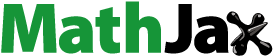
ABSTRACT
In recent years, halide perovskite quantum dots (HP-QDs) based composites have been widely developed and used in various applications owing to their unique photonic, electronic and mechanical properties, as well as high stability to water, oxygen, heat and illumination. Remarkable efforts have been made in the synthesis and applications of these materials in photonics, electronics, sensors and other fields. Besides these topics, we also cover enhancement of optoelectronic properties as well as chemical, thermal and photostability of HP-QDs-based composites. We hope this review will promote both the development and applications of perovskite-based materials.
GRAPHICAL ABSTRACT

CLASSIFICATION:
1. Introduction
With the rapid development of material science, more and more low-dimension materials, such as nanosheets, nanowires and nanodots, are being developed and applied in various fields due to their unique optical, electrical, mechanical, thermal and other properties [1–11]. Quantum dots (QDs), which are a zero-dimensional (0D) material, have attracted increasing attention as next-generation functional materials. They are widely used for optoelectronic devices including light-emitting diodes [Citation12–Citation14], lasers [Citation15], solar cells [Citation16–Citation18], photodetectors [Citation19] and many other applications including drug delivery, chemical analysis, sensor, bioimaging [Citation20–Citation23]. Due to their quantum confinement effect that appears when the semiconductor materials’ size is smaller than twice its Bohr radius, QDs process excitonic features and enhanced photoluminescence (PL) properties comparing to bulk materials [Citation24–Citation26]. Various types of QDs such as CdS [Citation27], CdSe [Citation13,Citation15,Citation28], ZnS [Citation29], PbS [Citation30], PbSe [Citation31], SnS [Citation32,Citation33], SnSe [Citation34] silicon [Citation35] and halide perovskites have been explored and applied in various applications.
Halide perovskite quantum dots (HP-QDs), with chemical formula of ABX3 (A = CH3NH3, CH5N2, Cs; B = Pb, Sn; C = I, Br, Cl), have emerged rapidly as a new outstanding class of QDs materials for their narrower full width at half maximum (FWHW), defect-tolerant structure and high synthesis feasibility comparing to those traditional classes of QDs [Citation36–Citation38]. Owing to their merits, including tunable bandgap, high light-absorption efficiency, low carrier recombination rate, high defect tolerance and high PL quantum yield, HP-QDs have been applied in various electronic and optoelectronic applications such as photoemission [Citation39–Citation41], photovoltaic [Citation42], photodetectors [Citation43], photocatalysts [Citation44] and memristors [Citation45]. For example, Dai et al. had reported HP-QDs exhibiting maximum PL quantum yield up to 100%, showing promising potential for photo-emission [Citation46]. Moreover, the easy and low-cost synthesis earned HP-QDs additional attractions. Via some simple methods such as one-step hot-injection and an even easier ligand-assisted recrystallization at room temperature, ideal HP-QDs with uniform morphology, tunable emission and other superior performance can be obtained [Citation47].
While exhibiting excellent optical properties, the as-obtained HP-QDs suffer from poor chemical, thermal and photostability. Their structure degrades in an atmosphere containing oxygen and water due to photooxidation [Citation48–Citation50]. With an ionic structure and highly dynamic ligand bonding, the photoexcited HP-QDs release electrons that would easily interact with oxygen molecules. The free radicals generated would then react with the amine salt, leading to the decomposition [Citation51]. HP-QDs are also extreme sensitive to many other environmental factors like high temperature and UV light [Citation48,Citation52]. Therefore, improving the environmental stability is always one of the hot topics in the studies of HP-QDs.
To enhance the stability of HP-QDs, various strategies such as shell design, ligand design and overcoating [Citation48] have been explored, of which compositing HP-QDs with other materials to passivate its surface and form a protection layer or heterojunction is a practical and promising one. Various materials including oxides, polymers, metallic ions, and many other organic and inorganic options have been explored in recent years. By diverse structures such as shelling the QDs at single-particle level, encapsulating the QDs into vast matrix, loading QDs onto the surface, ion-doping in the lattice of HP-QDs or forming HP-QDs/QDs nanocomposites, excellent results for HP-QDs based composites have been reported, showing outstanding improvement in stability and some other expected properties for enhanced performance in photonics, electronics, sensors and other fields (as shown in ) [Citation53,Citation54]. The two fields of material synthesis methods and material applications can promote the developments of each other. Therefore, it is of great necessity to make a periodic summary in this rapid development of scientific research era. Herein, we systematically discussed the recent development of common fabrication strategies and performances of HP-QDs based composites, and their application in photonics, electronics and sensors.
Scheme 1. Schematic illustration of the structures and applications of HP-QDs composites discussed in this review. Produced with permission from [Citation69,Citation77]. © 2018 ACS Publications [Citation105,Citation117];. © 2017 Royal Society of Chemistry [Citation116,Citation121]; © 2017 ACS publications [Citation134]; © 2018 ScienceDirect [Citation122,Citation177]; © 2018 ScienceDirect.
![Scheme 1. Schematic illustration of the structures and applications of HP-QDs composites discussed in this review. Produced with permission from [Citation69,Citation77]. © 2018 ACS Publications [Citation105,Citation117];. © 2017 Royal Society of Chemistry [Citation116,Citation121]; © 2017 ACS publications [Citation134]; © 2018 ScienceDirect [Citation122,Citation177]; © 2018 ScienceDirect.](/cms/asset/0aadf15e-f984-4b32-b3aa-192ab8a6b1ec/tsta_a_1752115_sch0001_oc.jpg)
2. Fabrication strategies
2.1. Fabrication strategies of halide perovskite quantum dots
The fabrication strategies of HP-QDs can be summarized into two kinds: 1) high-temperature hot-injection (HI) method, which needs serious reaction environment including certain high temperature and protective gas, 2) room temperature (RT) synthesis method, a relatively easy-to-operate and low-cost method [Citation47].
A typical HI synthesis method involves the preparation of required precursor by heating the mixture to a certain temperature under gas protection, and a quick injection into another solution. Via the mechanism of a quick ionic metathesis reaction, the desirable HP-QDs with excellent monodispersity and optical properties can be obtained [Citation55]. Using this method, the nucleation stage happened right after the injection to form nuclei and the growth stage started after its termination, where this separation between two stages allows achieving a narrow size distribution of the nanoparticles [Citation56]. The high reaction temperature also enables better control over the QDs shape and better phase purity [Citation57,Citation58]. CsPbBr3 QDs and some other HP-QDs can be obtained using this method [Citation55]. The adjustment of the operating temperature in this process plays an important role of helping control the size of the obtained QDs and would also influence the PL peak position [Citation59,Citation60].
Being less complex, RT synthesis method employs ‘good’ solvent to form the precursor without heating. Then, long-chain organic ligands and ‘poor’ solvent like toluene are mixed with the former solution under intense stirring to facilitate the formation of HP-QDs through recrystallization [Citation61–Citation64]. In this process, the nucleation and growth were started by the instantaneous supersaturation without a separation in timeline as in HI synthesis [Citation56]. Being more convenient for large-scale production though, RT synthesis also shows some drawbacks like limited control of QDs shape and possible dissolving of the produced QDs due to the existence of polar solvents [Citation65–Citation68].
2.2. Structures of HP-QDs based composites and their fabrication strategies
The HP-QDs-based composites can be mainly divided into core-shell structure, HP-QDs/matrix structure, ion doping and HP-QDs/QDs structure. Here, the fabrication strategies of these four kinds of HP-QDs based composites are discussed separately (as shown in ). It is worth noting that the difference between ‘core-shell structure’ and ‘HP-QDs/matrix structure’ is the number of HP-QDs. In ‘core-shell structure’, there are one or few HP-QDs are defined as ‘core’. However, there are large amounts of HP-QDs in the matrix in the structure of ‘HP-QDs/matrix’.
2.2.1. Core-shell structure
Shell design is considered as a promising way to protect sensitive materials from degradation caused by environmental factors. By covering the QDs with a sturdy and inert shell, not only can the formed shell insulate the QDs from oxygen or water molecules but also this outside layer can passivate the surface of the HP-QDs by decreasing its high surface energy and increasing the energy barrier [Citation53]. Oxide materials have been successfully applied as the shell material to form exquisite structure with great monodispersity. Here, taking examples of the most commonly used shell materials SiO2 [Citation69] and TiO2 [Citation70], several kinds of modulated sol-gel methods for the formation of the shell are discussed.
SiO2 has been utilized as shell material for QDs like CdSe [Citation71] for a long history since 2006 due to its robustness and chemical stability. Commonly, as shell material, SiO2 was synthesized by direct hydrolysis method in QD solutions of water and ethanol using tetraethyl orthosilicate (TEOS, SiOC2H5) as silica precursor [Citation72]. This method is called sol-gel method [Citation73], and its chemical process could be divided into three steps [Citation74]:
However, this traditional method is not completely suitable for HP-QDs due to the presence of water as media. To form a water-less reaction system, various kinds of modulated sol-gel methods have been carried out. In 2017, Hu et al. used 2-methoxyethanol to replace water molecules. In this work, the functional –OH group of 2-methoxyethanol helped to transfer TEOS to Si-O bonds and also passivate the surface of CsPbBr3 QDs to improve its water-resistance [Citation74]. Another group led by Huang adopted orthosilicate (TMOS, Si(OCH3)4) as silica precursor and toluene as reactive solvent with a water content of only 0.0184% to encapsulate MAPbBr3-QDs (MA: CH3NH3) [Citation75]. In this process, the hydrolysis rate is relatively fast (4 h) for the faster water consumption rate of TMOS [Citation75]. Cai et al. instead utilized a heating treatment in the open air to attract water molecules from the air to initiate the hydrolysis. Adding the capping agents in the waterless solution before the synthesis of HP-QDs resulted in a better control of the QD size [Citation76].
Figure 1. Schematic illustration of the synthesis strategies of (a) CsPbBr3 QD core/SiO2 shell structure. (b) CsPbBr3 QD core/TiO2 shell structure; transmission electron microscopy (TEM) images of (c) CsPbBr3 QDs (d) CsPbBr3 QD/TiO2 composites at single particle level. Produced with permission from [Citation69]. © 2018 ACS Publications, and [Citation70]. © 2018 Wiley.
![Figure 1. Schematic illustration of the synthesis strategies of (a) CsPbBr3 QD core/SiO2 shell structure. (b) CsPbBr3 QD core/TiO2 shell structure; transmission electron microscopy (TEM) images of (c) CsPbBr3 QDs (d) CsPbBr3 QD/TiO2 composites at single particle level. Produced with permission from [Citation69]. © 2018 ACS Publications, and [Citation70]. © 2018 Wiley.](/cms/asset/92767e62-7617-4621-b15f-669962f61790/tsta_a_1752115_f0001_oc.jpg)
In 2018, a modulated one-pot approach for HP-QDs/SiO2 composite at single-particle level was realized in a report by Zhong et al. using TMOS [Citation69]. Both the perovskite QDs and the outer silica shell were synthesized in one-pot process at room temperature. In this process, at 30°C, the mixture of perovskite precursor including CsBr, PbBr2, oleic acid and oleylamine dissolved in dimethylformamide (DMF) was quickly injected into toluene solution of tetramethoxysilane (TMOS). The perovskite QDs were obtained immediately by recrystallization. Then, after two more hours under magnetic stirring, the CsPbBr3 QDs core (with average size of 10.5 nm)/SiO2 shell (with average thickness around 7.7 nm) structure was prepared (). In the presence of oleic acid and oleylamine, the firstly synthesized QDs could be well dispersed uniformly within the solution, while the silica oligomers produced by TMOS were gradually attracted to the QDs to form the shell [Citation69]. Their group has also successfully synthesized a CsPbBr3 QDs/SiO2 Janus structure earlier in 2018 via a quick injection of deionized (DI) water into the mixture of TMOS and Cs4PbBr6 within hexane solvent [Citation77]. In that process, the growth of SiO2 happened at the interface of hexane and water, and the Cs4PbBr6 was transformed to CsPbX3 QDs.
Another modulated sol-gel method, called hydrolysis-calcination method was successfully used for synthesizing HP-QDs/TiO2 core/shell structure with outstanding monodispersity by Li’s group [Citation70]. The HI synthesized colloidal CsPbBr3 QDs kept in toluene solution at 25°C was added dropwise by the prepared titanium dioxide precursor titanium butoxide (TBOT) under stirring within 30% humidity, allowing the hydrolysis reaction to happen and form a complex compound of titanium (as shown in ). At this step, large area of TiOx matrix around the QDs was observed. Then, the solution system was heated to 300°C for calcination and removal of water, yielding well-dispersed core/shell structures with a high abundance of single-particle-core and about 5 nm TiO2 shell [Citation70].
