ABSTRACT
Background
Major Depressive Disorder (MDD) is a prevalent and debilitating condition, necessitating novel therapeutic strategies due to the limited efficacy and adverse effects of current treatments. We explored how galanin receptor 2 (GALR2) and Neuropeptide Y1 Receptor (NPYY1R) agonists, working together, can boost brain cell growth and increase antidepressant-like effects in rats. This suggests new ways to treat Major Depressive Disorder (MDD).
Research Design and Methods
In a controlled laboratory setting, adult naive Sprague-Dawley rats were administered directly into the brain’s ventricles, a method known as intracerebroventricular (ICV) administration, with GALR2 agonist (M1145), NPYY1R agonist, both, or in combination with a GALR2 antagonist (M871). Main outcome measures included long-term neuronal survival, differentiation, and behavioral.
Results
Co-administration of M1145 and NPYY1R agonist significantly enhanced neuronal survival and maturation in the ventral dentate gyrus, with a notable increase in Brain-Derived Neurotrophic Factor (BDNF) expression. This neurogenic effect was associated with an antidepressant-like effect, an outcome partially reversed by M871.
Conclusions
GALR2 and NPYY1R agonists jointly promote hippocampal neurogenesis and exert antidepressant-like effects in rats without adverse outcomes, highlighting their therapeutic potential for MDD. The study’s reliance on an animal model and intracerebroventricular delivery warrants further clinical exploration to confirm these promising results.
1. Introduction
Depression, a common mental health issue, arises from complex interactions among biological, psychological, and environmental factors [Citation1–3]. Affecting upwards of 300 million people worldwide, it constitutes about 4.4% of the global populace, as indicated by the World Health Organization and corroborated by the Global Burden of Disease Study [Citation4,Citation5]. The underlying biological processes contributing to depression include, but are not limited to, alterations in monoamine levels, dysregulation of the hypothalamic-pituitary-adrenal (HPA) axis, changes in synaptic structures, inflammation, and neurogenesis, with hippocampal neurogenesis being particularly significant [Citation6].
The hippocampus, a critical area of the brain for learning, memory, and mood regulation, often appears smaller in people with depression, as shown by MRI (Magnetic Resonance Imaging) scans [Citation7–12]. This atrophy is mirrored at the cellular level, where reductions in mature granule cells and the granular layer’s volume have been observed in MDD patients [Citation13]. The dentate gyrus (DG), central to neurogenesis, is crucial for integrating new neurons into existing circuits, supporting cognitive functions and stress resilience – a process compromised in depression, potentially exacerbating the disorder [Citation14–22]. Notably, the ventral DG’s response to emotional stimuli and its role in stress modulation highlight the therapeutic potential of targeting neurogenesis. Studies have shown that antidepressants can stimulate neural progenitor proliferation in the DG, offering a pathway to mitigate depression by enhancing hippocampal neurogenesis [Citation23–34].
However, the efficacy of antidepressants targeting monoaminergic systems remains limited, prompting the exploration of alternative therapeutic approaches given the high failure rate of new antidepressant drugs in clinical trials [Citation35]. Emerging research has begun to pivot from monoamine-based models to investigating the roles of neuropeptides like neuropeptide Y (NPY) and neurotrophic factors such as Brain-Derived Neurotrophic Factor (BDNF) in mood regulation [Citation34,Citation36–38]. Neuropeptide Y (NPY), a peptide containing 36 amino acid residues with a broad distribution in the brain, plays multifaceted roles in emotional regulation, neuroprotection, and stress modulation, particularly within key regions such as the amygdala and hippocampus [Citation39–42]. Its significance in mood disorders is evidenced by altered NPY1 receptor (NPY1R) levels in depression models and its capacity to mitigate depressive behaviors and enhance BDNF levels, indicating a crucial role in mood regulation and neurogenesis [Citation43–48]. These findings highlight the potential of targeting NPY and NPY1R pathways for developing novel antidepressant strategies.
Galanin (GAL), akin to Neuropeptide Y, plays a significant role in the central nervous system, influencing mood regulation through its receptors distributed in brain regions like the ventral CA1 and the dentate gyrus (DG) [Citation49–51]. The activation of GAL2 receptors (GALR2), in particular, has been associated with antidepressant-like effects in experimental models, underscoring GAL’s therapeutic potential for mood disorders. Experimental evidence suggests that GAL’s modulation of its receptors can influence depressive behaviors, with GALR2 activation linked to positive antidepressant outcomes and GalR2-knockout models displaying depression-consistent behaviors. Recent developments, including a GALR2 agonist, have further highlighted GAL’s promise in depression therapy [Citation52–57].
Emerging clinical evidence indicates that alterations in NPY and GAL receptors are associated with depression and suicidal behavior, underlining the therapeutic potential of targeting these neuropeptide systems in the context of stress-induced mood disorders and emphasizing the significance of exploring the NPY-GAL interaction for novel depression treatments [Citation58,Citation59]. Building upon these insights, extensive research has explored the intricate interplay between NPY and GAL, particularly focusing on the potential formation of NPY1R-GALR2 heteroreceptor complexes in several brain regions, including the amygdala, hippocampus, hypothalamic areas, and the medial prefrontal cortex [Citation60–66]. The intricate interplay between the signaling pathways of NPY and GAL, and the possibility of these neuropeptides forming heteroreceptor complexes, offers a groundbreaking avenue for addressing the complex nature of depression. By exploring the synergistic effects of NPY1R and GALR2 agonists, our research aims to unveil novel mechanisms of action for antidepressant therapies, potentially overcoming the drawbacks of existing treatments. This focus not only highlights the innovative approach of our study but also emphasizes its potential to contribute significantly to the development of targeted, more effective antidepressant strategies.
Building upon the recognition of the limited efficacy and adverse effects associated with current antidepressants, this study seeks to explore novel therapeutic strategies for Major Depressive Disorder (MDD). Specifically, our aim is to investigate the synergistic effects of NPY1R and GALR2 agonists on hippocampal neurogenesis and their influence on antidepressant-like effects in an experimental model. By focusing on these neuropeptide receptors’ roles within the ventral hippocampus, we propose a potentially groundbreaking approach to addressing the complexities of MDD. This study does not only aim to enhance our understanding of the neurobiological bases of depression but also to identify innovative targets for the development of antidepressant therapies. Ultimately, our research attempts to contribute to the advancement of treatments capable of more effectively managing the multifaceted symptoms of depression, potentially reducing the reliance on current treatments with known limitations and side effects.
2. Materials and methods
2.1. Animal housing, care, and experimental design
Male Sprague-Dawley rats, sourced from CRIFFA, Barcelona, weighing between 200 and 250 grams and aged 6–8 weeks, were housed in large group cages, accommodating six animals each, to promote social interaction and environmental enrichment. To enhance their environmental complexity and stimulate natural behaviors, each cage was supplemented with paper shavings and cardboard tubes, allowing the rats to burrow and explore. These enrichments were designed to improve the well-being and physiological robustness of the animals, potentially reducing stress-induced variability in experimental outcomes. The housing conditions maintained a strict 12-hour light/dark cycle, with environmental parameters such as relative humidity (55–60%) and temperature (22 ± 2°C) carefully controlled. Behavioral experiments were conducted between 09:00 and 14:00 hours. All animal care and experimental protocols were conducted in strict adherence to the EU Directive 2010/63/EU and Spanish legislation (Real Decreto 53/2013), with approval from the University of Málaga’s Local Committee for the Ethics of Animal Experimentation, Spain (CEUMA 45–2022-A).
