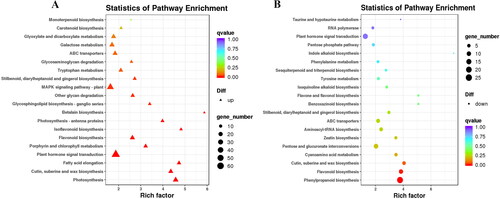
DEGs related to terpenoid metabolism
In this study, consistent with the accumulation of terpenoids in lavender callus and tissue-cultured seedlings, genes involved in terpenoid biosynthesis were significantly upregulated, including 1-deoxy-D-xylulose 5-phosphate synthase (DXS), 1-deoxy-D-xylulose 5-phosphate reductoisomerase (DXR), limonene synthase (LIS), cytochrome P450 (P450), linalool synthase (LS), geranylgeranyl diphosphate synthase (FPPS), geranyl diphosphate synthase small subunit (GPPS), etc ().
Figure 7. Potential regulatory mechanisms of terpene biosynthesis in callus and tissue culture seedlings of Lavandula angustifolia.
Note: Red indicates up-regulated genes, Green indicates down-regulated genes. See also Supplemental Table S1.
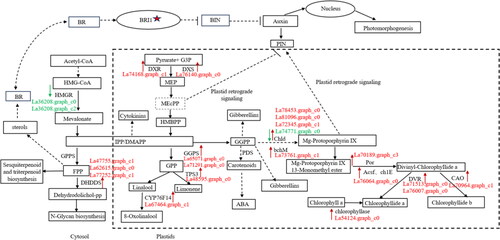
In addition, genes involved in the synthesis of secondary metabolites, including arylacetamide deacetylase (AADAC), 2,3-oxidosqualene cyclase (OS) and hydroxymethylglutaryl-CoA reductase (HMGR), were significantly downregulated.
DEGs related to carbohydrate metabolic pathway
In plants, the metabolism and transformation of sugars, which provide the carbon skeleton for terpenoid synthesis, are closely related to plant secondary metabolism. In this study, transcriptome sequencing revealed several DEGs involved in the pentose and gluconate mutual transformation pathway and sugar metabolism pathway between callus and tissue culture seedlings of L. angustifolia (). These DEGs regulated the entose and gluconate mutual transformation and sugar metabolism pathways.
BRI1 and BR hormones are involved in terpenoid metabolic pathways
Plant BRI1 is distributed primarily within the cell membrane, with only a small fraction present in the cell wall (). The results showed that the transcriptional regulation of target genes had the same trend in BR and auxin signaling pathways and common regulatory elements.
Figure 9. Differentially expressed genes of hormone metabolic pathways in callus and tissue culture seedlings of Lavandula angustifolia.
Note: Red indicates up-regulated genes, Green indicates down-regulated genes. See also Supplemental Table S1.
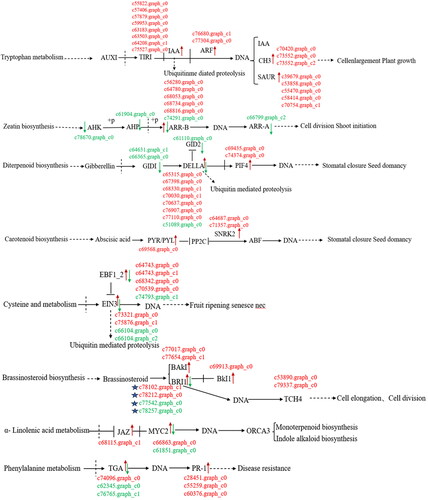
In plants, BRI1 is a leucine-rich repeat receptor-like protein kinase. According to the transcriptome sequencing results, four BRI1 genes were differentially expressed: LaBRI1-1–4 (c77542, c78102, c78212, c78257). To classify the LaBRI1 genes, a phylogenetic tree was constructed with 34 Arabidopsis thaliana and 4 LaBRI1 genes. The phylogenetic tree was divided into 8 branches; the 4 LaBRI1 genes were distributed in 3 branches. LaBRI1-1 and LaBRI1-4 were in the same branch, indicating that the two are highly related ().
Motif analysis showed that the 4 BRI1 protein family members shared conserved motifs (motifs 5–8), all of which contained LRR and protein kinase domains (). They are typical structural BRI1 gene families of plants [Citation21]. The BR receptor island domain was identified in LacBRI1-2, which is typical of BRI1, but the leucine-rich protein kinase domain was identified in the other four genes (), indicating that it also belongs to the BRI1 family [Citation22].