Thanks to the inert shell formed by oxides, the HP-QDs were fully coated with few oxygen or water molecule getting though, achieving long-tern stability against air. And the surface of QDs was passivated to reach much better water-resistance. Placing both the HP-QDs/SiO2 composites and pure HP-QDs within an environment of 75% humidity and exposed to air, the X-ray diffraction (XRD) peak of the composite only decreased slightly after 4 weeks while the pattern of pure QDs has been totally distorted within 3 days [Citation69]. The CsPbBr3/TiO2 composite showed even better stability against water for maintaining great optical properties after 3 months within it, and even the size and morphology of the composite kept unchanged along this time. The stability against UV light was also tested, 75% PL intensity of the original value was kept after 24 h. Besides the outstanding enhancement in stability, the exquisite core/shell structure could help reduce anion-exchange effect to some extent since the QDs were insulated from each other, and TiO2 shell was also verified to promote charge carrier transfer [Citation70].
2.2.2. HP-QDs/matrix structure
2.2.2.1. Encapsulated into material matrix
Without extreme high requirement for monodispersity, encapsulating a vast of HP-QDs into matrix of the coating materials is considered a more simple and efficient strategy to insulate and protect the HP-QDs, and thus is a more widely adopted method. Common materials utilized to encapsulate HP-QDs contain polymers, oxides, metallic compounds like CaF2 [Citation78], ionic compounds like NaNO3 [Citation79] and other organic materials like carboxybenzene [Citation80]. And the synthesis strategies here are divided into template-free methods and template methods according to whether a template is adopted.
2.2.2.1.1. Template-free methods
Sol-gel method. Sol-gel method is one kind of template-free method that is commonly used for synthesizing HP-QDs/Oxide matrix composites. Usually, the process could be divided into two parts: fabricate HP-QDs first, and then inject the oxide precursor into the HP-QDs solution system. After the mixing, the pre-synthesized HP-QDs and the precursor, which is the resource of oxide molecules, got in touch. Then, the precursor molecules went through a hydrolysis process to form oxide matrix around the QDs as protection. Similar to the synthesis of shell materials discussed before, the choices of precursor reagent and medium of the solution system are the key factors to the properties of resulted HP-QDs/SiO2 composites.
For silicon oxide, Sun et al. [Citation74] applied (3-aminopropyl)triethoxysilane (APTES) to an organic cross-linked Si-O-Si silica matrix. In this work, non-aqueous solvent octa-decene (ODE) was used to mix the APTES and HP-QDs for a waterless system while the freely dispersed QDs were capped by the whole APTES molecules as a shell, indicating that the formation of silica matrix did not begin. Then, the solution system was exposed to the ambient air to allow the water molecules to be caught and initiate the hydrolysis process. As shown in , APTES here played important roles since not only it was hydrolysed to generate the Si-O-Si cross-linked silica structures over the QDs but also the surface of the QDs was passivated by its amino group to maintain the original optical and photoelectronic properties well [Citation74].
Figure 2. (a) Schematic illustration of synthesis strategy of QD/silica composites. (b) The simplified synthesis of CsPbBr3/SiO2 composite; PL intensity of CsPbBr3/SiO2 composite under (c-d) heat, (e) 365 nm UV light (f) xenon lamp irradiation. Produced with permission from [Citation74]. © 2016 Wiley, and [Citation82]. © 2019 ScienceDirect.
![Figure 2. (a) Schematic illustration of synthesis strategy of QD/silica composites. (b) The simplified synthesis of CsPbBr3/SiO2 composite; PL intensity of CsPbBr3/SiO2 composite under (c-d) heat, (e) 365 nm UV light (f) xenon lamp irradiation. Produced with permission from [Citation74]. © 2016 Wiley, and [Citation82]. © 2019 ScienceDirect.](/cms/asset/03625760-23ab-4cb6-a54c-62c04438ba01/tsta_a_1752115_f0002_oc.jpg)
On the basis of this APTES mechanism, in 2017, Sun’s group further developed their Si-O-Si network strategy and successfully embedded CsPbX3 QDs into organic silica gel matrix via a HI of APTES and perovskite precursor Cs-oleate by turns for excellent flexibility, transparecy and enhanced stability. And since the organic silica matrix was chemically bonded to the QDs, this composite structure could be well maintained without shrinking [Citation81]. On the other path, in 2019, Cao et al. further simplified the traditional APTES method to realize that no complex operation other than stirring was needed during the whole process (). The as obtained CsPbBr3/organic silica matrix composite was then observed to exhibit enhanced photo- and chemical stability for a limited decrease of 5.7% and 1.4% in PL intensity after 30 days in air and 96 h under UV light () [Citation82].
Besides APTES for organic silica matrix, perhydropolysilazane (PHPS) [Citation83] as precursor to form dense inorganic silica matrix with enhanced hardness and chemical stability, zirconium n-propoxide as precursor for ZrO2 matrix [Citation84], Al-Si precursor for SiO2/Al2O3 binary matrix [Citation85], polysilazane for SiNx/SiNxOy/SiOy matrix structure [Citation86] were also reported using similar sol-gel method.
Atomic layer deposition. Atomic layer deposition (ALD) was adopted to encapsulate QDs by AlOx in 2017 [Citation87]. ALD is a technique that can deposit materials layer by layer at single-atom level on the surface of the substrate [Citation88]. The application of ALD on HP-QDs was firstly reported by Loiudice et al. They spin-coated CsPbX3 QD on a substrate and then deposited an amorphous alumina shell around the whole CsPbX3 QD layer. Optimal parameters of ALD were studied and the composites exhibited excellent stability against water and heat [Citation87]. Metal oxides like AlOx are also good candidates as encapsulating materials for their transparency and outstanding performance in protecting QDs from oxidative and other chemical factors due to their low ion diffusion rate. With this protection layer, the as prepared CsPbX3 QDs/AlOx composites exhibited great stability in air (for 45 days), under irradiation (8 h), heat (200°C) and water (1 h after being immersed) [Citation87].
Crystallization method. Crystallization method is commonly adopted for encapsulating HP-QDs in polymer matrix where the precursor of HP-QDs were added into the matrix. The precursor capped by the polymer structures would form QDs inside the matrix via crystallization process. Based on various specific operation methods, it could be further divided into simple blending [Citation89,Citation90], separate crystallization [Citation91], swelling-deswelling [Citation92] and melting-quenching with subsequent heat-treatment [Citation24].
Early in 2015, Li et al. reported crystallization of organic halide perovskite quantum dots (OHP-QDs) inside polymer matrix via a simple and direct blending of perovskite precursor and polymer matrix followed by annealing. The composite structure MAPbBr3-QDs/4,4-bis(N-carbazolyl)-1,1-biphenyl (CBP) was successfully obtained [Citation89]. Another group of Li et al. employed polyimide precursor dielectric (PIP) and obtained the PIP/MAPbBr3 QDs composites by so too. The as obtained composite thin film was pinhole-free and exhibited enhanced quantum efficiency [Citation90]. However, the dispersity of QDs inside the polymer was relatively low by physical blending only. To enhance the dispersity, two developed strategies of crystallization called separate crystallization and swelling-deswelling were carried out.
Figure 3. (a) Schematic illustration of MAPbBr3-QDs/PVDF synthesis via separate crystallization: Stage Ⅰ: mixture poured on the substrate; Stage II: evaporation of DMF; Stage III: removing the residual DMF. (b) Schematic illustration of the formation via Swelling-deswelling, and (c) via porous polymer template. Reproduced with permission from [Citation91,Citation92]. © 2016 Wiley, and [Citation105]. © 2017 Royal Society of Chemistry.
![Figure 3. (a) Schematic illustration of MAPbBr3-QDs/PVDF synthesis via separate crystallization: Stage Ⅰ: mixture poured on the substrate; Stage II: evaporation of DMF; Stage III: removing the residual DMF. (b) Schematic illustration of the formation via Swelling-deswelling, and (c) via porous polymer template. Reproduced with permission from [Citation91,Citation92]. © 2016 Wiley, and [Citation105]. © 2017 Royal Society of Chemistry.](/cms/asset/6bb7bb60-3c0a-4d3d-a7b4-8229cb53293b/tsta_a_1752115_f0003_oc.jpg)
Separate crystallization strategy () was firstly presented by Zhou et al. in 2016 [Citation91]. In their report, the precursor of MAPbBr3-QDs and precursor of polyvinylidene fluoride (PVDF) were all dissolved in DMF solvent uniformly. With the removing of DMF by vacuum pumping, PVDF crystals were formed first. As the concentration exceeded the limit, MAPbBr3 would finally be crystallized under the confinement of existed polymer matrix. The interactions between –CF2– group of the PVDF and MA+ component of MAPbBr3-QDs was also verified to play an important role of helping obtain uniform size and distribution during the crystallization of MAPbBr3 [Citation91]. Similarly, by pouring the DMF solution of perovskite precursor and NaNO3 into poor solvent toluene, HP-QDs/NaNO3 composites could also be obtained via crystallization method [Citation79].
Swelling-deswelling is another mechanism to obtain great monodispersity of HP-QDs inside the polymer matrix (). In 2016, Wang et al. reported this method and tried it on various polymer materials including commonly used polystyrene (PS), polycarbonate (PC), poly(methyl methacrylate) (PMMA) and many other polymers that swell in DMF, such as acrylonitrile butadiene styrene (ABS), cellulose acetate (CA) and polyvinyl chloride (PVC) [Citation92]. Swelling occurred when the specific polymer was in the DMF solvent, the chains of polymer swelled and expanded and allow the perovskite precursors to be carried inside it uniformly. As the DMF solvent is gradually removed, nanosized HP-QDs were formed followed by the deswelling process where the polymer chains would shrink back to coherently encapsulate the HP-QDs inside. The as obtained MAPbBr3 QDs/polymer composites processed enhanced dispersion and stability against water and heat [Citation92].
Melting-quenching with subsequent heat-treatment is always utilized for inorganic polymers including phospho-silicate glasses [Citation24,Citation93], tellurite-based glasses [Citation94], borosilicate glasses [Citation80,Citation95] and boro-germanate glasses [Citation80,Citation96]. In this process, the properly designed glass matrix and the precursors of perovskite were firstly prepared and mixed together as powders. After melting at a high temperature, the precursors of perovskite were uniformly encapsulated by molten glass, and then went through self-crystallization to form HP-QDs/glass composites under a heat-treatment at a relatively low temperature [Citation24]. The resulting HP-QDs/polymer composites exhibited excellent stability and preserved 100% of their PL quantum for 30 days in air and 85% PL quantum yield after 10 days in water [Citation97].
Electrospinning method. Electrospinning method, a simple and low-cost technique, where fibers could be obtained from polymer solution under a strong electronic field, was also used to fabricate HP-QDs/polymer composites. In 2016, Wang et al. synthesize CsPbX3 QDs/PS composite in a fiber membrane structure using this method. The electrospinning solution was prepared by dissolving PS and CsPbX3 QDs in the toluene solvent added by conductivity-assisted DMF. The result showed that CsPbX3 QDs distributed inside the PS fiber with outstanding dispersion, remained optical properties and enhanced stability to water and UV light [Citation98]. Moreover, instead of using pre-synthesized HP-QDs solution, Liao et al. slightly adjusted this strategy by blending solution of perovskite precursors with the polymer as the electrospinning solution, and realized tunable optical properties by controlling the composition of the perovskite precursor [Citation99].
Monomer-polymerization method. Forming HP-QD/monomer structure first and then polymerizing the monomers to obtain polymer matrix is another strategy. In 2018, Xin et al. adopted this technique using monomers to fabricate HP-QDs/polymer composites with high stability and flexibility. They transported perovskite precursor solution into bulk monomers of styrene, then UV-light or thermal process was carried out for its polymerization [Citation100]. Similarly, other polymer materials, such as epoxy resin also can be used to develop HP-QDs/polymer composites using this monomer-polymerization method [Citation97].
2.2.2.1.2. Template methods
The template method here is a method that precursors of perovskite were always added into the pre-synthesized mesoporous template, resulting in confined growth of HP-QDs within the holes wrapped by the matrix. This template structure is also expected to hinder interactions among different kinds of HP-QDs, thereby inhibiting anion exchange on optical properties. Thus, various mechanism of carrying perovskite precursors uniformly inside the pores were reported to realize a better monodispersity including physical stirring [Citation101], capillary force [Citation102] and recrystallization [Citation103].