Animals were randomly assigned to their respective experimental groups using the drawing lots method. This randomization process was crucial for minimizing selection bias and ensuring the integrity of our experimental design. By randomly allocating animals to each group, we aimed to evenly distribute any inherent biological variability among the experimental conditions, thus enhancing the reliability and validity of our findings.
To ensure unbiased observation and analysis, blinding was implemented during the assessment phase. Individuals analyzing the data were not involved in the experimental procedures and were unaware of the group allocations. Furthermore, the names of the animal groups were coded, concealing their identity from the analysts. This blinding strategy was crucial for eliminating observer bias, allowing for objective assessment and interpretation of the behavioral and physiological outcomes.
2.2. Preparation of drugs
Peptides were freshly prepared by dilution in artificial cerebrospinal fluid (aCSF), composed of 120 mM NaCl, 20 mM NaH2CO3, 2 mM KCl, 0.5 mM KH2PO4, 1.2 mM CaCl2, 1.8 mM MgCl2, 0.5 mM Na2SO4, and 5.8 mM D-glucose, adjusted to a pH of 7.4. This solution served as the vehicle for control groups. Receptor-specific agonists and antagonists, including Galanin receptor 2 agonist (M1145), Y1R receptor agonist [Leu31, Pro34] NPY, and GALR2 Antagonist M871, were procured from Tocris Bioscience (Bristol, UK). Detailed methodologies for the intracerebroventricular (icv) administration of these peptides are elaborated in the Supplementary Material.
2.3. Experimental design
The experimental subjects were categorized into five groups: (1) control (aCSF), (2) treated with M1145 (3 nmol), (3) treated with [Leu31, Pro34] NPY (3 nmol), (4) a combination treatment of M1145 and Y1R agonist, and (5) a trio treatment involving M1145, [Leu31, Pro34]NPY, and the GALR2 antagonist M871 (3 nmol each), with four rats in each group. The treatment was administered once daily over a three-day period, aligning with established protocols that suggest the durability of NPY’s efficacy [Citation46,Citation67–71]. Behavioral and neurochemical assessments were conducted on separate sets of animals 3 weeks after the peptide administration. This timeline aligns with findings from Decressac’s study on the neurogenic effects of NPY1R [Citation69], along with other research, demonstrating that newborn neurons can start to functionally contribute to hippocampal activity in rats within 2–3 weeks [Citation15,Citation17,Citation18].
2.4. Evaluation of hippocampal cell survival
Rats received two intraperitoneal (i.p.) injections of 5′-Bromo-2′-deoxyuridine (BrdU, Sigma, St. Louis, MO, U.S.A.) dissolved in a sterile 0.9% NaCl solution at a concentration of 15 mg/mL, at a dosage of 50 mg/kg body weight every 2 hours over 3 days, starting at 9:00 AM. Three weeks post-icv treatment, animals were anesthetized with pentobarbital (Mebumal, 100 mg/kg, i.p.) and perfused with 4% paraformaldehyde. Brain tissues were sectioned at 30 μm through the ventral hippocampus, following protocols established in previous studies [Citation15,Citation69,Citation72,Citation73].
2.4.1. Immunohistochemistry
Brain sections were incubated free-floating in saline sodium citrate buffer (pH 6; 10 nM sodium citrate) for 90 min at 65°C, followed by 30 min with 0,6% H2O2 to remove endogenous peroxidases. After 30 min in 2 M hydrochloric acid (HCl) to denature deoxyribonucleic acid (DNA), sections were incubated for neutralization with 0.1 M sodium borate (pH 8). Then, slices were incubated at 4◦C overnight with a primary antibody against BrdU (Abcam, ab152095, 1:1000) in 2,5% donkey serum. Following additional washed with PBS and incubated with a secondary antibody for 90 min (biotinylated anti-rabbit IgG, 1/200, Vector Laboratories), sections were amplified with ExtrAvidin peroxidase (Sigma, St. Louis, MO, U.S.A.) diluted 1:100 in darkness at room temperature for 1 hour. Immunolabeling was exposed with 0.05% diaminobenzidine (DAB; Sigma) and 0.03% H2O2 in PBS. After various washes, sections were mounted on gelatin-coated slides, dehydrated in graded alcohols, and cover-slipped in DePeX mounting medium (Merck Life Science SLU, Darmstadt, Germany). BrdU-labeled cells with morphological characteristics of glial precursors, i.e. small, irregularly shaped cell bodies, were excluded. Only round, regularly shaped BrdU-positive nuclei located in the DG were counted since new granule cells migrate approximately 2 cell body widths from the SGZ into the granule layer [Citation46]. As previously described, BrdU-labeled cells were analyzed using the optical fractionator method in unbiased stereological microscopy (Olympus B×51Microscope, Olympus, Denmark) [Citation62,Citation63,Citation65] (see Supplementary Material for details).
2.4.2. Double immunofluorescence
Following established protocols, sections were blocked, permeabilized, and incubated with primary antibodies against BrdU and either DCX or NeuN [Citation60,Citation61,Citation63–65]. Briefly, an initial incubation with blocking (5% goat serum) and permeabilization (0.3% triton X100 in PBS) solutions was performed for 60 min each. Pair of primary antibodies rabbit anti-BrdU (Abcam, ab152095, 1:1000)/mouse anti-DCX (C-18, Santa Cruz, 1:500) or rabbit anti-BrdU (Abcam, ab152095, 1:1000)/mouse anti-NeuN (Abcam, ab1042241, 1:1000) were used to incubate the sections for 24 h, at 4°C. Then, incubations were performed with proper secondary antibodies: Donkey anti-mouse AlexaFluor 488 (Abcam, ab150105, 1:200) and Donkey anti-rabbit AlexaFluor 647 (Abcam, ab150075, 1:200). Sections were mounted on slides with a fluorescent mounting medium with 4,’6-diamidino-2-phenylindole (DAPI) to detect nuclei (Abcam, ab104139). BRDU/DCX- and BRDU/NeuN double-stained cells in the DG were quantified using z-scan confocal microscopy (Leica Stellaris 8) at 40X magnification. The entire length of the DG was assessed through the septo-temporal axis of the hippocampus, analyzing at least four representative 150 μm, evenly spaced sections per animal. All analyzes were conducted in sequential scanning mode to eliminate potential cross-bleeding between channels. Each cell was scrutinized using a multi-channel configuration, and colocalization with NeuN or GFAP was verified by examining multiple optical planes for each cell on the z-axis. Z-stacks were generated at 0.85 μm intervals throughout the 30 μm section to ensure accurate double-labeling of BrdU-IR cells [Citation63,Citation74].