Figure 11. Conserved motif and conserved structure of LaBRI1 gene.
Note: A: Conserved motif of LaBRI1 gene B: Conserved structure of LaBRI1 gene.
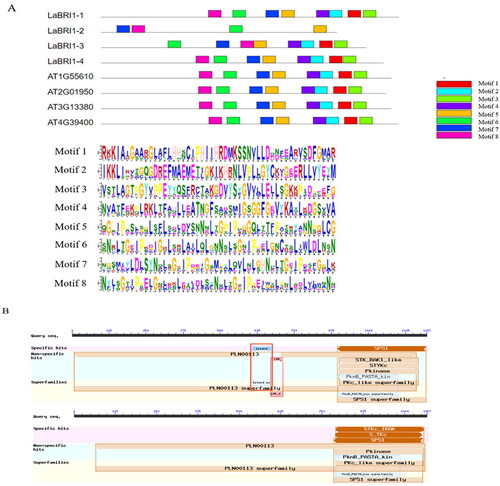
Analysis of the physicochemical properties of the 4 BRI1 proteins showed that the amino acid number was 952–1196, predicted molecular weight was 102.24–128.86 kDa and isoelectric point was 4.89–7.01. Three of the four proteins had an isoelectric point (PI) value < 7, which is acidic. Analysis of protein subcellular localization showed that LaBRI1-1, LaBRI1-3 and LaBRI1-4 are present in the cell membrane and LaBRI1-2 is located in the cell wall ().
Table 3. Predicted physicochemical properties of four BRI1 proteins in Lavandula angustifolia.
Discussion
Though many genes related to the EO biosynthesis pathway in lavender were reported [Citation15,Citation23–25], the molecular mechanism of EO biosynthesis in lavender has not been fully elucidated. This study examined the influence of specific genes on the biosynthesis of essential oil in both callus and tissue-cultured plants using transcriptomic techniques. Transcriptome analysis revealed changes in the expression of certain hypothetical key enzyme-encoding genes related to the regulation of CKs and auxins, including the cyclin gene (CYCD 6), the type-B authentic response regulator gene (ARR-B) and auxin-responsive protein gene (SAUR15)). This indicated that they are maybe regulated by related hormones during development from callus to seedlings. The volatile oil content was low in the callus, indicating that the secondary metabolic synthesis of plants was related to the differentiation of plant organs and tissues. In this study, by comparing the transcriptomes of lavender callus and tissue-cultured seedlings, the molecular mechanism of plant tissue organ differentiation and biosynthesis of secondary metabolites, such as EO, were predicted. The results of our study may provide reference value to breed new lavender varieties with a high EO content in the future.
A total of 2394 differentially expressed genes (1561 up-regulated and 833 down-regulated) were identified in callus and tissue culture seedlings. Enrichment data analysis revealed 102 and 130 differentially expressed genes in GO and KEGG pathways, respectively, associated with signal transduction. There were 35 KEGG pathways associated with secondary metabolic pathways. KEGG pathway analysis activated auxin, cytokinin and BR in callus signaling pathways (). Hormone activation in these pathways triggers a range of gene transcriptions, promotes callus differentiation into tissue culture seedlings and produces secondary metabolites [Citation26]. Various hormones influence the content of essential oils such as aromatic oil and lavender acetate in callus and tissue culture seedlings through complex regulatory networks in the MVA and MEP terpenoid biosynthesis pathways. Methylerythritol cyclodiphosphate is an intermediate of metabolic pathways and also affects the synthesis of related hormones, possibly affecting plant growth and development [Citation27].