Mesoporous silica powder (MSP) was always used as template matrix, here as an example to state these mechanisms. In 2016, simple physical stirring () was used in the report by Wang et al. They mixed the MSP and precursor of inorganic CsPbX3 QDs in non-polar solvent hexane followed by continuous stirring and by so obtained the silica-wrapped CsPbX3 QDs structure with various pore size [Citation101]. Another team of Malgras et al. dissolved the precursor of organic MAPbBrxI3-x QDs in N,N-dimethylformamide (DMF) and added it dropwise into the dried MSP, utilizing capillary force from the difference in surface tension between DMF and silica to bring HP-QDs precursor into the pores uniformly (). The composites exhibited excellent thermal and photostability [Citation102]. Recrystallization mechanism was later adopted by Zhao et al. in 2018 using magnesium silicate hollow spheres (MSHS) as the template [Citation103]. In their work, a MAPbX3 QDs/MSHS composite () was synthesized by dropping perovskite precursor which was dissolved in good solvent DMF into toluene solution of MSHS. Tunability of emission from blue to red and outstanding thermal and photostability were verified [Citation103].
Figure 4. (a) Schematic illustration of HP-QDs/MSP synthesis via physical stirring. (b-c) TEM images of HP-QDs/MSP obtained via capillary force. (d-e) TEM images of HP-QDs/MSP obtained via recrystallization. Reproduced with permission from [Citation101]. © 2016 Wiley, and [Citation102]. ©2016 ACS publication, and [Citation103]. © 2018 ACS publication.
![Figure 4. (a) Schematic illustration of HP-QDs/MSP synthesis via physical stirring. (b-c) TEM images of HP-QDs/MSP obtained via capillary force. (d-e) TEM images of HP-QDs/MSP obtained via recrystallization. Reproduced with permission from [Citation101]. © 2016 Wiley, and [Citation102]. ©2016 ACS publication, and [Citation103]. © 2018 ACS publication.](/cms/asset/b7f9005e-a0ad-40d7-99b8-ff7e67cda097/tsta_a_1752115_f0004_oc.jpg)
Immersion is a more simple and convenient method for templates other than powders where the well-designed template was directly immersed into the solution of perovskite precursor. In 2017, Demchyshyn et al. chose nanoporous alumina scaffold as template to directly synthesize HP-QDs within specific format [Citation104]. In their work, firstly the mesoporous aluminum oxide nanotubes were prepared via evaporating aluminum on glass substrate, followed by anodizing with specific voltage to form nanosized pores. After cleaning processes, the alumina nanotubes were infiltrated into perovskite precursor solution to confine the growth of HP-QDs carried inside the pores [Citation104]. Similarly, porous polymer material obtained by preparing polydimethylsiloxane (PDMS) film with many size-controllable gold nanoparticles (AuNPs) inside and then remove the AuNPs [Citation105] has also been reported to obtain composites with HP-QDs via immersion method ().
Using template-methods to form HP-QDs-based composites could help control the emission wavelength of HP-QDs and enhance their stability at the same time [Citation106]. Compared with simple stirring method and recrystallization method that lead to random distribution of QDs, capillary force method or immersion method with well-designed templates could firstly better encapsulate the ordered HP-QDs inside the matrix for enhanced stability, and secondly improve the monodispersity of HP-QDs within the pores. The monodispersity could contribute to better prevent on anion-exchange effect of MAPbX3 with tunable ratio of the X components for tunable emission peak. It was verified that the obtained great tunable properties of HP-QDs also benefits from the controllable QDs size by the controlled pore size of the templates, since not only did the template act as isolating layer, it also help confine the growth of QDs to reduce its structural disorder [Citation102].
Besides these strategies, CsPbBr3 QDs/ethylene vinyl acetate (EVA) composite with long-term stability and great flexibility was obtained by Li et al. via one-pot RT-synthesis through recrystallization of CsPbBr3 QDs and dissolution of EVA [Citation107]. And for specific application, Huang’s team in 2018 and Tana et al. in 2019 adopted molecularly imprinted polymers (MIPs) for sensitive detection [Citation108,Citation109], and Xuan et al. employed hydrophobic porous polymer frameworks (SHFW) to obtain HP-QDs-based composite with extraordinary water-resistant performance [Citation110].
2.2.2.2. Loading on materials surface
Loading HP-QDs on the surface of other materials is another efficient way to form composites and usually leads to nanojunctions. In this part, synthesis methods for composite structures differ from physical contact to chemical bonding would be discussed separately.
2.2.2.2.1. Physical contact
Physical contact structure is commonly obtained via relatively direct and simple physical methods including spin-coating and physical blending. Using this method, the utilization of metal oxides including TiO2 [Citation34], Al2O3, ZrO2 and SnO2 [Citation106] were studied at 2009 and 2012 by Kojima et al. In their work, prepared porous Al2O3 paste and the perovskite precursor solution (MABr and PbBr2 in DMF) were successively spin-coated on a glass substrate. Al2O3/MAPbBr3 nanocomposites were obtained via self-organization of HP-QDs separately on the surface of the Al2O3 spheres. The ZrO2/MAPbBr3 composites, SnO2/MAPbBr3 composites, TiO2/MAPbX3 composites were obtained via a similar process. Enhanced optical properties of these heterojunctions were also reported which benefit from the greater energy band gap and ionization potential of Al2O3 and ZrO2 to intense emission [Citation106], and lower conductive level of TiO2 and SnO2 to speed up the electron injection [Citation111].
Blending Al2O3 nanocrystals and the perovskite precursor together first and then spin-coated it on the substrate, Longo et al. obtained and further studied the Al2O3/MAPbBr3 nanocomposites thin film. In this work, they stated the assistance of aluminum oxides in confining perovskite into nanoscale by comparing results with different amount of Al2O3 [Citation112].
Spin-coating pre-synthesized HP-QDs on the surface is another convenient physical method. In 2017, MAPbI3 solution was spin-coated over the TiO2 nanotubes (NTs) to obtain MAPbI3/TiO2 NTs composites by Zheng et al., and enhanced stability against moisture/heat and the improved responsivity in photodetector application area were verified [Citation113]. Lu et al. spin-coated CsPbX3 QDs on the 3D radial junction over a silica nanowire structure [Citation114]. In 2019, Zhao et al. prepared a composite structure of Ag/CsPbBr3-QDs/g-C3N4 (CN) via spin-coating, where the HP-QDs were uniformly distributed on CN layer, followed by a layer of Ag spin-coated on the above for photocatalyst [Citation115].
Besides these physical methods, one-pot synthesis for HP-QDs/nanosheet has also been reported with graphene oxide (GO) as matrix material [Citation116]. In that work, the graphene oxide and PbBr2 were blended in DMF together followed by quick injection of the perovskite precursor Cs-oleate solution. The as obtained CsPbBr3 QD/GO composite, with HP-QDs uniformly distributed on the GO sheet, exhibited great electron consumption rate and potential in photocatalysis applications [Citation116].
2.2.2.2.2. Chemical bonding
Chemical bonding structure here could be obtained via two main strategies: linker molecules and ion exchange reaction. For using linker molecules, Zhou et al. in 2017 reported a TiO2/HP-QDs composite using bifunctional linker molecule 3 mercaptopropionic acid (MPA) to improve the electron transfer rate of the nanojunction [Citation117]. TiO2 paste, pure MPA and CsPbBr3 QDs solution were spin-coated in turn on the substrate, where the thiol groups of MPA interacted with CsPbBr3 QDs with its carboxylic groups reacting with hydroxyl group of TiO2 nanoparticles. The TiO2/MPA/CsPbBr3-QDs composites were obtained with enhanced electron transfer rate.
Figure 5. (a) Schematic illustration of the a-SiO2/HP-QDs synthesis. (b-d) TEM images of the a-SiO2/HP-QDs composite. (e) Size distribution of the loaded HP-QDs. Reproduced with permission from [Citation118]. © 2017 Wiley.
![Figure 5. (a) Schematic illustration of the a-SiO2/HP-QDs synthesis. (b-d) TEM images of the a-SiO2/HP-QDs composite. (e) Size distribution of the loaded HP-QDs. Reproduced with permission from [Citation118]. © 2017 Wiley.](/cms/asset/c7e2acd4-987f-4aa5-b928-416cf2fb08d7/tsta_a_1752115_f0005_oc.jpg)
Silica oxide, as another versatile oxide material, has also been explored in this area using monodisperse aminated SiO2 (A-SiO2) spheres () [Citation118]. In this work, they blended A-SiO2 spheres, perovskite precursor, surfactant together in ODE followed by heat-treatment. During this process, amination played the important role of stimulating the adhesion of HP-QDs through chemical interaction, and the HP-QDs would grow on or attach to the spheres. These A-SiO2/HP-QDs composites were verified to exhibit outstanding stability for performing little PL degradation after 40 days, and only 20% degradation after 108 h under UV light [Citation118].
Ion-exchange reaction method was utilized for CsPbBr3@NH4Br composites by Lou et al. They added excess NH4Br into a toluene solution of CsPbCl3 nanoparticles where the CsPbCl3 would be transformed into CsPbBr3 by anion-exchange effect when getting close to the NH4Br molecules. Then, with the replacement of Cl− to Br−, the HP-QDs were chemically attached to the NH4Br and thus formed tense composites. The composites showed enhanced thermal stability than pure QDs. And the [NH4]+ group could help stabilize the colloidal structure of HP-QDs and by so improve their stability against polar solvent as water [Citation119].
2.2.3. Ion doping
Ion doping is a promising strategy in adjusting the electronic and optical properties of QDs [Citation120]. For HP-QDs, it was verified that introducing metal ions like Mn2+ [Citation121–Citation124], Sn2+ [Citation121,Citation125], Ce3+ [Citation126], Eu3+ [Citation127] into the perovskite lattice of CsPbX3 QD could help reach tunable energy gap [Citation123], enhanced thermal stability [Citation121] and improved PL efficiency [Citation126]. The fabrication strategies of ion doping contains hot-injection and melt-quenching.
Hot-injection method was used to add the precursor of ions into the PbX3 solution followed by HI of Cs precursor. In 2016, the synthesis of Mn2+:CsPbX3 QDs composites was realized by this approach using MnX2 as the ion-precursor, where tunable band gap and mechanism were studied [Citation123,Citation124]. On this basis, by slightly modifying the Mn/Pb ratio in the process, Zou et al. further studied the Mn2+:CsPbX3 QDs composites and firstly stated its enhancement in stability against high temperature and ambient air. This was attributed to the reduced ionic radius of metal ions than the replaced Pb2+ in the lattice that leads to higher formation energy which would radically improve the thermal stability of HP-QDs (). In this work, the similar applications of Cd2+, Co2+, Zn2+, Sr2+ and Sn2+ ions in doping CsPbBr3 QDs were also explored [Citation121]. Ce3+:CsPbBr3 QDs composite was synthesized by this method too in 2018, where the CeBr3 was hot-injected first into the PbX3 solution system followed by the halide precursor, exhibiting enhanced PL quantum yield [Citation126].
Figure 6. Schematic illustrations of (a) the structures of crystal lattice of perovskite CsPbX3 before and after Mn2+doping; (b) the formation of CsPbBr3 and Eu3+:CsPbBr3 QDs via melt-quenching. (c-d) TEM images of Mn2+:CsPbX3 QDs prepared via HI. (e-f) TEM images of Eu3+:CsPbBr3 QDs prepared via melt-quenching. Reproduced with permission from [Citation121]. © 2017 ACS publications, and [Citation127]. © 2019 ScienceDirect.
![Figure 6. Schematic illustrations of (a) the structures of crystal lattice of perovskite CsPbX3 before and after Mn2+doping; (b) the formation of CsPbBr3 and Eu3+:CsPbBr3 QDs via melt-quenching. (c-d) TEM images of Mn2+:CsPbX3 QDs prepared via HI. (e-f) TEM images of Eu3+:CsPbBr3 QDs prepared via melt-quenching. Reproduced with permission from [Citation121]. © 2017 ACS publications, and [Citation127]. © 2019 ScienceDirect.](/cms/asset/dad8754e-2b8f-4d06-8490-2c38f82d44f5/tsta_a_1752115_f0006_oc.jpg)
Melt-quenching was used in 2019 for Sn2+ and Eu3+ doping by embedding HP-QDs inside borosilicate glass matrix [Citation128]. Wu et al. reported Eu3+:CsPbBr3 QDs composites synthesized via blending Eu2O3, precursors of glass and precursors of CsPbBr3 together with proper ratio followed by heat treatment and cooling () [Citation127]. Sn2+:CsPbBr3 QDs composite was obtained similarly using SnBr2 instead as the source of Sn2+. This method promised enhanced stability against heat and open air due to the protection of glass in the synthesis process [Citation125].
Ion-doped HP-QDs were combined with other compositing strategies mentioned above for enhanced stability, including encapsulating Mn2+: CsPbX3 QDs in polymer (epoxy resin) matrix [Citation129], in oxide (SAM) matrix [Citation130], in PMMA matrix and silica shell [Citation131]. Ion doping for OHP-QDs using Cs to obtain organic-inorganic hybrid HP-QDs with improved optical properties and stability have also been studied [Citation132,Citation133].