2.5. Evaluation of brain-derived neurotrophic factor (BDNF) induction in the ventral dentate gyrus
Various free-floating sections were processed for antigen retrieval at 65°C for 90 minutes in saline sodium citrate buffer (pH 6; 10 nM sodium citrate), followed by a 30-minute treatment in 0.6% H2O2. Subsequently, slices were incubated overnight at room temperature with primary antibody rabbit anti-BDNF (Chemicon, Sigma-Aldrich, AB1534SP, 1:500) in 2.5% donkey serum. Post-PBS washes, slices were treated with biotinylated anti-rabbit IgG (1:200, B8895, Sigma, St. Louis, MO, U.S.A.) for 90 minutes and then with ExtrAvidin peroxidase (1:100, Sigma, St. Louis, MO, U.S.A.) in darkness for 1 hour. Detection was completed with 0.05% diaminobenzidine (DAB) and 0.03% H2O2 in PBS, followed by mounting on gelatin-coated slides and coverslipping with DePeX mounting medium (Merck Life Science SLU, Darmstadt, Germany). The BDNF-marked cells were then analyzed via optical fractionator methodology in unbiased stereological microscopy (Olympus B×51Microscope, Olympus, Denmark).
2.6. Hippocampal cell culture and conditions
Rat primary hippocampal neuronal cells were purchased from QBM Cell Science (Montreal, Canada) and cultured in Neuro basal medium supplemented with 10% FBS, 2 mM GlutaMAX-1, 1 mM sodium pyruvate, 100 U/ml penicillin G, and 100 μg/ml streptomycin and 2% B-27 supplement at 37°C in a humidified 10% CO2 environment according to manufacturer’s instructions. Half part of the medium was changed every 3 days. The cells were grown under the above conditions (control condition) for 7 days. Cultured hippocampal neurons were grown and treated for 14 days under specific pharmacologic conditions. Treated hippocampal cells were divided into experimental groups: (1) Control group; (2) M1145-treated group (100 nM); (3) Y1R agonist-treated group receiving an NPYY1R agonist [Leu31,Pro34]NPY (100 nM); (4) GAL+Y1R: Group treated with both substances; (5) GAL+Y1+M871: Group incubated with GAL, [Leu31,Pro34]NPY and the GALR2 antagonist (M871; 1 μM). Cells were grown on poly-D-lysine-coated glass coverslips and fixed with 4% formaldehyde solution for 20 min followed by two washes with PBS containing 20 mM glycine to quench the aldehyde groups.
2.7. Hippocampal neuronal cells’ viability analysis
The MTS assay determined cell viability in rat primary hippocampal neuronal cells using the CellTiter 96 Aqueous One Solution Cell Proliferation Assay kit (G3580, Promega, Madison, WI, U.S.A.). This assay determines the levels of cellular 3-[4,5-dimethylthiazol-2-yl-5-(3-carboxymethoxyphenyl)-2-(4-sulfo-phenyl)-2 H tetrazolium, inner salt] (MTS) reduction to formazan, a measure of mitochondrial function. Cultured hippocampal neurons (7 days in vitro) were collected, and approximately 2 × 104 cells per well were plated into 96-well plates, which were treated for 0, 3, 6, and 9 days, respectively in B27-deprived medium, and then 20 µL of MTS solution was added to each well. Plates were incubated at 37°C for 3 h. The absorbance was determined with a POLARstar Optima plate reader (BMG Labtech) at 490 nm, directly proportional to the number of living cells in the culture. This protocol was performed as described in [Citation63,Citation75].
2.8. Analysis of neurite length
A different set of cultured hippocampal neurons was grown under the specific pharmacologic conditions described above. Cells were grown on poly-D-lysine-coated glass coverslips and fixed with 4% formaldehyde solution for 20 min followed by two washes with PBS containing 20 mM glycine to quench the aldehyde groups. Then, after permeabilization with PBS containing 0.2% Triton X-100 for 5 min, cells were treated with PBS containing 1% bovine serum albumin. Cells were labeled with Neuro-Chrom Pan Neuronal Marker primary antibody (ABN2300, 1:100, Sigma-Aldrich; Merck Life Science S.L.U.) for 1 h, extensively washed, and stained with the green fluorescence secondary antibody goat anti-rabbit DyLight 488 (Jackson Laboratories InmunoResearch, 1:100). Cell nuclei were counterstained with DAPI (blue) contained in the mounting medium. Acquisition of microscopy images and morphometric quantifications were performed as described [Citation76,Citation77].
2.9. Behavioral assessment of antidepressant-like effects
The antidepressant-like effect elicited by the administration of the Forced Swim Test (FST), a behavioral test for assessing despair-like behavior in rodents, often used in antidepressant screening [Citation78–80].
Behavioral experiments were conducted between 09:00 and 14:00 hours, three-week post-administration. Naive rats were acclimatized to handling and the experimental environment before being randomly assigned to the experimental groups. The Open-Field Test (OFT) was used to determine eligibility for the FST. Rats were individually placed and allowed to freely explore the behavioral apparatus over a 10 minutes period. Locomotor parameter of distance moved was analyzed using the video tracking software Smart2.5 (Panlab, SL). After each trial, all surfaces were cleaned with 70% ethanol solution [Citation72,Citation81–83]. In the current study, the OFT was conducted followed by the Forced Swim Test (FST) on two consecutive days [Citation72,Citation81,Citation82]. To address potential confounding variables associated with the sensitization procedure, the animals were subjected to the Forced Swim Test (FST) a single time [Citation84]. The FST involved a 10-minute swim in a cylinder filled with water at 25 ± 0.2°C. Immobility, swimming and climbing behaviors were recorded and analyzed using Raton Time 1.0 software (Fixma S.L., Valencia, Spain). Immobility time was recorded when the animal made minimum movements necessary to keep its body afloat. Swimming time was regarded as the time when an animal performed an active swimming activity beyond the necessary movements to keep itself floating, while climbing was defined as vigorous forepaw movements directed toward the walls of the cylinder. Depression-related behavior was inferred from an increase in the time the rat spent immobile, which is thought to represent a lack of motivation to escape from the water. Post-test, rats were dried and returned to their home cages. Observers blinded to the experimental conditions conducted the behavioral experiments.
2.10. Statistical analysis
Data were analyzed with the assumption that each individual rat served as an experimental unit. Data were exhibited as mean ± SEM, with the number of samples denoted in the figure legends. GraphPad PRISM 8.0 (GraphPad Software, La Jolla, CA, U.S.A.) was harnessed for data examination. One-way ANOVA was conducted followed by the Newman-Keuls posttest for comparative analysis, or Student’s unpaired t-test where necessary. Significance thresholds were defined as *p < 0.05, **p < 0.01, ***p < 0.001.
3. Results
3.1. GALR2 and Y1R agonist co-injection promotes survival of newborn neurons in the ventral hippocampus
Our investigation into the cellular mechanisms behind GALR2 and Y1R agonist icv subacute co-administration on adult ventral hippocampal cell proliferation was performed using the thymidine analogue 5-Bromo-2’-deoxyuridine (BrdU)().