In plants, terpenoids are mainly synthesized through the cytoplasmic MVA and plastidal MEP pathways. The isoprenoid produced through these pathways is the precursor of hormones, such as ABA, CK, GA3 and BR and primary metabolites, including chlorophyll, ubiquinone, carotenoids and plastoquinone () [Citation10]. Therefore, these pathways are closely related to plant growth and development [Citation28]. In the MVA pathway, HMGR is the first important rate-limiting enzyme and an important regulatory point of the pathway [Citation29]. The gene family encoding HMGR has been identified in many plants, including Arabidopsis thaliana L., Brassica juncea and Solanum lycopersicum [Citation30]. In Arabidopsis, HMGR plays an important role in sterol and triterpene biosynthesis [Citation31]. Notably, this study revealed that HMGR expression was significantly downregulated in tissue-cultured seedlings. The role of HMGR in lavender terpene synthesis warrants further investigation. DXS is believed to be a rate-limiting enzyme in the MEP pathway [Citation32,Citation33]. This study showed that the expression of DXS in lavender tissue-cultured seedlings was higher than that in the callus. In addition, DXS expression showed tissue specificity and was specifically detected in the chloroplasts. The lack of chloroplasts in the callus leads to a low content of DXS [Citation34,Citation35]. This study also showed that the expression of DXR in lavender tissue culture seedlings was higher than that in callus tissue. In our study, TPS genes were down-regulated compared the Callus to tissue culture seedlings of Lavandula angustifolia, which is consistent with previous findings [Citation36,Citation37]. Interestingly, the expression of the DHDDS (Dehydrodolichyl diphosphate synthase) gene was also significantly up-regulated in the lavender seedlings. The protein encoded by it catalyzes the elongation of the cis-isoprene chain to produce the polyisoprene backbone of polyterpene alcohols. This gene was not reported in the previous terpenoid biosynthesis pathway and may be of great significance.
In this study, some genes involved in chlorophyll biosynthesis and plastid retrograde signaling were found to be different for example Chld, bchM, DVR, CAO and chlorophyllase genes (). The main function of plastid signaling is to maintain chloroplast development and the normal operation of photosynthetic function by regulating the expression of photosynthetic-related genes in the nucleus. Additionally, plastid retrograde signals are involved in multiple growth and developmental processes of Arabidopsis thaliana [Citation38,Citation39]. Some researchers believe that plastid retrograde signaling coordinates endogenous hormone signals to form a complex intracellular signal transduction network [Citation40]. In addition, this research also found that MYB-related transcription factor LHY (LHY) and protein CCA1 HIKING EXPEDITION (CHE) were involved in the regulation of Circadian rhythm in lavender tissue culture seedlings. The zinc finger protein CONSTANS (CO) also showed notable upregulation, which was in line with the previous results that the MEP and MVA pathways were regulated by the plant diurnal rhythm and were involved in the regulation of terpenoid emission genes [Citation41].
According to the general consensus, plant brassinosteroids (BRs) are multifunctional and regulate a wide range of important developmental processes by interacting with auxin signaling pathways [Citation42]. The membrane steroid receptor brassinosteroid insensitive 1 (BRI1) binds BR, triggering a chain reaction of cytoplasmic signals and resulting in the expression of BR-related genes. BRI1 family proteins have highly conserved three-dimensional structures [Citation43]. In the terpenoid metabolic pathway of L. angustifolia, endogenous BR hormones can be produced through the MAV pathway and BRI1 affects BR signal transduction [Citation44]. During BR signaling, BRI1 recognizes BR and transmits the BR signal through the phosphorylation and dephosphorylation of BRI1 [Citation45]. Studies have shown that the BR and auxin signaling pathways converge with coregulatory elements targeting genes at the transcriptional level [Citation46]. This study showed significant differences in BRI1 expression associated with BR signal transduction, suggesting that BR and BRI1 play a significant role in the transformation of lavender callus into tissue culture seedlings.
Conclusions
The results indicated significant differences in the essential oil content between callus and tissue culture seedlings of L. angustifolia. The essential oil content and some terpenes in tissue culture seedlings were much higher than those in callus. Transcriptome sequencing data provided important insights into the molecular mechanisms underlying the difference in essential oil content between callus and tissue culture seedlings of L. angustifolia. A total of 2394 differentially expressed genes were identified, including 1561 up-regulated genes and 833 down-regulated genes, mainly involved in the biosynthesis of secondary metabolites. Additionally, 18 differentially expressed genes were found in the terpene synthesis pathway. These genes were involved in the biosynthesis of secondary metabolites such as linalool in L. angustifolia tissue culture seedlings by affecting the metabolic synthesis of hormones and some primary metabolites. Interestingly, four BRI1 genes were differentially expressed in callus and tissue culture seedlings, which has been less reported in relevant literature previously. This suggests that BR hormones may play a significant role in terpenoid synthesis and callus development by regulating cell homeostasis. This study provides insights for the industrial production and variety improvement of lavender essential oil bioreactors.
