Abstract
We fabricated nanosized KMgF3 single crystals via a dry pulsed laser ablation process using femtosecond laser pulses. The sizes, shapes, and crystallographic properties of the crystals were evaluated by transmission electron microscopy (TEM). Almost all of the particles were spherical with diameters of less than 100 nm, and they were not highly agglomerated. Selected-area electron diffraction and high-resolution TEM analyses showed that the particles were single crystals. Particle diameter was controlled within a wide range by adjusting the Ar ambient gas pressure. Under low gas pressures (1 and 10 Pa), relatively small particles (primarily 10 nm or less) were observed with a high number density. With increasing pressure, the mean diameter increased and the number density drastically decreased. Vacuum-ultraviolet cathodoluminescence was observed at 140–230 nm with blue shift and broadening of spectrum.
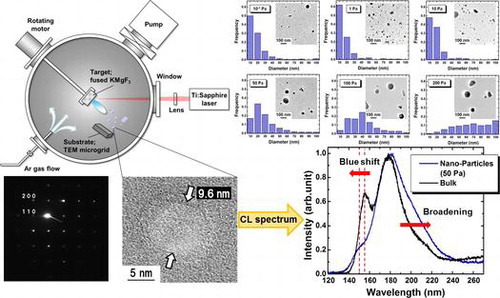
1. Introduction
Ternary metal fluorides such as AIMIIF3 (A = alkaline metal elements; M = bivalent metal elements) have received considerable attention as important inorganic functional materials. They have interesting physical and chemical properties such as ferromagnetism, nonmagnetic insulator behavior, and piezoelectric characteristics along with advantageous optical properties.[Citation1,2]
For example, ternary KF compounds such as KMgF3 and KCaF3 have been studied as scintillators.[Citation3,4] They show fast fluorescence in the vacuum-ultraviolet (VUV) region because of the electronic transitions from the F− 2p valence band to the K+ 3p core band (i.e. crossluminescence).
KMgF3 has excellent characteristics such as homogeneous optical properties, high thermal stability, anisotropy, high optical transparency, and high luminous efficiency.[Citation5–7] Rare earth ion-doped KMgF3 has been widely studied for use in dosimeters, laser rods, and scintillators.[Citation8–10] KMgF3 was also applied as a VUV phosphor in our recent study wherein we grew a KMgF3 thin film phosphor via pulsed laser deposition and developed a field emission VUV lamp using the KMgF3 thin film.[Citation11] The lamp operated in the wavelength range of 140–220 nm, which is the shortest wavelength ever reported for a solid-state phosphor lamp.
Owing to these promising results, it is important to expand this area of research to the nanometer scale. In the past few years, the preparation and characterization of materials on the nanometer scale has provided not only new physics in reduced dimensions, but also the possibility of fabricating novel materials. Particularly in luminescent materials, many researchers have investigated semiconductors based on materials such as Si, SiC, CdSe, and ZnO.[Citation12–24] These studies revealed that quantum effects result in nanoparticles having different luminescence properties in terms of quantum efficiency, luminescent decay, and emission wavelength.[Citation25,26] The most interesting result is that the emission shifts to a shorter wavelength with decreasing particle size. For example, with decreasing particle diameter, Si nanoparticles show a blue shift in luminescence from 900 nm (average particle diameter = 4.8 nm) to 610 nm (average particle diameter = 2.8 nm).[Citation12] This attractive phenomenon must also be present in other multiple phosphors; however, there are only a few reports on fluoride composite materials, and their synthetic routes are limited to liquid-phase methods such as thermal decomposition.[Citation5,27–31]
In this study, we fabricated KMgF3 nanoparticles via a dry process using pulsed laser ablation (PLA). The synthesis of nanocrystals via PLA was recently reported [Citation22,32]; this preparation method has the distinct advantage of high cleanliness because the high-energy laser beam can be focused on a small spot on the surface of the target in the presence of a high-purity inert gas. Thus, PLA is a non-contact process, and contamination from a crucible is avoided.[Citation33,34] In this study, we also applied femtosecond laser pulses as such pulses were previously shown to reduce the differences in the compositional ratio from the source target and particles above 100 nm.[Citation35,36] In addition, it is important to investigate the luminescence properties of nanosized KMgF3 in the VUV region because luminescence in this region is important in applications such as surface treatment, optical cleaning of semiconductor substrates, and sterilization. Although a solid-state phosphor is required as a substitute for the gas phosphor, there are few existing solid-state phosphors that possess high quantum efficiency in the VUV region. Thus, improving the luminescence properties and particularly changing the emission wavelength by atomization would greatly enhance the applications of VUV light sources.