Figure 1. Cell survival analysis in the ventral dentate gyrus following intracerebroventricular co-administration of galanin receptor 2 and NPY1R.
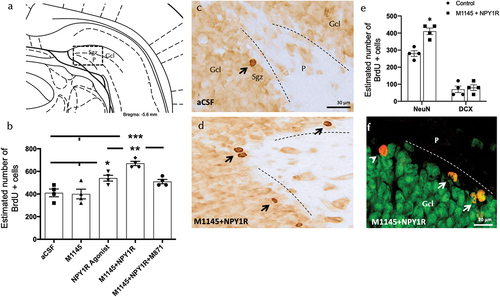
ICV co-administration of M1145 and the Y1R agonist significantly increased the number of BrdU-IR profiles in the subgranular zone (Sgz) of the dentate gyrus compared to the control group, as well as the M1145 and Y1R agonist alone groups (one-way ANOVA, F4,15 = 13.68, p < 0.001), with post-hoc tests indicating significant differences compared to control, M1145, and Y1R agonist groups (p < 0.001 against control and M1145; p < 0.01 against Y1R agonist) (). The addition of GALR2 antagonist M871 blocked this effect (p < 0.01 in post-hoc test), indicating the participation of GALR2 in the M1145/NPYY1R agonist interaction to stimulate cell survival. shows the increase in BrdU-positive cells in the subgranular zone, highlighting the increased survival rate three weeks after the treatments.
Moreover, the icv administration of the Y1R agonist alone induced an increase in the number of BrdU positive cells in the subgranular zone (Sgz) of the ventral hippocampus () compared with the control and M1145 groups (Newman-Keuls post-hoc test: p < 0.05) (). However, M1145 icv infused alone lacked effects on the numbers of BrdU-IR profiles () compared with the control group ().
3.2. Identification of cellular types affected by increased survival after M1145 and Y1R agonist administration
Additionally, we studied the cellular types affected by the icv infusion of M1145 and the Y1R agonist. Quantification of BrdU labeled cells coexpressing either doublecortin (DCX)-expressing neuroblasts or marker of mature neurons (NeuN)-expressing cells was performed (). Results revealed that the number of BrdU+/NeuN+ cells increased significantly after the combined Y1R-GALR2 agonists treatment compared to the control group (t-test, t = 3.153, df = 6; p < 0.05)) (), indicating a preference for newly generated cells to differentiate toward a neuronal lineage (mature neurons). No significant change was noted in BrdU+/DCX+ cells (t-test, t = 0.654, df = 6).
3.3. Association of increased survival of mature neurons with enhanced brain-derived neurotrophic factor (BDNF) through coactivation of M1145 and Y1R agonists
To explore the mechanisms associated with the rise in neuronal survival, we conducted an examination of BDNF expression within the ventral hippocampal dentate gyrus (DG) following the icv administration of either M1145 or Y1R agonist. We detected BDNF-positive cells specifically localized in the granular region of the ventral hippocampus, along with sporadic cells in the polymorphic layer (P) of the ventral DG (). Through stereological quantification, we found a notable increase in the BDNF positive cells subsequent to the icv combined administration of M1145 and NPY1R agonist, when compared to control (one-way ANOVA, F4, 15 = 12.39, p < 0.001, Newman-Keuls post-hoc test: p < 0.001), M1145 (Newman-Keuls post-hoc test: p < 0.001) or NPY1R1 agonist alone (Newman-Keuls post-hoc test: p < 0.05) (). In alignment with the observations mentioned earlier, the presence of GALR2 antagonist M871 fully suppressed the increase prompted by the icv combined injection (Newman-Keuls post-hoc test: p < 0.05) (), highlighting GALR2’s role in this interaction.
Figure 2. M1145 and the Y1R agonist effects on hippocampal brain-derived neurotrophic factor immunoreactive (BDNF-IR) cells of the ventral dentate gyrus (DG) hippocampal region.
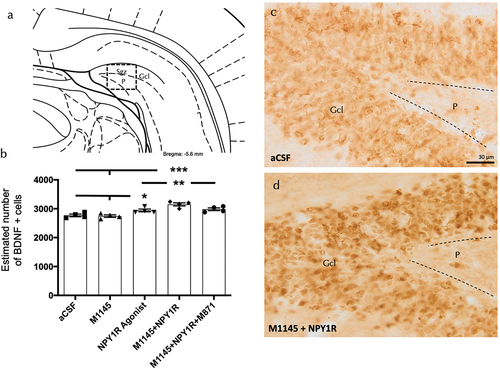
Furthermore, the icv administration of the Y1R agonist alone induced an increase in the number of BDNF positive cells in the granular zone of the ventral hippocampus () compared with the control and M1145 groups (Newman-Keuls post-hoc test: p < 0.05) (). Conversely, the icv infusion of M1145 agonist alone showed no impact on the count of BDNF-positive cells in the ventral DG.
3.4. Enhancing effect of coadministration of GALR2 and the NPY1R agonists on survival and length of neurite processes
To detect overall neuronal viability modulating effects, the effects of the GALR2 agonist M1145 and/or the NPY1R agonist were monitored in an MTS cell proliferation assay after incubating cells with each substance in a time-course manner during 9 days. The results of these experiments are summarized in . Upon incubation with M1145 and the NPY1R agonist, hippocampal neurons’ viability was stable and resulted in an increased rate of survival at 9 days, as compared with the control (t = 2.535, df = 14; p < 0.05), M1145 (t = 2.765, df = 14; p < 0.05), and NPY1R agonist (t = 2.649, df = 14; p < 0.05) groups (). Moreover, the specific GALR2 antagonist M871 abolished this effect (t = 2.755, df = 14; p < 0.05) (), demonstrating that this effect was mediated through the coactivation of GALR2 and NPYY1R. These results indicate that the co-incubation of the GALR2 agonist and the NPY1R agonist in solution exerts a significant effect on the survival of neurons. The incubation with the NPY1R agonist alone revealed a non-significant tend to increased neuronal viability. Moreover, incubation with M1145 alone revealed a minor influence on neuronal viability compared to the control.
Figure 3. Evaluation of survival and neurite outgrowth on hippocampal neuronal cells.
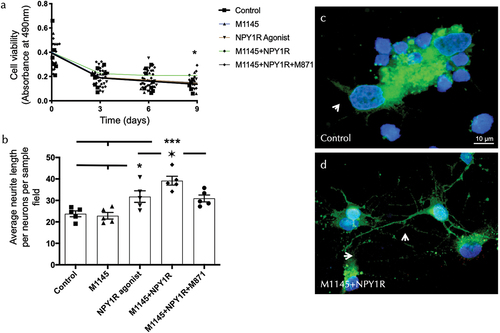
Due to the changes observed in the survival of hippocampal cells upon treatments, we decided to study the morphology and structural plasticity changes. The data show a significant synergistic increase of mean neurite length upon coactivation of M145 and the Y1R agonist compared to the NPY1R agonist group alone (one-way ANOVA, F4, 20 = 12.18, p < 0.001, Newman-Keuls post-hoc test: p < 0.05), control (Newman-Keuls post-hoc test: p < 0.001) and M1145 alone (Newman-Keuls post-hoc test: p < 0.001) (). Furthermore, the presence of GALR2 antagonist M871 entirely blocks the synergic effects (Newman-Keuls post-hoc test: p < 0.05) (). The Y1R agonist incubated alone increased the neurite length compared to control and M1145 alone (Newman-Keuls post-hoc test: p < 0.05) ().