2.2.4. HP-QDs/QDs composite
HP-QDs/HP-QDs composites, also called dual-phase HP-QDs composites, exhibited enhanced current efficiency, ionic conductivity, structural stability and emission lifetime [Citation134,Citation135]. The crystal structure of dual-phase CsPbBr3/CsPb2Br5 composite is shown in . Its synthesis strategies include temperature-assisted methods and saturated recrystallization methods.
Figure 7. Crystal structures of (a) CsPbBr3, (b) CsPb2Br5 and (c) dual-phase CsPbBr3/CsPb2Br5 composite. Reproduced with permission from [Citation134]. © 2018 ScienceDirect.
![Figure 7. Crystal structures of (a) CsPbBr3, (b) CsPb2Br5 and (c) dual-phase CsPbBr3/CsPb2Br5 composite. Reproduced with permission from [Citation134]. © 2018 ScienceDirect.](/cms/asset/641c02c5-8dbe-4197-918b-c91a229a2327/tsta_a_1752115_f0007_oc.jpg)
Temperature-assisted method was studied in 2016 by Zhang et al. [Citation135]. In their report, the phase transition of HP-QDs at specific temperature was stated and dual-phase CsPbBr3/CsPb2Br5 composite was obtained via synthesizing CsPbBr3 QDs first in low temperature (100°C) followed by heat-treatment to 130°C, with excess PbBr2 as resources. Similarly, Song’s group synthesized CsPbBr3/CsPb2Br5 composite via a traditional hot-injection process (190°C) only with more PbBr2 in the solution [Citation134].
Saturated recrystallization method was always utilized to obtain CsPbBr3/Cs4PbBr6 composites with various ratios by adjusting the ratio of Cs: Pb: Br in the reaction system. When the solution was Cs-rich or Br-rich, the main product would be Cs4PbBr6, which could be transferred into CsPbBr3 via reacting with excess PbBr2. On the contrary, when solution was Pb-rich, CsPbBr3 would be the main product [Citation136,Citation137]. On the basis of this mechanism, Li et al. synthesized CsPbBr3/Cs4PbBr6 composites by injecting precursor into antisolvent TEOS [Citation138]. APTES has also been used here for binary protection of CsPbBr3@Cs4PbBr6/SiO2 structure [Citation139]. Lou et al. obtained the composites via adding Br− into saturated solution of PbBr2 and CsBr2 in 2019 [Citation140]. Comparing with temperature-assisted method, this method is simpler and thus better for large-scale production.
Composites of HP-QDs and other QDs were also reported, such as CsPbBr3/Rb4PbBr6 QDs synthesized via phase transformation [Citation141], CsPbBr3/PbSe nanocomposites with modified structure obtained via facile hot-injection synthesis [Citation142] and CsPbBr3/ZnS QDs prepared by adding zinc precursor and sulfur precursor into the HP-QDs solution followed by physical stirring [Citation143].
3. Applications of HP-QDs based composites
Based on different structures mentioned above, HP-QDs based composites have enhanced performance than pure HP-QDs in various applications, including white light-emitting diodes, photoemission, detector, photocatalyst, photovoltaic and memristor.
3.1. White light-emitting diodes
HP-QDs have been widely applied in white light-emitting diodes (WLED) as phosphor for their unique optical properties including high PL quantum yield and narrow bandwidth [Citation53,Citation144]. For HP-QDs based composites, the particular structure encapsulating HP-QDs within material matrix exhibited an even better performance in WLED since the matrix could not only improve the stability and working lifetime of HP-QDs in an open environment but also prevent anion exchange among different kinds of HP-QDs in the mixture [Citation53].
WLEDs are commonly obtained via combining green phosphor and red phosphor together on the blue LED chip. Various merits such as high color rendering index (CRI), high luminous efficiency (LE) and color coordinate of the Commission International de L’Eclairage (CIE) closer to the standard (0.33, 0.33) are required in WLED application. Since many review papers published recently had comprehensively listed the materials and parameters for HP-QD/matrix’s application in WLED [Citation53,Citation54], here we just stated them briefly. In some works, two types of HP-QDs based composites were blended together as the green/red phosphors () [Citation78,Citation81,Citation83,Citation101,Citation103,Citation145–Citation147]. For example, Sun et al. employed CsPbBr3 QDs/SiO2 composite as the green part and CsPb(Br/I)3 QDs/SiO2 composite as the red part and the as obtained WLED performed (0.33, 0.33) of CIE and LE of 61.2 lm/W [Citation74]. Some other works adopted other materials such as CaAlSiN3 [Citation82], CdSe [Citation77], (Sr, Ca)AlSiN3:Eu2+ [Citation107], CaSrAlN3:Eu2+ [Citation125,Citation148], K2SiF6:Mn4+ [Citation86,Citation91,Citation110] as the red part or YAG:Ce3+ [Citation129,Citation130,Citation149] as green part to be blended with HP-QDs-based composites (). CsPbBr3/SiO2 composite with CaAlSiN3,for example, was applied as green/red phosphor in Cao’s work and color coordinate of (0.3255, 0.3321) with 58.9 lm/W efficiency was obtained [Citation82].
Figure 8. (a) PL spectra of CsPb(Br/I)3 QDs with varying Br/I ratio. (b) Picture of CsPb(Br/I)3 solution under UV light (top) and normal white light (bottom). (c) CIE color coordinates of CsPbBr3, CsPbBr1,2I1.8 and CsPbI3 separately. (d) Electroluminescence spectrum of CsPbBr3 QDs/SHFW composites, K2SiF6:Mn4+ and blue LED chip. (e) CIE color coordinate of CsPbBr3 QDs/SHFW/blue chip WLED. Reproduced with permission from [Citation83]. © 2018 ScienceDirect, and [Citation110]. © 2019 ACS Publications.
![Figure 8. (a) PL spectra of CsPb(Br/I)3 QDs with varying Br/I ratio. (b) Picture of CsPb(Br/I)3 solution under UV light (top) and normal white light (bottom). (c) CIE color coordinates of CsPbBr3, CsPbBr1,2I1.8 and CsPbI3 separately. (d) Electroluminescence spectrum of CsPbBr3 QDs/SHFW composites, K2SiF6:Mn4+ and blue LED chip. (e) CIE color coordinate of CsPbBr3 QDs/SHFW/blue chip WLED. Reproduced with permission from [Citation83]. © 2018 ScienceDirect, and [Citation110]. © 2019 ACS Publications.](/cms/asset/9da03d4f-df3b-49b2-8071-c18da9f3fe96/tsta_a_1752115_f0008_oc.jpg)
3.2. Photoemission
Pure HP-QDs have been widely applied in photoemission due to their outstanding electrical, optical properties. And with great performance as high PL value and external quantum efficiency (EQE), HP-QDs have been reported as promising candidate for photoemission devices as LED and laser [Citation36,Citation150,Citation151]. For the composite structure of HP-QDs embedded in matrix, a good protection layer as silicon or polymer could efficiently enhance its stability against environmental factors, enabling the material to work within water, solar solvent or open air, the adoption of template in the fabrication process could also help maintain a smaller size of HP-QDs [Citation99]. Thus, these merits make HP-QDs based composites great candidates for luminescent ink [Citation99]. The application in light-emitting diodes of HP-QDs/oxide matrix [Citation84,Citation152] and HP-QDs/polymer matrix were studied and high PL emission was well maintained after treated with water () [Citation110], heat or UV light [Citation84,Citation99,Citation152], high color purity via narrow FWHW (of 25 nm) [Citation83,Citation85], high LE (around 80 lm/W) [Citation85], flexibility [Citation90] were successfully obtained. In addition, for the strong scattering properties and greater optical gain, HP-QDs/SiO2 [Citation118], HP-QDs/glass [Citation94] composites had also been applied in random laser emission with obviously decreased threshold (by 50%) and enhanced efficiency (388%) [Citation75].
Figure 9. (a) Stability of PL quantum yield of CsPbBr3 QDs/SHFW in water. (b) Photos of CsPbBr3 QDs/SHFW in water after 3 months and 6 months under UV light (right) and normal white light (left). (c) Photos of CsPbBr3 QDs/SHFW in white light, UV light and under water drop. (d) PL spectra of CsPbBr3 QDs/SHFW composites. Reproduced with permission from [Citation110]. © 2019 ACS Publications.
![Figure 9. (a) Stability of PL quantum yield of CsPbBr3 QDs/SHFW in water. (b) Photos of CsPbBr3 QDs/SHFW in water after 3 months and 6 months under UV light (right) and normal white light (left). (c) Photos of CsPbBr3 QDs/SHFW in white light, UV light and under water drop. (d) PL spectra of CsPbBr3 QDs/SHFW composites. Reproduced with permission from [Citation110]. © 2019 ACS Publications.](/cms/asset/ba4214f4-5149-437a-a24a-b17a97773dac/tsta_a_1752115_f0009_oc.jpg)
The structure of HP-QDs loaded on the surface of oxides and the HP-QDs/HP-QDs composites were also applied in photoemission with improved performance due to their heterojunction structures. For HP-QDs/oxide, the greater energy band gap and ionization potential of Al2O3 and ZrO2 could help prevent electron injection and lead to intense emission [Citation106,Citation112]. For dual face HP-QDs/HP-QDs, the introduced lead-rich CsPb2Br5 QDs were found to help minimize free exciton emission and improve the ionic conductivity of pure CsPbBr3 QDs to reach an outstanding performance in LED with increased lifetime, EQE around 2.21% [Citation135], narrower FWHM (19 nm) [Citation138].
Ion-doping, another type of compositing, was verified to enhance the thermal stability of HP-QDs via enhancing the formation energy [Citation121], and improve their PL efficiency and intensity [Citation127] by modulating the PL kinetics, where EQE of 4.4% was achieved [Citation126]. Besides, by introducing ions, the toxicity of Pb2+ could be reduced since some of them were replaced, and the emission peak position of HP-QDs could be adjusted by controlling the concentration of ions [Citation153]. In the report of Eu3+:CsPbBr3 QDs by Wu et al., partial Eu3+ replaced Pb2+, leading to a blue shift of the peak, while others exhibited red light emission [Citation154]. With the increasing concentration of Eu3+, more of them entered the cell structure of HP-QDs, broadened emission peak and adjusted light from green to blue and then red were achieved [Citation127].
3.3. Sensor
Optical sensing devices require a wide spectral response and high responsivity. Thus, semiconductor heterojunction with high charge separation and transport efficiency would be ideal candidate [Citation113]. For the heterojunction of HP-QDs loaded on oxides’ surface, Al2O3 and ZrO2 were adopted in photoemission for their greater energy band gap, while on the contrary, possessing conductive level below the HP-QDs, TiO2, ZnO and SnO2 would easily permit electron injection (). Hence, the difference in band gap leads to efficient charge separation while the easy electron injection means fast electron transfer from HP-QDs to the oxide which ensures higher responsivity [Citation117,Citation155,Citation156]. With these merits, the HP-QDs/TiO2 composite was applied as a great sensitizer where TiO2 play the role of n-type semiconductor [Citation111,Citation157–Citation159].
For titanium, Zheng et al. decorated MAPbI3 QDs on TiO2 NTs to form heterojunctions with physical contact (). In their work, the MAPbI3/TiO2 NTs composites not only maintained the pure titanium’s absorption of UV light but also significantly improved the response performance in visible light for a broadened detection range. Also, the composites were verified to be more tolerant to moist air (72 h) and heat (100°C), and exhibited great flexibility and transparency (85%) [Citation113]. HP-QDs/TiO2 composite with chemical contact using linker molecule MPA was reported by Zhou et al. They found that the composite with MPA showed much faster electron transfer from QDs to TiO2 (40 ns) than simple physical contact composite (290 ns). Thus, the responsivity was significantly enhanced from 2.2 AW−1 to 24.5 AW−1. It is also proved that the linker molecule could help attach more HP-QDs on the surface of TiO2 for higher efficiency [Citation117,Citation160]. Besides, MAPbBr3/TiO2 composites [Citation111] and MAPbI3/TiO2 nanowires (NWs) [Citation113], were all reported for the application in photodetector, reaching broadband detection (UV to entire visible range) and high electron injection efficiency (near 99%, ) [Citation161].
Figure 10. Energy level diagram of (a) MAPbI3/TiO2, (b) CsPbBr3/TiO2 and CsPbBr3/ZrO2. (c) Schematic illustration of MAPbI3/TiO2 NTs composites. (d) I–V curves and (e) I-t curves of pure TiO2 NTs, MAPbI3/TiO2 NTs and MAPbI3/TiO2 NWs under darkness, 350 nm light, 700 nm light. Reproduced with permission from [Citation113]. © 2017 Wiley, and [Citation157]. © 2018 ACS Publications.