2. Experimental methods
Figure shows a schematic drawing of the experimental setup. The fused KMgF3 target (KF:MgF2 = 1:1) was placed in a vacuum chamber with a base pressure of 10−1 Pa and prepared by melting under Ar:CF4 (95:5) atmosphere at 1220°C. After melting, the target took 1 h to reach 900°C and an additional 48 h to reach room temperature. The target was then irradiated with femtosecond laser pulses at a wavelength of 790 nm, a pulse width of 206 fs, and a repetition rate of 1 kHz. The laser beam was focused on the target with a fluence of 11 J cm–2. The particles were collected on a transmission electron microscopy (TEM) microgrid. For thin film fabrication via PLA, the substrate was generally placed toward the target (on-axial position). In contrast, we placed the TEM microgrid in an off-axial position (x = 25 mm, 50 mm below the target) to prevent the deposition of large particles. For irradiation, the chamber gate valve connected to the pumping system was closed, and ambient Ar gas was leaked into the chamber. Experimental studies have been conducted on the effects of ambient gas pressure on the sizes and shapes of nanoparticles.[Citation37,38] Thus, in this study, the Ar gas pressure was adjusted as the dominant parameter within the range of 10−1 to 200 Pa to investigate its influence on particle size.
3. Results and discussion
The crystallographic properties of the fabricated KMgF3 nanoparticles were investigated by high-resolution TEM (HR-TEM) and selected-area electron diffraction (SAED; Figure ). Figure (a), which was obtained by observing a KMgF3 nanoparticle prepared at 50 Pa at a magnification of 300,000 × , clearly shows the crystal lattice fringes. Figure (b) shows narrow diffraction spots from a KMgF3 nanoparticle that was prepared under an Ar pressure of 50 Pa. This pattern indicates the single crystallinity of the sample. The prominent diffractions of the (200) and (110) planes in the SAED pattern are consistent with cubic KMgF3. Approximately equivalent results were obtained for the particles fabricated at other Ar pressures. These results indicate that nanosized KMgF3 single crystals were successfully fabricated by PLA.
Figure 2. (a) HR-TEM image and (b) SAED pattern of a KMgF3 nanoparticle prepared at an Ar pressure of 50 Pa.
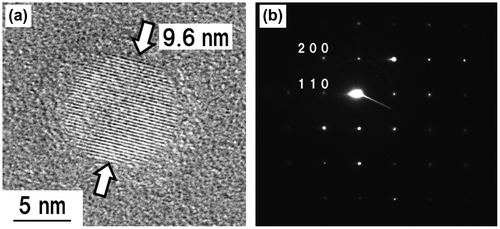
Figure shows the TEM images and size distributions of KMgF3 nanoparticles prepared under indicated Ar pressures. Almost all the particles were spherical with diameters less than 100 nm and did not exhibit considerable agglomeration. Furthermore, almost all the particles with diameters <10 nm were single crystalline for all Ar pressures (Figure (a)). The observed particle shape may be influenced by surface tension during the nucleation and growth of the nanoparticle.[Citation39–43] The size distributions were statistically analyzed using the TEM images by roughly measuring 300 nanoparticles. Since relatively large agglomerates with larger than submicron dimensions were occasionally mixed in with the nanoparticles, these larger particles were excluded from the measurements. The ratio of the volume of submicron particles to the volume of nanoparticles exceeded 10 for all Ar pressures except 0.1 Pa. Under an Ar pressure of 1 Pa, approximately 60% of the particles were less than 10 nm in diameter. In contrast, under higher Ar pressure, the peak of the size distribution histogram shifted toward larger diameter, and the peak width increased (Figure ).