3.5. Enhanced antidepressant-like response induced by GALR2 and Y1R agonists in the forced swim test
In the Forced swim Test (FST), we assessed the functional outcomes following icv delivery of GALR2 and NPY1R agonists. Rats underwent a 10-minute FST session 3 weeks icv post-administration. The analysis of immobility and swimming behaviors revealed significant changes. Specifically, coadministration of M1145 and Y1R agonists resulted in a marked decrease in immobility time (one-way ANOVA, F4,25 = 10.12, p < 0.001; ) compared to the NPY1R agonist group alone (Newman-Keuls post-hoc test: p < 0.05), control (Newman-Keuls post-hoc test: p < 0.001) and M1145 alone (Newman-Keuls post-hoc test: p < 0.001). Enhanced swimming activity of M1145 and Y1R agonists icv combination (one-way ANOVA, F4,25 = 11.86, p < 0.001; ) compared to the NPY1R agonist group alone (Newman-Keuls post-hoc test: p < 0.05), control (Newman-Keuls post-hoc test: p < 0.001) and M1145 alone (Newman-Keuls post-hoc test: p < 0.001) was also observed. The addition of GALR2 antagonist M871 counteracted these effects, as indicated by the post-hoc tests (p < 0.05 for both immobility and swimming). illustrates the significant reduction in immobility time in the FST, while shows the corresponding increase in swimming behavior following treatment.
Figure 4. Behavioral responses to combined GALR2 and NPY Y1 receptor agonists in the forced swim test (FST).
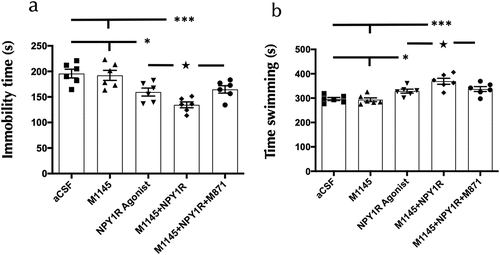
The icv infusion of the NPY1R agonist alone decreased the time of immobility compared to the control (Newman-Keuls post-hoc test: p < 0,05; ) and the M1145 (Newman-Keuls post-hoc test: p < 0,05; ) groups; while induced an increase in the swimming behavior compared to the control (Newman-Keuls post-hoc test: p < 0,05; ) and the M1145 (Newman-Keuls post-hoc test: p < 0,05; ) groups. However, the administration of the GalR2 agonist M1145 alone lacked effects on the FST () compared with the control group. No differences were observed between groups in climbing time on the FST (Supplementary Table S1). Moreover, no differences were observed between groups in locomotor activity on the open field test (Supplementary Table S2).
4. Discussion
Our research sheds light on how GALR2 and NPY1R agonists work together to boost cell survival, growth, and BDNF levels in the brain, offering new hope for treating depression. This research not only corroborates the pivotal role of neurogenesis in mood regulation but also highlights the therapeutic potential of targeting specific neuropeptide receptors to enhance neurogenic processes and alleviate depressive symptoms. The elevation of BDNF, a key factor in neuronal survival and differentiation, highlights a common mechanistic pathway that may underlie the antidepressant effects of diverse treatments, from physical exercise to pharmacological interventions. By comparing these outcomes with existing literature, our findings suggest that the co-activation of GALR2 and NPY1R offers a novel approach to modulate the neurobiological underpinnings of depression, potentially overcoming limitations of current antidepressant therapies. This critical analysis not only situates our work within the broader scientific dialogue but also proposes a direction for future research to explore the clinical applicability of these findings.
Our research contributes valuable insights into the beneficial effects of combined intracerebroventricular administration of GALR2 and NPY1R agonists on neuronal survival and maturation within the dentate gyrus of the ventral hippocampus. Having outlined the specific impacts of GALR2 and NPY1R agonist co-activation on neuronal survival and differentiation, it’s crucial to contextualize these findings within the larger framework of neurogenesis and its role in depression. This leap from micro to macro-level understanding allows us to appreciate the potential of targeting neurogenesis as a therapeutic strategy for mood disorders. In our study, the intracerebroventricular (ICV) co-administration of M1145 and the Y1R agonist led to a significant increase in the number of BrdU-immunoreactive (BrdU-IR) profiles within the subgranular zone of the dentate gyrus. The magnitude of this change, and the observed reversal of this effect by the GALR2 antagonist M871, emphasize the specific contribution of GALR2 in synergy with NPY1R to promote neuronal survival. This increase aligns with findings that, by the third week post-administration, a significant number of neurons not only survive but also actively contribute to hippocampal functions in rodent models [Citation15]. Moreover, our findings demonstrated a significant increase in the number of cells co-expressing BrdU and the neuronal nuclear protein NeuN, a marker of mature neurons, following combined treatment. This signifies not just an increase in neuronal survival but also a progression toward neuronal maturation, indicating that these neuropeptide agonists foster an environment conducive to neuronal differentiation. This observation of increased BrdU+/NeuN+ cells after combined agonist treatment compared to the control group highlights the substantial nature of neurogenic enhancement and neuronal maturation prompted by the treatment. This trend is consistent with other studies that have highlighted NeuN’s role as a marker for mature neurons, visible three weeks following such interventions [Citation86]. Yet, our findings also reflect lower counts of cells co-expressing BrdU and doublecortin (DCX), a marker for neuronal precursors, at the three-week mark, corroborating earlier observations [Citation15,Citation87]. The potential mechanisms underlying these remarkable changes may involve the upregulation of Brain-Derived Neurotrophic Factor (BDNF), a key player in supporting neurogenesis and neuronal survival.
Building upon the understanding of neurogenesis in depression, our findings invite comparisons with other compounds known for their neurogenic and antidepressant properties. Such comparisons not only highlight the unique contributions of our study but also frame our results within the broader pursuit of effective depression treatments. Intriguingly, recent research has highlighted the potential of enhancing neurogenesis within the ventral hippocampal DG to reinforce resilience against depressive states [Citation88]. Compounds like P7C3 have been shown to augment cell proliferation in the hippocampal DG, correlating with antidepressant effects across both rodent and primate studies. The neuroprotective effects of P7C3 are believed to stem from its ability to safeguard mitochondrial membrane integrity, a critical factor in maintaining cellular energy balance and preventing apoptosis. While the precise molecular target of P7C3 remains to be fully elucidated, research in rodent models and cellular systems has demonstrated that P7C3 effectively increases neuronal NAD flux under conditions that would otherwise lead to cell death [Citation89,Citation90]. While the exploration of other compounds provides valuable context, the enhanced neuronal survival and BDNF elevation observed with the long-term co-activation of GALR2 and NPY1R highlight the potential of these receptors as targets for innovative antidepressant strategies. At the cellular level, the observed increases in hippocampal neuronal survival and differentiation may be mediated by elevated levels of Brain-Derived Neurotrophic Factor (BDNF) in the ventral hippocampal DG post GALR2 and NPY1R agonists treatment. BDNF plays a crucial role in enhancing neurogenesis, impacting both cell proliferation and survival, and is a pivotal mediator in the neuroprotective effects of antidepressants [Citation91–93]. Physical activity, for instance, has been shown to mitigate depressive symptoms by augmenting hippocampal neurogenesis alongside BDNF levels [Citation94]. Conversely, reductions in hippocampal BDNF levels have been reported in postmortem analyzes of MDD sufferers and suicide victims [Citation95,Citation96], while BDNF injections into the hippocampus have demonstrated a capacity to alleviate depression-like behaviors in rodent models [Citation97]. Our findings are in line with prior evidence suggesting NPY’s BDNF-mediated neuroprotective effect in neurodegeneration models [Citation46,Citation98,Citation99]. Moreover, physical exercise has been shown to enhance BDNF signaling and neuronal proliferation in the ventral hippocampus, correlating with antidepressant effects [Citation94].