![Figure 10. Energy level diagram of (a) MAPbI3/TiO2, (b) CsPbBr3/TiO2 and CsPbBr3/ZrO2. (c) Schematic illustration of MAPbI3/TiO2 NTs composites. (d) I–V curves and (e) I-t curves of pure TiO2 NTs, MAPbI3/TiO2 NTs and MAPbI3/TiO2 NWs under darkness, 350 nm light, 700 nm light. Reproduced with permission from [Citation113]. © 2017 Wiley, and [Citation157]. © 2018 ACS Publications.](/cms/asset/dc876ed1-49ec-4b1a-94e6-18aaa61721c9/tsta_a_1752115_f0010_oc.jpg)
HP-QDs/polymer composites have been applied in chemical sensors too. Wang et al. used PS fiber membrane to both improve the stability of HP-QDs and enhance the surface area for sensing Rhodamine 6 G [Citation98]. And special molecularly imprinted polymer was adopted for its unique chemical properties as a recognition system for the HP-QDs to achieve high sensitivity and specificity in sensing phoxim [Citation108].
3.4. Photocatalyst and photovoltaic
Photocatalyst for CO2 reduction, water splitting or degradation of organic compounds have attracted great attention as a promising strategy for solar-chemical conversion. Various materials have been applied for high efficiency, high selectivity and stable photocatalyst that could utilized visible light [Citation162,Citation163]. Processing a wide absorption range for visible light and long carrier diffusion length, HP-QDs could be ideal candidates for photocatalyst only if their poor chemical stability could be improved [Citation164–Citation166].
For HP-QDs based composites, the structure of HP-QDs loaded on the surface of the matrix were preferred for the application in photocatalyst since the heterojunction structure of QDs with functional materials could not only improve the stability but also reach higher electron transportation efficiency. CsPbBr3 QD/GO composite was firstly studied in catalysing CO2 reduction into solar fuels via injecting electrons into CO2, reaching selectivity over 99.3% and improved charge consumption rate [Citation116]. In that work, enhanced EQE and similar light absorbance of the composites compared to pure HP-QDs were observed (), indicating that the enhancement in efficiency mainly came from enhanced charge separation and transportation efficiency [Citation167]. This was further confirmed by the tests on photoelectrochemical performances, where improved photocurrent response and reduced charge-transfer resistance were observed (). And, by using ethyl acetate as the solvent for CO2, where ethyl acetate could help stabilize the QDs, no degradation was observed after working 12 h [Citation116]. Other composites as CsPbBr3/g-C3N4 nanosheet with chemical bonding [Citation168], HP-QDs/metal-organic framework (MOF) with outstanding yield of 1559 μmol/g were also reported [Citation169,Citation170]. Another composite Ag-CsPbBr3/CN was fabricated and reported by Zhao et al. to degrade 7-aminocephalosporanic acid and exhibited outstanding catalytic activity due to the reduced charge recombination, improved charge-separation efficiency and light absorption of the whole composite structure [Citation115].
Figure 11. (a) Yield of product from CO2 reduction and (b) quantum efficiency using CsPbBr3 QD and CsPbBr3 QD/GO composite as the photocatalyst, respectively. (c) I-t curves under −0.4 V and electrochemical impedance Nyquist plots under 150 mW/cm2 and −0.4 V. Reproduced with permission from [Citation116]. © 2017 ACS Publications.
![Figure 11. (a) Yield of product from CO2 reduction and (b) quantum efficiency using CsPbBr3 QD and CsPbBr3 QD/GO composite as the photocatalyst, respectively. (c) I-t curves under −0.4 V and electrochemical impedance Nyquist plots under 150 mW/cm2 and −0.4 V. Reproduced with permission from [Citation116]. © 2017 ACS Publications.](/cms/asset/4d2401ed-5e82-4b18-84ce-2bac72382a54/tsta_a_1752115_f0011_oc.jpg)
As for photovoltaic, devices with high power conversion efficiency (PCE), low cost and great stability are required. In perovskite solar cell, introducing composite materials could help improve the phase stability and carrier mobility of the HP-QDs. For example, Sanehira et al. adopted A-site cation halide salt (AX)-coated CsPbI3 QDs where AX salt treatment could double the mobility, enhance the photocurrent and achieve highest PCE at 13.4% and short-circuit current density (JSC) of 14.37 mA/cm2 [Citation171]. And in silicon solar cell (), the HP-QDs based composites could be used as luminescent downconverter layer [Citation172], such as Mn2+:CsPbCl3 [Citation122,Citation173]. The efficiency of the original silicon solar cell () was low for short wavelength because of the undesirable parasitic optical absorption, which resulted in recombination loss and limited the device performance [Citation174]. Using Mn2+:CsPbCl3 as the composite layer on the front of silicon, the electrons excited by UV light would then relax to the lower band of Mn2+ (4T1, as shown in ). Thus, with a large Stokes shift (200 nm) and high PL quantum yield (62%), this composite layer could convert UV light into visible light (). With increasing concentration of Mn2+:CsPbCl3, the surface reflectance of the device on UV light significantly decreased, indicating that more UV light was absorbed and then converted () to reach a higher PCE. This device obtained enhanced JSC (by 5.1%) and PCE (by 6.2%) compared to the original silicon device [Citation122].
Figure 12. (a) Schematic illustration and (b) EQE of traditional silicon solar cell. (c) Energy level diagram of Mn2+:CsPbCl3. (d) Working lifetime of pure Mn2+, CsPbCl3 QDs and Mn2+:CsPbCl3 QDs. (e) Schematic illustration and (f) Surface reflectance spectra of silicon solar cell with Mn2+:CsPbCl3 layer. Influence of Mn2+:CsPbCl3 QDs layer on second-reflection with different concentration. (g) 3 mg/mL and (h) 18 mg/mL. Reproduced with permission from [Citation122]. © 2018 ScienceDirect.
![Figure 12. (a) Schematic illustration and (b) EQE of traditional silicon solar cell. (c) Energy level diagram of Mn2+:CsPbCl3. (d) Working lifetime of pure Mn2+, CsPbCl3 QDs and Mn2+:CsPbCl3 QDs. (e) Schematic illustration and (f) Surface reflectance spectra of silicon solar cell with Mn2+:CsPbCl3 layer. Influence of Mn2+:CsPbCl3 QDs layer on second-reflection with different concentration. (g) 3 mg/mL and (h) 18 mg/mL. Reproduced with permission from [Citation122]. © 2018 ScienceDirect.](/cms/asset/35ea5b56-6267-4fbe-bfd5-3643c42d8298/tsta_a_1752115_f0012_oc.jpg)
3.5. Memristor
Possessing excellent photoelectronic properties, HP-QDs were also considered as promising candidates for memristor via light-stimulated resistive switching; however, instability to environmental factors, low electron-transport efficiency and easy interfacial reaction with electrode layer limit their application [Citation175,Citation176]. Stable devices with high photoresponsivity and efficiency are required for light-stimulated memristor. Forming the composite structure of HP-QDs encapsulated in matrix such as PMMA is an efficient strategy in improving the stability. Adopting CsPbCl3/PMMA composite as the active layer, the memristive device exhibited improved retention time (104 s) [Citation177]. HP-QDs/organic-semiconductor (poly(3,3-didodecylquarterthiophene) ‘PQT-12’ was used here) composite in this application was also studied, where HP-QDs/PQT-12 composite film was utilized as light-absorbing and charge-transporting layer () [Citation178]. Compared to pure HP-QDs and pure PQT-12, the composite exhibited enhanced optical absorption of a wider spectrum (), quenched PL intensity for reduced rate of carrier recombination (). With these merits and the disordered interfaces of the composite, this device exhibited improved charge-separation efficiency and induced delayed decay () for higher photoresponsivity and efficiency [Citation178].
Loading HP-QDs on the material’s surface is another method to protect the HP-QDs, where the protection layer such as PMMA could be formed on both sides of HP-QDs [Citation179,Citation180]. And for functional oxide materials such as ZnO, not only could the oxide layer prevent HP-QDs from contacting the electrode but also it help form HP-QDs/ZnO heterojunction to achieve enhanced rapid response speed (<1 ms) [Citation181].
Figure 13. (a) TEM image and (b) XRD pattern of pure CsPbBr3 QDs. (c) Schematic illustration of the device structure. (d) Optical absorption and (e) PL spectrum of pure CsPbBr3 QDs, PQT-12 and CsPbBr3 QDs/PQT-12 composites. Optical response of CsPbBr3 QDs/PQT-12 composites. Reproduced with permission from [Citation178]. © 2019 Wiley.
![Figure 13. (a) TEM image and (b) XRD pattern of pure CsPbBr3 QDs. (c) Schematic illustration of the device structure. (d) Optical absorption and (e) PL spectrum of pure CsPbBr3 QDs, PQT-12 and CsPbBr3 QDs/PQT-12 composites. Optical response of CsPbBr3 QDs/PQT-12 composites. Reproduced with permission from [Citation178]. © 2019 Wiley.](/cms/asset/af649b98-45ca-4a5e-969c-1ecf7c192154/tsta_a_1752115_f0013_oc.jpg)
4. Summary
Here, we have presented various works on HP-QDs based composites with different structures and enhanced properties. On the one hand, to enhance the stability of pure HP-QDs, two strategies of compositing were explored. By encapsulating HP-QDs into material matrix or more precisely core-shell structure, the protection materials including oxides, glass, organic polymers, salts, semiconductors and MOFs could enhance the stability against environmental factors and maintain their unique optical properties at the same time. Template-free methods with outstanding monodispersity and template method having better control in size confinement of the QDs are all discussed. And by ion-doping, the lead in the perovskite lattice was replaced by mental ions to some extend to improve its formation energy and by so enhance the thermal stability. On the other hand, heterojunctions with various functional materials for enhanced optical or photoelectronic properties were obtained in composites via loading HP-QDs on the surface physically by spin-coating or chemically by linker molecules and HP-QDs/QDs structures.
These composites exhibiting enhanced stability and photoelectronic properties enabled HP-QDs to be better applied in various applications including photo-emission, photocatalytic, photodetector, photovoltaic, light-stimulated memristor and some chemical sensors. Benefit from the protecting layer with much lower penetration rate of molecules from the environment, the HP-QDs/matrix composites with great water-resistance, long-term stability and reduced anion-exchange effect shows promising potential in LED and WLED. Ion-doping for enhanced thermal stability and modulated PL kinetics have also attracted attentions in LED and solar cell for high PL efficiency, light conversion efficiency and reduced Pb toxicity of their lattice structure. By forming heterojunctions of HP-QDs with other functional materials, the composites could exhibit different merits for various chosen materials. Oxides with different energy band for HP-QDs/oxide heterostructures may induce limited electron injection for intense light emission in LED or enhanced electron injection for great performance in photocatalytic and photodetector, and semiconductor materials with enhanced efficiency in charge separation and transport can be used in light-stimulated memristor. However, protecting matrix layer that isolates the QDs well would unavoidably limit its photoelectronic performance to some extent, and functional materials forming heterojunctions with enhanced photoelectronic properties always have little contribution to the instability issue of HP-QDs. To combine the merits of different structures for a better performance in application, various matrix materials for better maintained properties, composites with more than one structure mentioned above, and functional materials that could both form heterojunction and play as capping matrix have all been explored. Recent examples include 1) paraffin as encapsulating material with high transparency to UV light that can maintain the high PL quantum yield of HP-QDs [Citation182], 2) poly(ethylene oxide) as matrix to both protect the ligands and deactivate the defects at the QDs surface [Citation183] and 3) MOF materials that enhance the stability and carrier-transport efficiency of HP-QDs [Citation169,Citation170]. We believe that composites with better performance will be achieved in the future.
Acknowledgments
The authors acknowledge the grants from National Natural Science Foundation of China (Grant Nos. 61974093 and 51902205), Guangdong Province Special Support Plan for High-Level Talents (Grant No. 2017TQ04X082), Guangdong Natural Science Funds for Distinguished Young Scholar (Grant No. 2018B030306028), the Science and Technology Innovation Commission of Shenzhen (Grant Nos. JCYJ20180507182042530 and JCYJ20180507182000722) and the Natural Science Foundation of SZU (Grant No. 860-000002110420).
Disclosure statement
No potential conflict of interest was reported by the authors.
Additional information
Funding
Notes on contributors

Yaxin Wang
Yaxin Wang is currently an undergraduate student in the Institute for Advanced Study at Shenzhen University. Her research interest focuses on 2D functional material-based memory devices.

Ye Zhou
Prof. Ye Zhou is an IAS Fellow in the Institute for Advanced Study, Shenzhen University. His research interests include flexible and printed electronics, organic/inorganic semiconductors, surface and interface physics, nanostructured materials, and nano-scale devices for technological applications, such as logic circuits, data storage, energy harvesting, photonics and sensors.