Figure 3. TEM images and size distributions of KMgF3 nanoparticles prepared under indicated gas pressure.
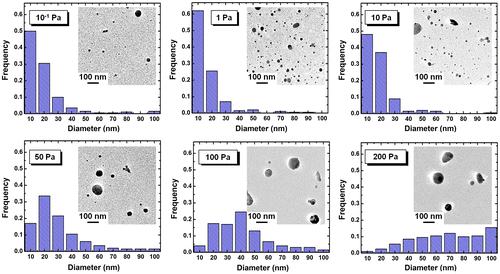
The mean particle diameters and number densities were also measured from TEM images (Figure ). Under an Ar pressure of 1 Pa, we obtained the highest number density of 172.3 μm−2 and the smallest mean diameter of 12 nm. By increasing the Ar pressure to 200 Pa, the number density was dramatically decreased to 4.5 mm−2, and the mean diameter was increased to 54 nm. These results are attributed to the difference in collision frequency among ablated particles and Ar atoms. In the primary phase of gas-phase ablation, the collisions between ablated fine particles and ambient gas atoms play leading roles, resulting in the dissipation of their kinetic energy.[Citation44,45] These effects determine the nucleation and the growth of nanoparticles. High Ar pressure caused frequent collisions, resulting in abundant growth. On the other hand, infrequent collisions resulted in low number densities at pressures under 10−1 Pa. The results suggest that an Ar pressure of 1 Pa is suitable for obtaining a large quantity of fine particles with diameters of several nm. In contrast to these dramatic changes caused by Ar pressure, the fluence and substrate arrangement had little effect on particle diameter in our experiments. For example, increasing the fluence from 7.6 to 16.2 J cm–2 changed the mean diameter from 12 nm (at 14.0 J cm–2) to 14 nm (at 7.6 J cm–2), while changing the substrate arrangement from x = 5 to 35 mm increased the mean diameter from 12 nm (at x = 5 mm) to 25 nm (at x = 35 mm).
During the above measurements, it was difficult to identify particles with diameters of less than 5 nm in the TEM images. We may be able to obtain a higher number density and a more detailed size distribution from the samples prepared under low Ar pressure by using a higher magnification or higher-resolution images.
Figure shows the cathodoluminescence (CL) spectrum of KMgF3 nanoparticles prepared under an Ar pressure of 50 Pa; the CL spectrum of bulk KMgF3 is indicated by the black line in this figure. VUV fluorescence was observed under electron beam excitation in the wavelength region from 140 to 230 nm with a slight change in the spectrum shape. The main peak at 180 nm exhibited a long-wavelength tail. The peak at 150 nm became weak and shifted slightly toward shorter wavelengths. Since submicron-sized agglomerates occasionally became mixed with the nanoparticles in the method used in this study, it was difficult to exclude them from the CL measurements. Thus, we could not determine the relationship between particle size and CL spectrum. Note that the changes in spectral shape such as splitting have been reported in other materials;[Citation46–48] however, this study represents the first such observation in the VUV region.