The rationale behind conducting experiments on hippocampal cell viability and neurite length, and the subsequent findings, form a pivotal aspect of our study, emphasizing the therapeutic potential of GALR2 and NPY1R agonists. These investigations were designed to explore not only the neurogenic effects of these agonists but also their impact on the structural and functional maturation of neurons, a critical dimension in understanding antidepressant mechanisms. Enhanced cell viability in B27-deprived conditions indicates a robust neuroprotective effect, suggesting that the treatments could counteract stress-induced cellular vulnerability, a hallmark of depressive states. Similarly, the observed increase in neurite length aligns with the enhancement of neuronal connectivity and network integration, essential for the restoration of normal hippocampal function implicated in mood regulation. These cellular outcomes, potentially mediated by BDNF highlight a mechanistic pathway through which the combined agonist treatment exerts its effects [Citation100,Citation101]. By examining these parameters, our study provides a comprehensive insight into how GALR2 and NPY1R agonists promote neuronal health and connectivity, offering a foundation for their antidepressant-like efficacy observed in behavioral assays.
Functionally, the antidepressant-like effects of the GALR2 and NPY1R agonist combination were validated using the forced swim test (FST), according with findings that implicate the ventral hippocampus in the antidepressant efficacy of NPY in models of posttraumatic stress disorder [Citation102]. This observation highlights the therapeutic potential of modulating specific neuropeptide receptors, such as GALR2 and NPY1R, in eliciting antidepressant responses. Similarly, treatments like NPY and NPY1R agonists have shown promise in inducing antidepressant effects across various species, reinforcing the value of targeting these pathways [Citation70,Citation103,Citation104]. In our study, the differential increase in swimming behavior observed in the rat Forced Swim Test suggests that the scoring of swimming time can provide valuable insights into the underlying mechanisms mediating antidepressant-like effects. Specifically, akin to the action of selective 5-HT reuptake inhibitors, the decrease in immobility and concurrent increase in swimming behavior observed could indicate a serotonergic mechanism at play, underlining the complexity of neurochemical interactions involved in modulating antidepressant-like effects [Citation105]. It is pertinent to note the absence of locomotor alterations elicited by the treatments. This observation is critical as it underscores that the observed antidepressant-like effects are not confounded by changes in general activity levels, thus providing a clearer interpretation of the specific therapeutic potential of the treatments without the influence of altered locomotion.
While our findings illuminate the antidepressant-like effects of the NPY1R and GALR2 agonist combination, it is essential to weave this narrative into a broader context that acknowledges species-specific responses to these treatments. Research employing intranasal GALR2 agonist treatments in mice has unveiled critical insights into the nature of antidepressant efficacy across different species [Citation57,Citation106]. Furthermore, the exploration of stabilized GALR2 agonists like M39b opens new horizons for therapeutic interventions, demonstrating the evolving landscape of neuropeptide-based treatments [Citation107]. The promising therapeutic potential of these agonists not only aligns with our study’s outcomes but also propels the discussion toward the future of depression treatment. It underscores the importance of continuing to explore the intricate dynamics between neuropeptide receptors and their agonists, particularly in the context of developing more effective and targeted antidepressant therapies. By situating our study within these broader discussions, we emphasize the interconnectedness of our findings with ongoing research into the mechanisms of action and therapeutic applications of GALR2 agonists. This approach aims to highlight the significance of our results while acknowledging the complexity and diversity of research directions that stem from understanding the roles of GALR2 and NPY1R in depression treatment. Future research must delve into critical unanswered questions, such as the precise mechanisms of receptor interaction, optimal dosing strategies for maximal efficacy with minimal side effects, and the potential for synergistic effects with other therapeutic agents. Addressing these inquiries will be pivotal in translating our findings into viable clinical applications.
It is crucial to acknowledge certain limitations that might affect the interpretation and generalizability of our findings. First, the choice of animal model, while instrumental in elucidating the neurobiological underpinnings of antidepressant-like effects and the potential therapeutic effects of NPY1R and GALR2 agonists, may not fully capture the complexity of Major Depressive Disorder as it manifests in humans. Rodent models, despite their utility, inherently possess differences in brain structure and function that could influence the translation of these findings to clinical settings. Second, while our experimental design focused on the intracerebroventricular administration of the agonists, representing a highly controlled method of drug delivery, we acknowledge it might not directly correspond to methods available for human treatment, raising questions about the feasibility of translating these specific administration routes and dosages to clinical practice. However, exploring the potential of intranasal administration of these peptides presents a promising avenue for enhancing their translational value to clinical settings, offering a more direct and noninvasive route that could facilitate the therapeutic application of our findings. Additionally, while we have made efforts to minimize variability and ensure rigorous control within our experimental setup, the relatively small sample size and the exclusion of female subjects limit the breadth of our conclusions. The inclusion of a broader demographic, considering sex differences, could provide a more comprehensive understanding of the treatment effects across populations. Lastly, while our study highlights the promising therapeutic potential of co-activating GALR2 and NPY1R, the mechanisms underlying these effects warrant further investigation to fully understand their implications for depression therapy. These limitations highlight the need for continued research, including larger, more diverse studies and exploration into the mechanisms of action, to build upon our findings and advance toward clinically relevant applications. In our research, the utilization of naive animal models, which were not subjected to any specific depression-inducing procedures, was instrumental in shedding light on the potential pathophysiological mechanisms underlying mood disorders and the action mechanisms of antidepressant agents. This methodology holds particular significance in the investigation of subclinical symptoms and the exploration of the preventative capacities of therapeutic interventions. Previous studies leveraging naive models have been pivotal in enhancing our comprehension of the neurobiological pathways and behavioral dynamics related to mood regulation [Citation44,Citation108,Citation109]. These contributions highlight the value of employing naive models during the preliminary phases of research into new therapeutic targets and mechanisms. This foundational work sets the stage for further, more detailed investigations in models that closely mimic the complex pathology of depression, such as exposition to chronic unpredictable stress, corticosterone or LPS injection.