Authors’ contributions
Methodology, conceived and designed the work, HM and SG; Supervision, HM, SG and QM; Experimental and data analysis, S.C., X.Z., J.W., Y.J. and X.L.; Materials and analysis tools, X.Z. and Y.W.; Formal analysis, SC and QM; Data management, JW, YJ, XL and YW; Writing – original draft, SC and XZ; Writing - review and editing, SC, DZ and HM; Funding, HM. All authors have read and agreed to the published version of the manuscript.
Supplemental Material
Download MS Word (16.4 KB)Acknowledgements
Sequencing services for this study were provided by Qingdao Baimeike technology company.
Disclosure statement
No potential conflict of interest was reported by the author(s).
Data availability statement
All RNA-Seq raw data used in this study were deposited in the Sequence Read Archive (SRA) at NCBI under the accession number PRJNA929460 (https://www.ncbi.nlm.nih.gov/bioproject/PRJNA929460). All other data that support the findings reported in this study are available from the corresponding author [HM] upon reasonable request.
Additional information
Funding
References
- Li H, Li J, Dong Y, et al. Time-series transcriptome provides insights into the gene regulation network involved in the volatile terpenoid metabolism during the flower development of lavender. BMC Plant Biol. 2019;19(1):313. doi: 10.1186/s12870-019-1908-6.
- Li J, Wang Y, Dong Y, et al. The chromosome-based lavender genome provides new insights into Lamiaceae evolution and terpenoid biosynthesis. Hortic Res. 2021;8(1):53–53. doi: 10.1038/s41438-021-00536-9.
- Guo D, Kang K, Wang P, et al. Transcriptome profiling of spike provides expression features of genes related to terpene biosynthesis in lavender. Sci Rep. 2020;10(1):6933. doi: 10.1038/s41598-020-63950-4.
- Prusinowska R, Śmigielski KB. Composition, biological properties and therapeutic effects of lavender (Lavandula angustifolia L.). A review. Herba Polonica. 2014;60(2):56–66. doi: 10.2478/hepo-2014-0010.
- Silva GLd, Luft C, Lunardelli A, et al. Antioxidant, analgesic and anti-inflammatory effects of lavender essential oil. An Acad Bras Cienc. 2015;87(2 Suppl):1397–1408. doi: 10.1590/0001-3765201520150056.
- Tholl D. Terpene synthases and the regulation, diversity and biological roles of terpene metabolism. Curr Opin Plant Biol. 2006;9(3):297–304. doi: 10.1016/j.pbi.2006.03.014.
- Aaron JA, Christianson DW. Trinuclear metal clusters in catalysis by terpenoid synthases. Pure Appl Chem. 2010;82(8):1585–1597. doi: 10.1351/PAC-CON-09-09-37.
- Benabdelkader T, Guitton Y, Pasquier B, et al. Functional characterization of terpene synthases and chemotypic variation in three lavender species of section Stoechas. Physiol Plant. 2015;153(1):43–57. doi: 10.1111/ppl.12241.
- Wang Y, Zou J, Jia Y, et al. The mechanism of lavender essential oil in the Treatment of acute colitis based on "quantity-effect" weight coefficient network pharmacology. Front Pharmacol. 2021;12:644140. doi: 10.3389/fphar.2021.644140.
- Adal E, Eren S. Rosemary and oregano essential oils as natural antioxidant to preserve pistachio puree. J Food Sci Eng. 2019;9(8):318–332. doi: 10.17265/2159-5828/2019.08.003.
- Fan S, Jia Y, Wang R, et al. Multi-omics analysis the differences of VOCs terpenoid synthesis pathway in maintaining obligate mutualism between Ficus hirta Vahl and its pollinators. Front Plant Sci. 2022;13:1006291. doi: 10.3389/FPLS.2022.1006291.
- Bushra A, Zainab S, Sumaya H. Qualitative and quantitative evaluation of active constituents in callus of lavandula angustifolia plant in vitro. Baghdad Sci J. 2020;2(SI):0591–0591. doi: 10.21123/bsj.2020.17.2(si).0591.
- Murashige T, Skoog F. A revised medium for rapid growth and bio assays with tobacco tissue cultures. Physiol Plant. 1962;15(3):473–497. doi: 10.1111/j.1399-3054.1962.tb08052.x.