4. Conclusions
We have successfully fabricated size-controllable, nanosized KMgF3 single crystals via a dry PLA process with femtosecond laser pulses. Almost all the fabricated particles were spherical with diameters less than 100 nm, and the particles were not highly agglomerated. The crystal lattice fringes observed in the HR-TEM images and the spot patterns seen in the SAED patterns indicated that the particles are single crystals. The Ar ambient gas pressure influenced the particle size more strongly than other parameters such as fluence and substrate arrangement. At Ar pressures of approximately 1 and 10 Pa, relatively small particles were produced with high number densities. At higher gas pressures, the mean diameter increased, and the number density decreased dramatically. An Ar pressure of 1 Pa was suitable for obtaining a large quantity of fine particles with diameters of several nanometers in our experiments. Upon electron beam excitation, the KMgF3 nanoparticles emitted VUV fluorescence in the wavelength region from 140 to 230 nm with blue shift and broadening of spectrum. These results demonstrate the possibility of using KMgF3 nanoparticles as a novel VUV phosphor.
Acknowledgements
This work was partly supported by Nanotechnology Platform Program (Molecule and Material Synthesis) of the Ministry of Education, Culture, Sports, Science and Technology (MEXT), Japan. The authors are grateful to Dr S. Nakao from the Institute for Molecular Science (IMS) for technical support of this research. This work was supported by Grant-in-Aid for Scientific Research (C) [grant number 16K04961] from the Japan Society for the Promotion of Science (JSPS). The authors would like to thank Enago (www.enago.jp) for the English language review.
Disclosure statement
No potential conflict of interest was reported by the authors.
References
- Allan NL, Dayer MJ, Kulp DT, et al. Atomistic lattice simulations of the ternary fluorides AMF3 (A=Li, Na, K, Rb, Cs; M= Mg, Ca, Sr, Ba). J Mater Chem. 1991;1:1035–1039.10.1039/jm9910101035
- Meijerink A. Spectroscopy and vibronic transitions of divalent europium in LiBaF3. J Lumin. 1993;55:125–138.10.1016/0022-2313(93)90033-J
- Jasons JL, Krumins VJ, Rachko ZA, et al. Crossluminescence of KF and related compounds. Solid State Comm. 1988;67:183–185.10.1016/0038-1098(88)90960-X
- Makhov VN, Khaidukov NM. Cross-luminescence peculiarities of complex KF-based fluorides. Nucl Instrum Methods Pys Res A. 1991;308:205–207.10.1016/0168-9002(91)90627-3
- Cao M, Wang Y, Qi Y, et al. Synthesis and characterization of MgF2 and KMgF3 nanorods. J Solid State Chem. 2004;177:2205–2209.10.1016/j.jssc.2004.01.005
- Kobayashi T, Mroczkowski S, Owen JF. Fluorescence lifetime and quantum efficiency for 5d -> 4f transitions in Eu2+ doped chloride and fluoride crystals. J Lumin. 1980;21:247–257.10.1016/0022-2313(80)90004-6
- Sahnoun M, Zbiri M, Daul C, et al. Mater Chem Phys. 2005;91:185–191.10.1016/j.matchemphys.2004.11.019
- Komar VK, Gektin AV, Ivanov NP, et al. Growth and study of properties of pure and rare-earth-doped KMgF3 crystals. J Cryst Growth. 1996;166:419–422.10.1016/0022-0248(96)00095-4
- Horsch G, Paus HJ. A new color center laser on the basis of lead-doped KMgF3. Opt Comm. 1986;60:69–73.10.1016/0030-4018(86)90119-7
- Masson NJML, Vink AP, Dorenbos P, et al. Ce3+ and Pr3+ 5d-energy levels in the (pseudo) perovskites KMgF3 and NaMgF3. J Lumin. 2003;101:175–183.10.1016/S0022-2313(02)00411-8
- Yanagihara M, Yusop MZ, Tanemura M, et al. Vacuum ultraviolet field emission lamp utilizing KMgF3 thin film phosphor. APL Mater. 2014;2:046110.10.1063/1.4871915
- Ledoux G, Guillois O, Porterat D, et al. Photoluminescence properties of silicon nanocrystals as a function of their size. Phys Rev B. 2000;62:15942.10.1103/PhysRevB.62.15942