5. Conclusions
This study provides compelling evidence that the synergistic administration of Galanin Receptor 2 (GALR2) and Neuropeptide Y1 Receptor (NPYY1R) agonists facilitates hippocampal neurogenesis and induces antidepressant-like behaviors in a rodent model. The findings indicate a significant increase in neuronal survival and differentiation, alongside an upregulation of Brain-Derived Neurotrophic Factor (BDNF), suggesting a potential mechanistic pathway for the observed antidepressant effects. Importantly, these therapeutic effects were achieved without the report of adverse events, highlighting the safety profile of these treatments within the study’s scope.
The clinical implications of these results are substantial, offering a promising avenue for the development of new therapeutic strategies for Major Depressive Disorder (MDD). By targeting specific neural pathways involved in neurogenesis and mood regulation, GALR2 and NPYY1R agonists could represent a novel class of antidepressants with potentially fewer side effects compared to traditional treatments.
However, it is important to acknowledge that while the study’s outcomes are encouraging, they are derived from a preclinical model. The generalizability of these results to the broader patient population with MDD remains to be established through clinical trials. Further research is warranted to validate the efficacy and safety of GALR2 and NPYY1R agonists in humans, as well as to explore the long-term implications of their use in the treatment of depressive disorders.
Declaration of interest
The authors have no relevant affiliations or financial involvement with any organization or entity with a financial interest in or financial conflict with the subject matter or materials discussed in the manuscript. This includes employment, consultancies, honoraria, stock ownership or options, expert testimony, grants or patents received or pending, or royalties.
Reviewer disclosures
Peer reviewers on this manuscript have no relevant financial or other relationships to disclose.
Author contributions
D Borroto-Escuela and M Narváez played a pivotal role in the conception and design of the study. They were instrumental in formulating the research questions and setting up the experimental framework. P Serrano-Castro, JA Sánchez-Pérez, K Fuxe and MA Barbancho-Fernández contributed extensively to the analysis and interpretation of the data, applying their expertise to decipher the complex results and understand their implications. M Narváez was involved in drafting the manuscript, providing critical insights that enhanced the intellectual content of the paper. All authors participated in revising the manuscript critically, offering valuable feedback and suggestions that significantly improved the quality of the final submission. They also gave their final approval of the version to be published, ensuring that it accurately reflects their findings and conclusions.
Availability of data and materials
The data that support the findings of this study are openly available in the Institutional repository of the University of Malaga (RIUMA) and from the corresponding author upon reasonable request.
Ethics approval and consent to participate
All experimental protocols were approved by the Local Animal Ethics, Care, and Use Committee for the University of Málaga, Spain (CEUMA 45–2022-A), and conducted in accordance with the EU Directive 2010/63/EU and Spanish Directive (Real Decretory 53/2013).
Supplementary EOTT reviewed.docx
Download MS Word (31.3 KB)Supplementary material
Supplemental data for this article can be accessed online at https://doi.org/10.1080/14728222.2024.2342517
Additional information
Funding
References
- Catala-Lopez F, Genova-Maleras R, Vieta E, et al. The increasing burden of mental and neurological disorders. Eur Neuropsychopharmacol. 2013 Nov;23(11):1337–1339.
- McEwen BS, Bowles NP, Gray JD, et al. Mechanisms of stress in the brain. Nat Neurosci. 2015 Oct;18(10):1353–1363.
- Schramm E, Klein DN, Elsaesser M, et al. Review of dysthymia and persistent depressive disorder: history, correlates, and clinical implications. Lancet Psychiatry. 2020 Sep;7(9):801–812.
- World Health OFM. Depression and other common mental disorders. World Health Org. 2017. p. 2–2.
- Global Burden of Disease Cancer C, Fitzmaurice C, Akinyemiju TF, et al. Global, Regional, and National Cancer Incidence, Mortality, Years of Life Lost, Years Lived With Disability, and Disability-Adjusted Life-Years for 29 Cancer Groups, 1990 to 2016: A Systematic Analysis for the Global Burden of Disease Study. JAMA Oncol. 2018 Nov 1;4(11):1553-1568.
- Dean J, Keshavan M. The neurobiology of depression: an integrated view. Asian J Psychiatr. 2017 Jun;27:101–111. doi: 10.1016/j.ajp.2017.01.025
- Burgess N, Maguire EA, O’Keefe J. The human hippocampus and spatial and episodic memory. Neuron. 2002 Aug 15;35(4):625–641. doi: 10.1016/S0896-6273(02)00830-9
- Davidson RJ, Lewis DA, Alloy LB, et al. Neural and behavioral substrates of mood and mood regulation. Biol Psychiatry. 2002 Sep 15;52(6):478–502. doi: 10.1016/S0006-3223(02)01458-0
- Sahay A, Hen R. Adult hippocampal neurogenesis in depression. Nat Neurosci. 2007 Sep;10(9):1110–1115. doi: 10.1038/nn1969
- Videbech P, Ravnkilde B. Hippocampal volume and depression: a meta-analysis of MRI studies. Am J Psychiatry. 2004 Nov;161(11):1957–1966. doi: 10.1176/appi.ajp.161.11.1957
- MacQueen GM, Campbell S, McEwen BS, et al. Course of illness, hippocampal function, and hippocampal volume in major depression. Proc Natl Acad Sci USA. 2003 Feb 4;100(3):1387–1392. doi: 10.1073/pnas.0337481100
- Warner-Schmidt JL, Duman RS. Hippocampal neurogenesis: opposing effects of stress and antidepressant treatment. Hippocampus. 2006;16(3):239–249. doi: 10.1002/hipo.20156
- Boldrini M, Santiago AN, Hen R, et al. Hippocampal granule neuron number and dentate gyrus volume in antidepressant-treated and untreated major depression. Neuropsychopharmacology. 2013 May;38(6):1068–1077.