Su-Ting Han
Prof. Su-Ting Han is an associate professor at Shenzhen University and a visiting associate professor at The University of Michigan. She received her MSc degree in Analytical Chemistry from Hong Kong Baptist University and her PhD degree in Physics and Materials Science from City University of Hong Kong. Her research interests include functional electronic devices and flexible, stretchable, and wearable electronics.
References
- Zhang S, Sunami Y, Hashimoto H. Mini review: nanosheet technology towards biomedical application. Nanomaterials-Basel. 2017;7(9):246.
- Shamsaei E, de Souza FB, Yao X, et al. Graphene-based nanosheets for stronger and more durable concrete: A review. Constr Build Mater. 2018;183:642–660.
- LaPierre RR, Chia ACE, Gibson SJ, et al. III–V nanowire photovoltaics: review of design for high efficiency. Phys Status Solidi-R. 2013;7(10):815–830.
- Zimmler MA, Capasso F, Müller S, et al. Optically pumped nanowire lasers: invited review. Semicond Sci Tech. 2010;25(2):024001.
- Langley D, Giusti G, Mayousse C, et al. Flexible transparent conductive materials based on silver nanowire networks: a review. Nanotechnology. 2013;24(45):452001.
- Yogi C, Kojima K, Hashishin T, et al. Size effect of Au nanoparticles on TiO2 crystalline phase of nanocomposite thin films and their photocatalytic properties. J Phys Chem C. 2011;115(14):6554–6560.
- Liu L, Chan J, Sham T-K. Calcination-induced phase transformation and accompanying optical luminescence of TiO2 nanotubes: an X-ray absorption near-edge structures and X-ray excited optical luminescence study. J Phys Chem C. 2010;114(49):21353–21359.
- Kuo -C-C, Lin C-H, Chen W-C. Morphology and photophysical properties of light-emitting electrospun nanofibers prepared from poly(fluorene) derivative/PMMA blends. Macromolecules. 2007;40(19):6959–6966.
- Chen -Y-Y, Kuo -C-C, Chen B-Y, et al. Multifunctional polyacrylonitrile-ZnO/Ag electrospun nanofiber membranes with various ZnO morphologies for photocatalytic, UV-shielding, and antibacterial applications. J Polym Sci Pol Phys. 2015;53(4):262–269.
- Ding G, Yang B, Zhou K, et al. Synaptic plasticity and filtering emulated in metal–organic frameworks nanosheets based transistors. Adv Electron Mater. 2020;6(1):1900978.
- Ding G, Zeng K, Zhou K, et al. Configurable multi-state non-volatile memory behaviors in Ti3C2 nanosheets. Nanoscale. 2019;11(15):7102–7110.
- Qian L, Zheng Y, Xue J, et al. Stable and efficient quantum-dot light-emitting diodes based on solution-processed multilayer structures. Nat Photonics. 2011;5(9):543–548.
- Colvin VL, Schlamp MC, Alivisatos AP. Light-emitting diodes made from cadmium selenide nanocrystals and a semiconducting polymer. Nature. 1994;370(6488):354–357.
- Coe S, Woo W-K, Bawendi M, et al. Electroluminescence from single monolayers of nanocrystals in molecular organic devices. Nature. 2002;420(6917):800–803.
- Dang C, Lee J, Breen C, et al. Red, green and blue lasing enabled by single-exciton gain in colloidal quantum dot films. Nat Nanotechnol. 2012;7(5):335–339.
- Nozik AJ. Quantum dot solar cells. Physica E. 2002;14(1):115–120.
- Kamat PV. Quantum dot solar cells. The next big thing in photovoltaics. J Phys Chem Lett. 2013;4(6):908–918.
- Malgras V, Nattestad A, Kim JH, et al. Understanding chemically processed solar cells based on quantum dots. Sci Technol Adv Mat. 2017;18(1):334–350.
- Konstantatos G, Howard I, Fischer A, et al. Ultrasensitive solution-cast quantum dot photodetectors. Nature. 2006;442(7099):180–183.
- Bera D, Qian L, Tseng T-K, et al. Quantum dots and their multimodal applications: a review. Materials. 2010;3(4):2260–2345.
- Ghaderi S, Ramesh B, Seifalian AM. Fluorescence nanoparticles “quantum dots” as drug delivery system and their toxicity: a review. J Drug Target. 2011;19(7):475–486.
- Frigerio C, Ribeiro DSM, Rodrigues SSM, et al. Application of quantum dots as analytical tools in automated chemical analysis: A review. Anal Chim Acta. 2012;735:9–22.
- Algar WR, Tavares AJ, Krull UJ. Beyond labels: A review of the application of quantum dots as integrated components of assays, bioprobes, and biosensors utilizing optical transduction. Anal Chim Acta. 2010;673(1):1–25.
- Ai B, Liu C, Wang J, et al. Precipitation and optical properties of CsPbBr3Quantum dots in phosphate glasses. J Am Ceram Soc. 2016;99(9):2875–2877.
- Chamarro M, Gourdon C, Lavallard P, et al. Enhancement of electron-hole exchange interaction in CdSe nanocrystals: A quantum confinement effect. Phys Rev B. 1996;53(3):1336–1342.
- Jun Y-W, Choi C-S, Cheon J. Size and shape controlled ZnTe nanocrystals with quantum confinement effect. Chem Commun (Camb). 2001;1:101–102.
- Chen L, Lai C, Marchewka R, et al. Use of CdS quantum dot-functionalized cellulose nanocrystal films for anti-counterfeiting applications. Nanoscale. 2016;8(27):13288–13296.
- Yamasaki Y, Asami H, Isoshima T, et al. Multi-wavelength intermittent photoluminescence of single CdSe quantum dots. Sci Technol Adv Mat. 2003;4(6):519–522.
- Samadpour M. Efficient CdS/CdSe/ZnS quantum dot sensitized solar cells prepared by ZnS treatment from methanol solvent. Sol Energy. 2017;144:63–70.
- Shen Q, Katayama K, Sawada T, et al. Ultrafast carrier dynamics in PbS quantum dots. Chem Phys Lett. 2012;542:89–93.
- Ahmad W, He J, Liu Z, et al. Lead Selenide (PbSe) colloidal quantum dot solar cells with >10% efficiency. Adv Mater. 2019;31(33):1900593.
- Li Y, Wang Z, Ren D, et al. SnS quantum dots as hole transporter of perovskite solar cells. ACS Appl Energ Mater. 2019;2(5):3822–3829.
- Oda Y, Shen H, Zhao L, et al. Energetic alignment in nontoxic SnS quantum dot-sensitized solar cell employing spiro-OMeTAD as the solid-state electrolyte. Sci Technol Adv Mat. 2014;15(3):035006.
- Zhang Y, Hao S, Zhao L-D, et al. Pressure induced thermoelectric enhancement in SnSe crystals. J Mater Chem A. 2016;4(31):12073–12079.
- Ghosh B, Shirahata N. Colloidal silicon quantum dots: synthesis and luminescence tuning from the near-UV to the near-IR range. Sci Technol Adv Mat. 2014;15(1):014207.
- Le QV, Hong K, Jang HW, et al. Halide perovskite quantum dots for light-emitting diodes: properties, synthesis, applications, and outlooks. Adv Electron Mater. 2018;4(12):1800335.
- Jung M, Shin TJ, Seo J, et al. Structural features and their functions in surfactant-armoured methylammonium lead iodide perovskites for highly efficient and stable solar cells. Energ Environ Sci. 2018;11(8):2188–2197.
- Huang H, Bodnarchuk MI, Kershaw SV, et al. Lead halide perovskite nanocrystals In The Research Spotlight: stability and defect tolerance. ACS Energy Lett. 2017;2(9):2071–2083.
- Shi Z, Li Y, Zhang Y, et al. High-efficiency and air-stable perovskite quantum dots light-emitting diodes with an all-inorganic heterostructure. Nano Lett. 2017;17(1):313–321.
- Mei S, Liu X, Zhang W, et al. High-bandwidth white-light system combining a micro-LED with perovskite quantum dots for visible light communication. ACS Appl Mater Inter. 2018;10(6):5641–5648.
- Zhang X, Liu H, Wang W, et al. Hybrid perovskite light-emitting diodes based on perovskite nanocrystals with organic–inorganic mixed cations. Adv Mater. 2017;29(18):1606405.
- Jiang C, Yao J, Huang P, et al. Perovskite quantum dots exhibiting strong hole extraction capability for efficient inorganic thin film solar cells. Cell Rep Phys Sci. 2020;1(1):100001.
- Ramasamy P, Lim D-H, Kim B, et al. All-inorganic cesium lead halide perovskite nanocrystals for photodetector applications. Chem Commun (Camb). 2016;52(10):2067–2070.
- Li Y, Shu Q, Du Q, et al. Surface modification for improving the photocatalytic polymerization of 3,4-ethylenedioxythiophene over inorganic lead halide perovskite quantum dots. ACS Appl Mater Inter. 2020;12(1):451–460.
- Yang H, Liu Y, Wu X, et al. High-performance all-inorganic perovskite-quantum-dot-based flexible organic phototransistor memory with architecture design. Adv Electron Mater. 2019;5(12):1900864.
- Dai S-W, Hsu B-W, Chen C-Y, et al. Perovskite quantum dots with near unity solution and neat-film photoluminescent quantum yield by novel spray synthesis. Adv Mater. 2018;30(7):1705532.
- Zhao Y, Li J, Dong Y, et al. Synthesis of colloidal halide perovskite quantum dots/nanocrystals: progresses and advances. Isr J Chem. 2019;59(8):649–660.
- Moon H, Lee C, Lee W, et al. Stability of quantum dots, quantum dot films, and quantum dot light-emitting diodes for display applications. Adv Mater. 2019;31(34):1804294.
- Yang J, Siempelkamp BD, Liu D, et al. Investigation of CH3NH3PbI3 degradation rates and mechanisms in controlled humidity environments using in situ techniques. ACS Nano. 2015;9(2):1955–1963.
- Christians JA, Miranda Herrera PA, Kamat PV. Transformation of the excited state and photovoltaic efficiency of CH3NH3PbI3 perovskite upon controlled exposure to humidified air. J Am Chem Soc. 2015;137(4):1530–1538.
- Huang S, Li Z, Wang B, et al. Morphology evolution and degradation of CsPbBr3 nanocrystals under blue light-emitting diode illumination. ACS Appl Mater Inter. 2017;9(8):7249–7258.
- Palazon F, Di Stasio F, Lauciello S, et al. Evolution of CsPbBr3 nanocrystals upon post-synthesis annealing under an inert atmosphere. J Mater Chem C. 2016;4(39):9179–9182.
- Lv W, Li L, Xu M, et al. Improving the stability of metal halide perovskite quantum dots by encapsulation. Adv Mater. 2019;31(28):e1900682.
- Liu J, Yang Z, Ye B, et al. A review of stability-enhanced luminescent materials: fabrication and optoelectronic applications. J Mater Chem C. 2019;7(17):4934–4955.
- Protesescu L, Yakunin S, Bodnarchuk MI, et al. Nanocrystals of cesium lead halide perovskites (CsPbX3, X = Cl, Br, and I): novel optoelectronic materials showing bright emission with wide color gamut. Nano Lett. 2015;15(6):3692–3696.
- Shamsi J, Urban AS, Imran M, et al. Metal halide perovskite nanocrystals: synthesis, post-synthesis modifications, and their optical properties. Chem Rev. 2019;119(5):3296–3348.
- Liu M, Zhang H, Gedamu D, et al. Halide perovskite nanocrystals for next-generation optoelectronics. Small. 2019;15(28):1900801.
- Katan C, Mercier N, Even J. Quantum and dielectric confinement effects in lower-dimensional hybrid perovskite semiconductors. Chem Rev. 2019;119(5):3140–3192.
- Song J, Li J, Li X, et al. Quantum dot light-emitting diodes based on inorganic perovskite cesium lead halides (CsPbX3). Adv Mater. 2015;27(44):7162–7167.
- Liu C, Wang K, Gong X, et al. Low bandgap semiconducting polymers for polymeric photovoltaics. Chem Soc Rev. 2016;45(17):4825–4846.
- Kim Y-H, Wolf C, Kim Y-T, et al. Highly efficient light-emitting diodes of colloidal metal–halide perovskite nanocrystals beyond quantum size. ACS Nano. 2017;11(7):6586–6593.
- Kim Y-H, Lee G-H, Kim Y-T, et al. High efficiency perovskite light-emitting diodes of ligand-engineered colloidal formamidinium lead bromide nanoparticles. Nano Energy. 2017;38:51–58.
- Jeon NJ, Noh JH, Kim YC, et al. Solvent engineering for high-performance inorganic–organic hybrid perovskite solar cells. Nat Mater. 2014;13(9):897–903.
- Wei Y, Cheng Z, Lin J. An overview on enhancing the stability of lead halide perovskite quantum dots and their applications in phosphor-converted LEDs. Chem Soc Rev. 2019;48(1):310–350.