- Norris DJ, Efros AL, Rosen M, et al. Size dependence of exciton fine structure in CdSe quantum dots. Phys Rev B. 1996;53:16347.10.1103/PhysRevB.53.16347
- Zeng H, Duan G, Li Y, et al. Blue luminescence of ZnO nanoparticles based on non-equilibrium processes: defect origins and emission controls. Adv Funct Mater. 2010;20:561–572.10.1002/adfm.v20:4
- Bhargava RN, Gallagher D, Hong X, et al. Optical properties of manganese-doped nanocrystals of ZnS. Phys Rev Lett. 1994;72:416.10.1103/PhysRevLett.72.416
- Kompe K, Borchert H, Storz J, et al. Green-emitting CePO4:Tb/LaPO4 core-shell nanoparticles with 70% photoluminescence quantum yield. Angew Chem Int Ed. 2003;42:5513–5516.10.1002/(ISSN)1521-3773
- Seo WS, Jo HH, Lee K, et al. Preparation and optical properties of highly crystalline, colloidal, and size-controlled indium oxide nanoparticles. Adv Mater. 2003;15:795–797.10.1002/adma.200304568
- Yang S, Kiraly B, Wang WY, et al. Fabrication and characterization of beaded SiC quantum rings with anomalous red spectral shift. Adv Mater. 2012;24:5598–5603.10.1002/adma.201202286
- Fan JY, Wu XL, Chu PK. Chu. Low-dimensional SiC nanostructures: fabrication, luminescence, and electrical properties. Prog Mater Sci. 2006;51:983–1031.10.1016/j.pmatsci.2006.02.001
- Efros AL, Efros AL. Interband absorption of light in a semiconductor sphere. Sov Phys Semicond. 1982;16:772–775.
- Brus LE. A simple model for the ionization potential, electron affinity, and aqueous redox potentials of small semiconductor crystallites. J Chem Phys. 1983;79:5566.10.1063/1.445676
- Yang S, WLiB Cao, Zeng H., et al. Origin of blue emission from silicon nanoparticles: direct transition and interface recombination. J Phys Chem C. 2011;115:21056–21062.10.1021/jp2075836
- Walters RJ, Kalkman J, Polman A, et al. Photoluminescence quantum efficiency of dense silicon nanocrystal ensembles in SiO2. Phys Rev B. 2006;73:132302.10.1103/PhysRevB.73.132302
- Jurbergs D, Rogojina E, Mangolini L, et al. Silicon nanocrystals with ensemble quantum yield exceeding 60%. Appl Phys Lett. 2006;88:233116.10.1063/1.2210788
- Brus L. Electronic wave functions in semiconductor clusters: experiment and theory. J Phys Chem. 1986;90:2555–2560.10.1021/j100403a003
- Wang Y, Herron N. Nanometer-sized semiconductor clusters; materials synthesis, quantum size effects, and photophysical properties. J Phys Chem. 1991;95:525–532.10.1021/j100155a009
- Wang F, Han Y, Lim CS, et al. Simultaneous phase and size control of upconversion nanocrystals through lanthanide doping. Nature. 2010;463:1061–1065.10.1038/nature08777
- Stouwdam JW, Veggel FCJM. Near-infrared emission of redispersible Er3+, Nd3+, and Ho3+ doped LaF3 nanoparticles. Nano Lett. 2002;2:733–737.10.1021/nl025562q
- Bender CM, Burlitch JM, Barber D, et al. Synthesis and fluorescence of neodymium-doped barium fluoride nanoparticles. Chem Mater. 2000;12:1969–1976.10.1021/cm9904741
- Nandiyanto ABD, Iskandar F, Ogi T, et al. Nanometer to submicrometer magnesium fluoride particles with controllable morphology. Langmuir. 2010;26:12260–12266.10.1021/la101194w
- Quan Z, Yang P, Li C, et al. Shape and phase-controlled synthesis of KMgF3 colloidal nanocrystals via microwave irradiation. J Phys Chem C. 2009;113:4018–4025.10.1021/jp810714k
- Yang GW. Laser ablation in liquids: applications in the synthesis of nanocrystals. Prog Mater Sci. 2007;52:648–698.10.1016/j.pmatsci.2006.10.016
- Sakiyama K, Koga K, Seto T, et al. Formation of size-selected Ni/NiO core-shell particles by pulsed laser ablation. J Phys Chem B. 2004;108:523–529.10.1021/jp035339x
- Sasaki T, Terauchi S, Koshizaki N, et al. Preparation of nanoparticles by excimer laser ablation of calcium iron complex oxide. Ceram Process. 1997;43:2636.