- Kirimer N, Mokhtarzadeh S, Demirci B, et al. Phytochemical profiling of volatile components of Lavandula angustifolia Miller propagated under in vitro conditions. Ind Crops Prod. 2017;96:120–125. doi: 10.1016/j.indcrop.2016.11.061.
- Falk L, Biswas K, Boeckelmann A, et al. An efficient method for the micropropagation of lavenders: regeneration of a unique mutant. J Essent Oil Res. 2009;21(3):225–228. doi: 10.1080/10412905.2009.9700154.
- Lesage-Meessen L, Bou M, Sigoillot JC, et al. Essential oils and distilled straws of lavender and lavandin: a review of current use and potential application in white biotechnology. Appl Microbiol Biotechnol. 2015;99(8):3375–3385. doi: 10.1007/s00253-015-6511-7.
- Grabherr MG, Haas BJ, Yassour M, et al. Full-length transcriptome assembly from RNA-Seq data without a reference genome. Nat Biotechnol. 2011;29(7):644–652. doi: 10.1038/nbt.1883.
- Ma Q, Song L, Niu Z, et al. Red light regulates the metabolite biosynthesis in the leaves of “Huangjinya” through amino acid and phenylpropanoid metabolisms. Front Plant Sci. 2021;12:810888. doi: 10.3389/fpls.2021.810888.
- Zhang J, Liang L, Xie Y, et al. Transcriptome and metabolome analyses reveal molecular responses of two pepper (Capsicum annuum L.) cultivars to cold stress. Front Plant Sci. 2022;13:975330. doi: 10.3389/fpls.2022.819630.
- Livak KJ, Schmittgen TD. Analysis of relative gene expression data using real-time quantitative PCR and the 2 [-Delta Delta C [T]] normalized to glyceraldehyde-3-phosphate dehydrogenase levels. qRT-PCR was method. Methods. 2001;25(4):402–408. doi: 10.1006/meth.2001.1262.
- Sharma N, Khurana P. Genome-wide identification, characterization and expression analysis of the BRI1 gene family in Triticum aestivum L. Plant Biotechnol Rep. 2022;16(6):777–791. doi: 10.1007/s11816-022-00762-0.
- Bojar D, Martinez J, Santiago J, et al. Crystal structures of the phosphorylated BRI 1 kinase domain and implications for brassinosteroid signal initiation. Plant J. 2014;78(1):31–43. doi: 10.1111/tpj.12445.
- Demissie ZA, Sarker LS, Mahmoud SS. Cloning and functional characterization of β-phellandrene synthase from Lavandula angustifolia. Planta. 2011;233(4):685–696. doi: 10.1007/s00425-010-1332-5.
- Demissie ZA, Cella MA, Sarker LS, et al. Cloning, functional characterization and genomic organization of 1, 8-cineole synthases from Lavandula. Plant Mol Biol. 2012;79(4-5):393–411. doi: 10.1007/s11103-012-9920-3.
- Lane A, Boecklemann A, Woronuk GN, et al. A genomics resource for investigating regulation of essential oil production in Lavandula angustifolia. Planta. 2010;231(4):835–845. doi: 10.1007/s00425-009-1090-4.
- Sato Y, Minamikawa MF, Pratama BB, et al. Autonomous differentiation of transgenic cells requiring no external hormone application: the endogenous gene expression and phytohormone behaviors. Front Plant Sci. 2024;15:1308417–1308417. doi: 10.3389/fpls.2024.1308417.
- Jiang J, Zeng L, Ke H, et al. Orthogonal regulation of phytochrome B abundance by stress-specific plastidial retrograde signaling metabolite. Nat Commun. 2019;10(1):2904. doi: 10.1038/s41467-019-10867-w.
- Pu X, Dong X, Li Q, et al. An update on the function and regulation of methylerythritol phosphate and mevalonate pathways and their evolutionary dynamics. J Integr Plant Biol. 2021;63(7):1211–1226. doi: 10.1111/jipb.13076.
- Rico J, Pardo E, Orejas M. Enhanced production of a plant monoterpene by overexpression of the 3-hydroxy-3-methylglutaryl coenzyme A reductase catalytic domain in Saccharomyces cerevisiae. Appl Environ Microbiol. 2010;76(19):6449–6454. doi: 10.1128/AEM.02987-09.
- Korth KL, Stermer BA, Bhattacharyya MK, et al. HMG-CoA reductase gene families that differentially accumulate transcripts in potato tubers are developmentally expressed in floral tissues. Plant Mol Biol. 1997;33(3):545–551. doi: 10.1023/A:1005743011651.