- Quan LN, Rand BP, Friend RH, et al. Perovskites for next-generation optical sources. Chem Rev. 2019;119(12):7444–7477.
- Zhou Y, Zhao H, Ma D, et al. Harnessing the properties of colloidal quantum dots in luminescent solar concentrators. Chem Soc Rev. 2018;47(15):5866–5890.
- Wang H, Kim DH. Perovskite-based photodetectors: materials and devices. Chem Soc Rev. 2017;46(17):5204–5236.
- Zeng Z, Xu Y, Zhang Z, et al. Rare-earth-containing perovskite nanomaterials: design, synthesis, properties and applications. Chem Soc Rev. 2020;49(4):1109–1143.
- Zhong Q, Cao M, Hu H, et al. One-pot synthesis of highly stable CsPbBr3@SiO2 core-shell nanoparticles. ACS Nano. 2018;12(8):8579–8587.
- Li Z-J, Hofman E, Li J, et al. Photoelectrochemically active and environmentally stable CsPbBr3/TiO2Core/shell nanocrystals. Adv Funct Mater. 2018;28(1):1704288.
- Selvan ST, Tan TT, Ying JY. Robust, non-cytotoxic, silica-coated CdSe quantum dots with efficient photoluminescence. Adv Mater. 2005;17(13):1620–1625.
- Zhao B, Yao Y, Gao M, et al. Doped quantum dot@silica nanocomposites for white light-emitting diodes. Nanoscale. 2015;7(41):17231–17236.
- Venkateswara Rao A, Bhagat SD. Synthesis and physical properties of TEOS-based silica aerogels prepared by two step (acid–base) sol–gel process. Solid State Sci. 2004;6(9):945–952.
- Sun C, Zhang Y, Ruan C, et al. Efficient and stable white LEDs with silica-coated inorganic perovskite quantum dots. Adv Mater. 2016;28(45):10088–10094.
- Hu Z, Liu Z, Bian Y, et al. Enhanced two-photon-pumped emission from in situ synthesized nonblinking CsPbBr3/SiO2 nanocrystals with excellent stability. Adv Opt Mater. 2018;6(3):1700997.
- Cai J, Gu K, Zhu Y, et al. Highly stable CsPbBr3@SiO2 nanocomposites prepared via confined condensation for use as a luminescent ink. Chem Commun (Camb). 2018;54(58):8064–8067.
- Hu H, Wu L, Tan Y, et al. Interfacial synthesis of highly stable CsPbX3/oxide janus nanoparticles. J Am Chem Soc. 2018;140(1):406–412.
- Wei Y, Xiao H, Xie Z, et al. Highly luminescent lead halide perovskite quantum dots in hierarchical CaF2 matrices with enhanced stability as phosphors for white light-emitting diodes. Adv Opt Mater. 2018;6(11):1701343.
- Yang G, Fan Q, Chen B, et al. Reprecipitation synthesis of luminescent CH3NH3PbBr3/NaNO3 nanocomposites with enhanced stability. J Mater Chem C. 2016;4(48):11387–11391.
- Pang X, Zhang H, Xie L, et al. Precipitating CsPbBr3 quantum dots in boro-germanate glass with a dense structure and inert environment toward highly stable and efficient narrow-band green emitters for wide-color-gamut liquid crystal displays. J Mater Chem C. 2019;7(42):13139–13148.
- Sun C, Shen X, Zhang Y, et al. Highly luminescent, stable, transparent and flexible perovskite quantum dot gels towards light-emitting diodes. Nanotechnology. 2017;28(36):365601.
- Cao P, Yang B, Zheng F, et al. High stability of silica-wrapped CsPbBr3 perovskite quantum dots for light emitting application. Ceram Int. 2019;46(3):3882–3888.
- Park DH, Han JS, Kim W, et al. Facile synthesis of thermally stable CsPbBr3 perovskite quantum dot-inorganic SiO2 composites and their application to white light-emitting diodes with wide color gamut. Dyes Pigm. 2018;149:246–252.
- Song T, Feng X, Ju H, et al. Enhancing acid, base and UV light resistance of halide perovskite CH3NH3PbBr3 quantum dots by encapsulation with ZrO2 sol. J Alloy Compd. 2019;816:152558.
- Li Z, Kong L, Huang S, et al. Highly luminescent and ultrastable CsPbBr3 perovskite quantum dots incorporated into a silica/alumina monolith. Angew Chem Int Ed Engl. 2017;56(28):8134–8138.
- Yoon HC, Lee S, Song JK, et al. Efficient and stable CsPbBr3 quantum-dot powders passivated and encapsulated with a mixed silicon nitride and silicon oxide inorganic polymer matrix. ACS Appl Mater Inter. 2018;10(14):11756–11767.
- Loiudice A, Saris S, Oveisi E, et al. CsPbBr3 QD/AlOx inorganic nanocomposites with exceptional stability in water, light, and heat. Angew Chem Int Ed Engl. 2017;56(36):10696–10701.
- Yin B, Sadtler B, Berezin MY, et al. Quantum dots protected from oxidative attack using alumina shells synthesized by atomic layer deposition. Chem Commun (Camb). 2016;52(74):11127–11130.
- Di D, Musselman KP, Li G, et al. Size-dependent photon emission from organometal halide perovskite nanocrystals embedded in an organic matrix. J Phys Chem Lett. 2015;6(3):446–450.
- Li G, Tan ZK, Di D, et al. Efficient light-emitting diodes based on nanocrystalline perovskite in a dielectric polymer matrix. Nano Lett. 2015;15(4):2640–2644.
- Zhou Q, Bai Z, Lu WG, et al. In situ fabrication of halide perovskite nanocrystal-embedded polymer composite films with enhanced photoluminescence for display backlights. Adv Mater. 2016;28(41):9163–9168.
- Wang Y, He J, Chen H, et al. Ultrastable, highly luminescent organic-inorganic perovskite-polymer composite films. Adv Mater. 2016;28(48):10710–10717.
- Chen D, Yuan S, Chen J, et al. Robust CsPbX3(X = Cl, Br, and I) perovskite quantum dot embedded glasses: nanocrystallization, improved stability and visible full-spectral tunable emissions. J Mater Chem C. 2018;6(47):12864–12870.
- Yuan S, Chen D, Li X, et al. In situ crystallization synthesis of CsPbBr3 perovskite quantum dot-embedded glasses with improved stability for solid-state lighting and random upconverted lasing. ACS Appl Mater Inter. 2018;10(22):18918–18926.
- Chen D, Liu Y, Yang C, et al. Promoting photoluminescence quantum yields of glass-stabilized CsPbX3 (X = Cl, Br, I) perovskite quantum dots through fluorine doping. Nanoscale. 2019;11(37):17216–17221.
- Ye Y, Zhang W, Zhao Z, et al. Highly luminescent cesium lead halide perovskite nanocrystals stabilized in glasses for light-emitting applications. Adv Opt Mater. 2019;7(9):1801663.
- Wei S, Zhu H, Zhang J, et al. Luminescent perovskite nanocrystal-epoxy resin composite with high stability against water and air. J Alloy Compd. 2019;789:209–214.
- Wang Y, Zhu Y, Huang J, et al. CsPbBr3 perovskite quantum dots-based monolithic electrospun fiber membrane as an ultrastable and ultrasensitive fluorescent sensor in aqueous medium. J Phys Chem Lett. 2016;7(21):4253–4258.
- Liao H, Guo S, Cao S, et al. A general strategy for in situ growth of all-inorganic CsPbX3 (X = Br, I, and Cl) perovskite nanocrystals in polymer fibers toward significantly enhanced water/thermal stabilities. Adv Opt Mater. 2018;6(15):1800346.
- Xin Y, Zhao H, Zhang J. Highly stable and luminescent perovskite–polymer composites from a convenient and universal strategy. ACS Appl Mater Inter. 2018;10(5):4971–4980.
- Wang H-C, Lin S-Y, Tang A-C, et al. Mesoporous silica particles integrated with all-inorganic CsPbBr3 perovskite quantum-dot nanocomposites (MP-PQDs) with high stability and wide color gamut used for backlight display. Angew Chem Int Ed Engl. 2016;55(28):7924–7929.
- Malgras V, Tominaka S, Ryan JW, et al. Observation of quantum confinement in monodisperse methylammonium lead halide perovskite nanocrystals embedded in mesoporous silica. J Am Chem Soc. 2016;138(42):13874–13881.
- Zhenfu Z, Zhihai W, Jiong C, et al. Nanocomposites of perovskite quantum dots embedded in magnesium silicate hollow spheres for multicolor display. J Phys Chem C. 2018;122(29):16887–16893.
- Demchyshyn S, Roemer JM, Groiß H, et al. Confining metal-halide perovskites in nanoporous thin films. Sci Adv. 2017;3(8):e1700738.
- Cha W, Kim H-J, Lee S, et al. Size-controllable and stable organometallic halide perovskite quantum dots/polymer films. J Mater Chem C. 2017;5(27):6667–6671.
- Kojima A, Ikegami M, Teshima K, et al. Highly luminescent lead bromide perovskite nanoparticles synthesized with porous alumina media. Chem Lett. 2012;41(4):397–399.
- Li Y, Lv Y, Guo Z, et al. One-step preparation of long-term stable and flexible CsPbBr3 perovskite quantum dots/ethylene vinyl acetate copolymer composite films for white light-emitting diodes. ACS Appl Mater Inter. 2018;10(18):15888–15894.
- Tan L, Guo M, Tan J, et al. Development of high-luminescence perovskite quantum dots coated with molecularly imprinted polymers for pesticide detection by slowly hydrolysing the organosilicon monomers in situ. Sensors Actuat B-Chem. 2019;291:226–234.
- Huang S, Guo M, Tan J, et al. Novel fluorescence sensor based on all-inorganic perovskite quantum dots coated with molecularly imprinted polymers for highly selective and sensitive detection of omethoate. ACS Appl Mater Inter. 2018;10(45):39056–39063.
- Xuan T, Huang J, Liu H, et al. Super-hydrophobic cesium lead halide perovskite quantum dot-polymer composites with high stability and luminescent efficiency for wide color gamut white light-emitting diodes. Chem Mater. 2019;31(3):1042–1047.
- Kojima A, Teshima K, Shirai Y, et al. Organometal halide perovskites as visible-light sensitizers for photovoltaic cells. J Am Chem Soc. 2009;131(17):6050–6051.
- Longo G, Pertegás A, Martínez-Sarti L, et al. Highly luminescent perovskite–aluminum oxide composites. J Mater Chem C. 2015;3(43):11286–11289.
- Zheng Z, Zhuge F, Wang Y, et al. Decorating perovskite quantum dots in TiO2 nanotubes array for broadband response photodetector. Adv Funct Mater. 2017;27(43):1703115.
- Lu J, Sheng X, Tong G, et al. Ultrafast solar-blind ultraviolet detection by inorganic perovskite CsPbX3 quantum dots radial junction architecture. Adv Mater. 2017;29(23):1700400.
- Zhao Y, Wang Y, Liang X, et al. Enhanced photocatalytic activity of Ag-CsPbBr3/CN composite for broad spectrum photocatalytic degradation of cephalosporin antibiotics 7-ACA. Appl Catal B-Environ. 2019;247:57–69.
- Xu YF, Yang MZ, Chen BX, et al. A CsPbBr3 perovskite quantum dot/graphene oxide composite for photocatalytic CO2 reduction. J Am Chem Soc. 2017;139(16):5660–5663.
- Zhou L, Yu K, Yang F, et al. Insight into the effect of ligand-exchange on colloidal CsPbBr3 perovskite quantum dot/mesoporous-TiO2 composite-based photodetectors: much faster electron injection. J Mater Chem C. 2017;5(25):6224–6233.
- Li X, Wang Y, Sun H, et al. Amino-mediated anchoring perovskite quantum dots for stable and low-threshold random lasing. Adv Mater. 2017;29(36):1701185.
- Lou S, Xuan T, Yu C, et al. Nanocomposites of CsPbBr3 perovskite nanocrystals in an ammonium bromide framework with enhanced stability. J Mater Chem C. 2017;5(30):7431–7435.
- Mocatta D, Cohen G, Schattner J, et al. Heavily doped semiconductor nanocrystal quantum dots. Science. 2011;332(6025):77.
- Zou S, Liu Y, Li J, et al. Stabilizing cesium lead halide perovskite lattice through Mn(II) substitution for air-stable light-emitting diodes. J Am Chem Soc. 2017;139(33):11443–11450.
- Wang F, Yang M, Ji S, et al. Boosting spectral response of multi-crystalline Si solar cells with Mn2+ doped CsPbCl3 quantum dots downconverter. J Power Sources. 2018;395:85–91.