- Koch J, Bohlen A, Hergenroder R, et al. Particle size distributions and compositions of aerosols produced by near-IR femto- and nanosecond laser ablation of brass. J Anal At Spectrom. 2004;19:267–272.10.1039/B310512A
- Dinh LN, Hayes SE, Wynne AE, et al. Properties of GaAs nanoclusters deposited by a femtosecond laser. J Mater Sci. 2002;37:3953–3958.10.1023/A:1019680111363
- Yoshida T, Takeyama S, Yamada Y, et al. Nanometer-sized silicon crystallites prepared by excimer laser ablation in constant pressure inert gas. Appl Phys Lett. 1996;68:1772.10.1063/1.116662
- Nichos WT, Malyavanatham G, Henneke DE, et al. Gas and pressure dependence for the mean size of nanoparticles produced by laser ablation of flowing aerosols. J Nanopart Res. 2000;2:141–145.10.1023/A:1010014004508
- Tull BR, Carey JE, Sheehy MA, et al. Formation of silicon nanoparticles and web-like aggregates by femtosecond laser ablation in a background gas. Appl Phys A. 2006;83:341–346.10.1007/s00339-006-3502-7
- Glover TE. Hydrodynamics of particle formation following femtosecond laser ablation. J Opt Soc Am B. 2003;20:125–131.10.1364/JOSAB.20.000125
- Amoruso S, Bruzzese R, Spinelli N, et al. Generation of silicon nanoparticles via femtosecond laser ablation in vacuum. Appl Phys Lett. 2004;84:4502.10.1063/1.1757014
- Bulgakov AV, Ozerov I, Marine W. Silicon clusters produced by femtosecond laser ablation: non-thermal emission and gas-phase condensation. Appl Phys A. 2004;79:1591–1594.10.1007/s00339-004-2856-y
- Glover TE, Ackerman GD, Lee RW, et al. Probing particle synthesis during femtosecond laser ablation: initial phase transition kinetics. Appl Phys B. 2004;78:995–1000.10.1007/s00340-004-1449-y
- Koshizaki N, Narazaki A, Sasaki T. Size distribution and growth mechanism of Co3O4 nanoparticles fabricated by pulsed laser deposition. Scripta Mater. 2001;44:1925–1928.10.1016/S1359-6462(01)00811-9
- Werwa E, Seraphin AA, Chiu LA, et al. Synthesis and processing of silicon nanocrystallites using a pulsed laser ablation supersonic expansion method. Appl Phys Lett. 1994;64:1821.10.1063/1.111766
- Goldys EM, Tomsia KD, Jinjun S, et al. Optical characterization of Eu-doped and undoped Gd2O3 nanoparticles synthesized by the hydrogen flame pyrolysis method. J Am Chem Soc. 2006;128:14498–14505.10.1021/ja0621602
- Hakkinen H, Moseler M, Kostko O, et al. Symmetry and electronic structure of noble-metal nanoparticles and the role of relativity. Phys Rev Lett. 2004;93:093401.10.1103/PhysRevLett.93.093401
- Zhou H, Cai W, Zhang L. Photoluminescence of indium-oxide nanoparticles dispersed within pores of mesoporous silica. Appl Phys Lett. 1999;75:495.10.1063/1.124427