- Suzuki M, Kamide Y, Nagata N, et al. Loss of function of 3-hydroxy-3-methylglutaryl coenzyme A reductase 1 (HMG1) in Arabidopsis leads to dwarfing, early senescence and male sterility, and reduced sterol levels. Plant J. 2004;37(5):750–761. doi: 10.1111/j.1365-313X.2004.02003.x.
- Jadaun JS, Sangwan NS, Narnoliya LK, et al. Over-expression of DXS gene enhances terpenoidal secondary metabolite accumulation in rose-scented geranium and Withania somnifera: active involvement of plastid isoprenogenic pathway in their biosynthesis. Physiol Plant. 2017;159(4):381–400. doi: 10.1111/ppl.12507.
- Lois LM, Rodríguez-Concepción M, Gallego F, et al. Carotenoid biosynthesis during tomato fruit development: regulatory role of 1-deoxy-D-xylulose 5-phosphate synthase. Plant J. 2000;22(6):503–513. doi: 10.1046/j.1365-313x.2000.00764.x.
- Lv X, Gu J, Wang F, et al. Combinatorial pathway optimization in Escherichia coli by directed co-evolution of rate-limiting enzymes and modular pathway engineering. Biotechnol Bioeng. 2016;113(12):2661–2669. doi: 10.1002/bit.26034.
- Yin X, Yan X, Qian C, et al. Comparative transcriptome analysis to identify genes involved in terpenoid biosynthesis in Agriophyllum squarrosum, a folk medicinal herb native to Asian temperature deserts. Plant Biotechnol Rep. 2021;15(3):369–387. doi: 10.1007/s11816-021-00674-5.
- Degenhardt J, Gershenzon J, Baldwin IT, et al. Attracting friends to feast on foes: engineering terpene emission to make crop plants more attractive to herbivore enemies. Curr Opin Biotechnol. 2003;14(2):169–176. doi: 10.1016/S0958-1669(03)00025-9.
- Wu S, Schalk M, Clark A, et al. Redirection of cytosolic or plastidic isoprenoid precursors elevates terpene production in plants. Nat Biotechnol. 2006;24(11):1441–1447. doi: 10.1038/NBT1251.
- Pesaresi P, Masiero S, Eubel H, et al. Nuclear photosynthetic gene expression is synergistically modulated by rates of protein synthesis in chloroplasts and mitochondria. Plant Cell. 2006;18(4):970–991. doi: 10.1105/tpc.105.039073.
- Woodson JD, Chory J. Coordination of gene expression between organellar and nuclear genomes. Nat Rev Genet. 2008;9(5):383–395. doi: 10.1038/nrg2348.
- Chi W, Sun X, Zhang L. Intracellular signaling from plastid to nucleus. Annu Rev Plant Biol. 2013;64(1):559–582. doi: 10.1146/annurev-arplant-050312-120147.
- Zheng X, Li P, Lu X. Research advances in cytochrome P450-catalysed pharmaceutical terpenoid biosynthesis in plants. J Exp Bot. 2019;70(18):4619–4630. doi: 10.1093/jxb/erz203.
- Chaudhuri A, Halder K, Abdin MZ, et al. Abiotic stress tolerance in plants: brassinosteroids navigate competently. Int J Mol Sci. 2022;23(23):14577. doi: 10.3390/ijms23231457711.
- Han C, Wang L, Lyu J, et al. Brassinosteroid signaling and molecular crosstalk with nutrients in plants. J Genet Genomics. 2023;50(8):541–553. doi: 10.1016/j.jgg.2023.03.004.
- Nakamura A, Fujioka S, Sunohara H, et al. The role of OsBRI1 and its homologous genes, OsBRL1 and OsBRL3, in rice. Plant Physiol. 2006;140(2):580–590. doi: 10.1104/pp.105.072330.
- Irani NG, Di Rubbo S, Mylle E, et al. Fluorescent castasterone reveals BRI1 signaling from the plasma membrane. Nat Chem Biol. 2012;8(6):583–589. doi: 10.1038/nchembio.958.
- Rana S, Hardtke CS. Plant biology: brassinosteroids and the intracellular auxin shuttle. Curr Biol. 2020;30(9):R407–R409. doi: 10.1016/j.cub.2020.02.073.