- Liu W, Lin Q, Li H, et al. Mn2+-doped lead halide perovskite nanocrystals with dual-color emission controlled by halide content. J Am Chem Soc. 2016;138(45):14954–14961.
- Parobek D, Roman BJ, Dong Y, et al. Exciton-to-dopant energy transfer in Mn-doped cesium lead halide perovskite nanocrystals. Nano Lett. 2016;16(12):7376–7380.
- Liu S, Shao G, Ding L, et al. Sn-doped CsPbBr3 QDs glasses with excellent stability and optical properties for WLED. Chem Eng J. 2019;361:937–944.
- Yao J-S, Ge J, Han B-N, et al. Ce3+-doping to modulate photoluminescence kinetics for efficient CsPbBr3 nanocrystals based light-emitting diodes. J Am Chem Soc. 2018;140(10):3626–3634.
- Wu J, Bai H, Qu W, et al. Preparation of Eu3+-doped CsPbBr3 quantum-dot microcrystals and their luminescence properties. Opt Mater. 2019;97:109454.
- Liu S, He M, Di X, et al. Precipitation and tunable emission of cesium lead halide perovskites (CsPbX3, X = Br, I) QDs in borosilicate glass. Ceram Int. 2018;44(4):4496–4499.
- He M, Cheng Y, Yuan R, et al. Mn-doped cesium lead halide perovskite nanocrystals with dual-color emission for WLED. Dyes Pigm. 2018;152:146–154.
- He M, Cheng Y, Shen L, et al. Mn-doped CsPbCl3 perovskite quantum dots (PQDs) incorporated into silica/alumina particles used for WLEDs. Appl Surf Sci. 2018;448:400–406.
- Chen D, Fang G, Chen X. Silica-coated Mn-doped CsPb(Cl/Br)3 inorganic perovskite quantum dots: exciton-to-Mn energy transfer and blue-excitable solid-state lighting. ACS Appl Mater Inter. 2017;9(46):40477–40487.
- Yan W, Qu Y, Gupta TD, et al. Semiconducting nanowire-based optoelectronic fibers. Adv Mater. 2017;29(27):606405.
- Xu B, Wang W, Zhang X, et al. Bright and efficient light-emitting diodes based on MA/Cs double cation perovskite nanocrystals. J Mater Chem C. 2017;5(25):6123–6128.
- Song P, Qiao B, Song D, et al. Colour- and structure-stable CsPbBr3-CsPb2Br5 compounded quantum dots with tuneable blue and green light emission. J Alloy Compd. 2018;767:98–105.
- Zhang X, Xu B, Zhang J, et al. All-inorganic perovskite nanocrystals for high-efficiency light emitting diodes: dual-Phase CsPbBr3-CsPb2Br5 composites. Adv Funct Mater. 2016;26(25):4595–4600.
- Akkerman QA, Park S, Radicchi E, et al. Nearly monodisperse insulator Cs4PbX6 (X = Cl, Br, I) nanocrystals, their mixed halide compositions, and their transformation into CsPbX3 nanocrystals. Nano Lett. 2017;17(3):1924–1930.
- Yang H, Zhang Y, Pan J, et al. Room-temperature engineering of all-inorganic perovskite nanocrsytals with different dimensionalities. Chem Mater. 2017;29(21):8978–8982.
- Li W, Deng W, Fan X, et al. Low toxicity antisolvent synthesis of composition-tunable luminescent all-inorganic perovskite nanocrystals. Ceram Int. 2018;44(15):18123–18128.
- Xu L, Chen J, Song J, et al. Double-protected all-inorganic perovskite nanocrystals by crystalline matrix and silica for triple-modal anti-counterfeiting codes. ACS Appl Mater Inter. 2017;9(31):26556–26564.
- Lou S, Xuan T, Liang Q, et al. Controllable and facile synthesis of CsPbBr3-Cs4PbBr6 perovskite composites in pure polar solvent. J Colloid Interf Sci. 2018;10:384–388.
- Wang B, Zhang C, Huang S, et al. Postsynthesis phase transformation for CsPbBr3/Rb4PbBr6 core/shell nanocrystals with exceptional photostability. ACS Appl Mater Inter. 2018;11(15):23303–23310.
- Nguyen TP, Ozturk A, Park J, et al. Facile synthesis of CsPbBr3/PbSe composite clusters. Sci Technol Adv Mat. 2016;138(45):10–17.
- Chen W, Hao J, Hu W, et al. Enhanced stability and tunable photoluminescence in perovskite CsPbX3/ZnS quantum dot heterostructure. Small. 2017;13(21):1604085.
- Pathak S, Sakai N, Wisnivesky Rocca Rivarola F, et al. Perovskite crystals for tunable white light emission. Chem Mater. 2017;29(27):8066–8075.
- Li X, Wu Y, Zhang S, et al. CsPbX3 quantum dots for lighting and displays: room-temperature synthesis, photoluminescence superiorities, underlying origins and white light-emitting diodes. Adv Funct Mater. 2018;19(1):2435–2445.
- Hai J, Li H, Zhao Y, et al. Designing of blue, green, and red CsPbX3 perovskite-codoped flexible films with water resistant property and elimination of anion-exchange for tunable white light emission. Chem Commun (Camb). 2017;53(39):5400–5403.
- Ren J, Li T, Zhou X, et al. Encapsulating all-inorganic perovskite quantum dots into mesoporous metal organic frameworks with significantly enhanced stability for optoelectronic applications. Chem Eng J. 2017;53:30–39.
- Zhang M, Wang M, Yang Z, et al. Preparation of all-inorganic perovskite quantum dots-polymer composite for white LEDs application. J Alloy Compd. 2018;748:537–545.
- Di X, Jiang J, Hu Z, et al. Stable and brightly luminescent all-inorganic cesium lead halide perovskite quantum dots coated with mesoporous silica for warm WLED. Dyes Pigm. 2017;146:361–367.
- Zhang L, Yang X, Jiang Q, et al. Ultra-bright and highly efficient inorganic based perovskite light-emitting diodes. Nat Commun. 2017;8(1):15640.
- Yang X, Zhang X, Deng J, et al. Efficient green light-emitting diodes based on quasi-two-dimensional composition and phase engineered perovskite with surface passivation. Nat Commun. 2018;9(1):570.
- Huang S, Li Z, Kong L, et al. Enhancing the stability of CH3NH3PbBr3 quantum dots by embedding in silica spheres derived from tetramethyl orthosilicate in “Waterless” toluene. J Am Chem Soc. 2016;138(18):5749–5752.
- Liu L, Li J, McLeod JA. Influence of Eu-substitution on luminescent CH3NH3PbBr3 quantum dots. Nanoscale. 2018;10(24):11452–11459.
- Yuan R, Shen L, Shen C, et al. CsPbBr3:xEu3+ perovskite QD borosilicate glass: a new member of the luminescent material family. Chem Commun. 2015;27(23):3395–3398.
- Kim H-S, Lee J-W, Yantara N, et al. High efficiency solid-state sensitized solar cell-based on submicrometer rutile TiO2 nanorod and CH3NH3PbI3 perovskite sensitizer. Nano Lett. 2013;13(6):2412–2417.
- Tan H, Jain A, Voznyy O, et al. Efficient and stable solution-processed planar perovskite solar cells via contact passivation. Science. 2017;355(6326):722.
- Scheidt RA, Kerns E, Kamat PV. Interfacial charge transfer between excited CsPbBr3 nanocrystals and TiO2: charge injection versus photodegradation. J Phys Chem Lett. 2018;9(20):5962–5969.
- Hultqvist A, Aitola K, Sveinbjörnsson K, et al. Atomic layer deposition of electron selective SnOx and ZnO films on mixed halide perovskite: compatibility and performance. ACS Appl Mater Inter. 2017;9(35):29707–29716.
- Yan S, Li Q, Zhang X, et al. A vertical structure photodetector based on all-inorganic perovskite quantum dots. J Soc Inf Display. 2019;28(1):9–15.
- Zhou L, Yu K, Yang F, et al. All-inorganic perovskite quantum dot/mesoporous TiO2 composite-based photodetectors with enhanced performance. Dalton T. 2017;46(6):1766–1769.
- Liu F, Zhang Y, Ding C, et al. Ultrafast electron injection from photoexcited perovskite CsPbI3 QDs into TiO2 nanoparticles with injection efficiency near 99. J Phys Chem Lett. 2018;9(2):294–297.
- Zhang G, Liu G, Wang L, et al. Inorganic perovskite photocatalysts for solar energy utilization. Chem Soc Rev. 2016;45(21):5951–5984.
- Zhou H, Ding L, Fan T, et al. Leaf-inspired hierarchical porous CdS/Au/N-TiO2 heterostructures for visible light photocatalytic hydrogen evolution. Appl Catal B-Environ. 2014;147:221–228.
- He M, Pang X, Liu X, et al. Monodisperse dual-functional upconversion nanoparticles enabled near-infrared organolead halide perovskite solar cells. Angew Chem Int Edit. 2016;55(13):4280–4284.
- Kazim S, Nazeeruddin MK, Grätzel M, et al. Perovskite as light harvester: a game changer in photovoltaics. Angewandte Chemie. 2014;53(11):2812–2824.
- Hou J, Cao S, Wu Y, et al. Inorganic colloidal perovskite quantum dots for robust solar CO2 reduction. Chem Eur J. 2017;23(40):9481–9485.
- Dotan H, Sivula K, Grätzel M, et al. Probing the photoelectrochemical properties of hematite (α-Fe2O3) electrodes using hydrogen peroxide as a hole scavenger. Energ Environ Sci. 2011;4(3):958–964.
- Man O, Wenguang T, Shengming Y, et al. Amino-assisted anchoring of CsPbBr3 perovskite quantum dots on porous g-C3N4 for enhanced photocatalytic CO2 reduction. Angewandte Chemie. 2018;130(41):13758–13762.
- Wu L-Y, Mu Y-F, Guo X-X, et al. Encapsulating perovskite quantum dots in iron-based metal–organic frameworks (MOFs) for efficient photocatalytic CO2 reduction. Angew Chem Int Edit. 2019;58(28):9491–9495.
- Wan S, Ou M, Zhong Q, et al. Perovskite-type CsPbBr3 quantum dots/UiO-66(NH2) nanojunction as efficient visible-light-driven photocatalyst for CO2 reduction. Chem Eng J. 2019;358:1287–1295.
- Sanehira EM, Marshall AR, Christians JA, et al. Enhanced mobility CsPbI3 quantum dot arrays for record-efficiency, high-voltage photovoltaic cells. Sci Adv. 2017;3(10):eaao4204.
- Zhao X, Yan G, Sun Y, et al. Preparation of ethyl cellulose composite film with down conversion luminescence properties by doping perovskite quantum dots. ChemistrySelect. 2019;4(21):6516–6523.
- Meinardi F, Akkerman QA, Bruni F, et al. Doped halide perovskite nanocrystals for reabsorption-free luminescent solar concentrators. ACS Energy Lett. 2017;2(10):2368–2377.
- Benick J, Richter A, Muller R, et al. High-efficiency n-type HP mc silicon solar cells. IEEE J Photovolt. 2017;7(5):1171–1175.
- Lee K-T, Guo JL, Park JH. Neutral- and multi-colored semitransparent perovskite solar cells. Molecules. 2016;21(4):475.
- Mao J-Y, Zhou L, Zhu X, et al. Photonic memristor for future computing: a perspective. Adv Opt Mater. 2019;7(22):1900766.
- An H, Kim WK, Wu C, et al. Highly-stable memristive devices based on poly(methylmethacrylate): CsPbCl3 perovskite quantum dot hybrid nanocomposites. Org Electron. 2018;56:41–45.
- Wang K, Dai S, Zhao Y, et al. Light-stimulated synaptic transistors fabricated by a facile solution process based on inorganic perovskite quantum dots and organic semiconductors. Small. 2019;15(11):e1900010.
- Wang Y, Lv Z, Liao Q, et al. Synergies of electrochemical metallization and valance change in all-inorganic perovskite quantum dots for resistive switching. Adv Mater. 2018;30(28):1800327.
- Wang Y, Lv Z, Chen J, et al. Photonic synapses based on inorganic perovskite quantum dots for neuromorphic computing. Adv Mater. 2018;30(38):1802883.
- Wu Y, Wei Y, Huang Y, et al. Capping CsPbBr3 with ZnO to improve performance and stability of perovskite memristors. Nano Res. 2017;10(5):1584–1594.
- Wu H, Zhang W, Wu J, et al. A visual solar UV sensor based on paraffin-perovskite quantum dot composite film. ACS Appl Mater Interfaces. 2019;11(18):16713–16719.
- Yu H, Lu Y, Feng Z, et al. A MAPbBr3: poly(ethyleneoxide) composite perovskite quantum dot emission layer: enhanced film stability, coverage and device performance. Nanoscale. 2019;11(18):9103–9114.