- Kang E, Wen Z, Song H, et al. Adult neurogenesis and psychiatric disorders. Cold Spring Harb Perspect Biol. 2016 Sep 1;8(9):a019026. doi: 10.1101/cshperspect.a019026
- Snyder JS, Choe JS, Clifford MA, et al. Adult-born hippocampal neurons are more numerous, faster maturing, and more involved in behavior in rats than in mice. J Neurosci. 2009 Nov 18;29(46):14484–14495. doi: 10.1523/JNEUROSCI.1768-09.2009
- Encinas JM, Sierra A. Neural stem cell deforestation as the main force driving the age-related decline in adult hippocampal neurogenesis. Behav Brain Res. 2012 Feb 14;227(2):433–439. doi: 10.1016/j.bbr.2011.10.010
- Schmidt-Hieber C, Jonas P, Bischofberger J. Enhanced synaptic plasticity in newly generated granule cells of the adult hippocampus. Nature. 2004 May 13;429(6988):184–187. doi: 10.1038/nature02553
- Bruel-Jungerman E, Laroche S, Rampon C. New neurons in the dentate gyrus are involved in the expression of enhanced long-term memory following environmental enrichment. Eur J Neurosci. 2005 Jan;21(2):513–521. doi: 10.1111/j.1460-9568.2005.03875.x
- Hill AS, Sahay A, Hen R. Increasing adult hippocampal neurogenesis is sufficient to reduce anxiety and depression-like behaviors. Neuropsychopharmacology. 2015 Sep;40(10):2368–2378. doi: 10.1038/npp.2015.85
- Snyder JS, Soumier A, Brewer M, et al. Adult hippocampal neurogenesis buffers stress responses and depressive behaviour. Nature. 2011 Aug 3;476(7361):458–461. doi: 10.1038/nature10287
- Saral S, Topcu A, Alkanat M, et al. Agomelatine attenuates cisplatin-induced cognitive impairment via modulation of BDNF/TrkB signaling in rat hippocampus. J Chem Neuroanat. 2023 Jul;130:102269. doi: 10.1016/j.jchemneu.2023.102269
- Hong N, Kim HJ, Kang K, et al. Photobiomodulation improves the synapses and cognitive function and ameliorates epileptic seizure by inhibiting downregulation of Nlgn3. Cell Biosci. 2023 Jan 12;13(1):8. doi: 10.1186/s13578-022-00949-6
- Fanselow MS, Dong HW. Are the dorsal and ventral hippocampus functionally distinct structures? Neuron. 2010 Jan 14;65(1):7–19. doi: 10.1016/j.neuron.2009.11.031
- Kheirbek MA, Drew LJ, Burghardt NS, et al. Differential control of learning and anxiety along the dorsoventral axis of the dentate gyrus. Neuron. 2013 Mar 6;77(5):955–968. doi: 10.1016/j.neuron.2012.12.038
- Tanti A, Belzung C. Neurogenesis along the septo-temporal axis of the hippocampus: are depression and the action of antidepressants region-specific? Neuroscience. 2013 Nov 12;252:234–252. doi: 10.1016/j.neuroscience.2013.08.017
- Drew LJ, Fusi S, Hen R. Adult neurogenesis in the mammalian hippocampus: why the dentate gyrus? Learn Mem. 2013 Nov 19;20(12):710–729. doi: 10.1101/lm.026542.112
- Baptista P, Andrade JP. Adult hippocampal neurogenesis: regulation and possible functional and clinical correlates. Front Neuroanat. 2018;12:44. doi: 10.3389/fnana.2018.00044
- Moreno-Jimenez EP, Flor-Garcia M, Terreros-Roncal J, et al. Adult hippocampal neurogenesis is abundant in neurologically healthy subjects and drops sharply in patients with Alzheimer’s disease. Nat Med. 2019 Apr;25(4):554–560.
- Boldrini M, Fulmore CA, Tartt AN, et al. Human hippocampal neurogenesis persists throughout aging. Cell Stem Cell. 2018 Apr 5;22(4):589–599 e5. doi: 10.1016/j.stem.2018.03.015
- Terreros-Roncal J, Moreno-Jimenez EP, Flor-Garcia M, et al. Response to comment on “impact of neurodegenerative diseases on human adult hippocampal neurogenesis”. Science. 2022 Apr 15;376(6590):eabo0920. doi: 10.1126/science.abo0920
- Boldrini M, Hen R, Underwood MD, et al. Hippocampal angiogenesis and progenitor cell proliferation are increased with antidepressant use in major depression. Biol Psychiatry. 2012 Oct 1;72(7):562–571. doi: 10.1016/j.biopsych.2012.04.024
- Anacker C, Zunszain PA, Cattaneo A, et al. Antidepressants increase human hippocampal neurogenesis by activating the glucocorticoid receptor. Mol Psychiatry. 2011 Jul;16(7):738–750.
- Santarelli L, Saxe M, Gross C, et al. Requirement of hippocampal neurogenesis for the behavioral effects of antidepressants. Science. 2003 Aug 8;301(5634):805–809. doi: 10.1126/science.1083328
- Eliwa H, Belzung C, Surget A. Adult hippocampal neurogenesis: is it the alpha and omega of antidepressant action? Biochem Pharmacol. 2017 Oct 1;141:86–99. doi: 10.1016/j.bcp.2017.08.005
- Wong CH, Siah KW, Lo AW. Estimation of clinical trial success rates and related parameters. Biostatistics. 2019 Apr 1;20(2):273–286. doi: 10.1093/biostatistics/kxx069
- Mahar I, Bambico FR, Mechawar N, et al. Stress, serotonin, and hippocampal neurogenesis in relation to depression and antidepressant effects. Neurosci Biobehav Rev. 2014 Jan;38:173–192. doi: 10.1016/j.neubiorev.2013.11.009
- Haroon E, Miller AH, Sanacora G. Inflammation, glutamate, and glia: a trio of trouble in mood disorders. Neuropsychopharmacology. 2017 Jan;42(1):193–215. doi: 10.1038/npp.2016.199
- Thorsell A, Mathe AA. Neuropeptide Y in alcohol addiction and affective disorders. Front Endocrinol. 2017;8:178. doi: 10.3389/fendo.2017.00178
- Kask A, Harro J, von Horsten S, et al. The neurocircuitry and receptor subtypes mediating anxiolytic-like effects of neuropeptide Y. Neurosci Biobehav Rev. 2002 May;26(3):259–283.
- Kormos V, Gaszner B. Role of neuropeptides in anxiety, stress, and depression: from animals to humans. Neuropeptides. 2013 Dec;47(6):401–419. doi: 10.1016/j.npep.2013.10.014
- Zaben MJ, Gray WP. Neuropeptides and hippocampal neurogenesis. Neuropeptides. 2013 Dec;47(6):431–438. doi: 10.1016/j.npep.2013.10.002
- Reichmann F, Holzer PNY. Neuropeptide Y: A stressful review. Neuropeptides. 2016 Feb;55:99–109. doi: 10.1016/j.npep.2015.09.008
- Jimenez-Vasquez PA, Diaz-Cabiale Z, Caberlotto L, et al. Electroconvulsive stimuli selectively affect behavior and neuropeptide Y (NPY) and NPY Y(1) receptor gene expressions in hippocampus and hypothalamus of flinders sensitive line rat model of depression. Eur Neuropsychopharmacol. 2007 Mar;17(4):298–308.
- Redrobe JP, Dumont Y, Fournier A, et al. The neuropeptide Y (NPY) Y1 receptor subtype mediates NPY-induced antidepressant-like activity in the mouse forced swimming test. Neuropsychopharmacology. 2002 May;26(5):615–624.
- Goyal SN, Upadhya MA, Kokare DM, et al. Neuropeptide Y modulates the antidepressant activity of imipramine in olfactory bulbectomized rats: involvement of NPY Y1 receptors. Brain Res. 2009 Apr 17;1266:45–53. doi: 10.1016/j.brainres.2009.02.033
- Corvino V, Marchese E, Podda MV, et al. The neurogenic effects of exogenous neuropeptide Y: early molecular events and long-lasting effects in the hippocampus of trimethyltin-treated rats. PLOS ONE. 2014;9(2):e88294. doi: 10.1371/journal.pone.0088294
- Cohen H, Liu T, Kozlovsky N, et al. The neuropeptide Y (NPY)-ergic system is associated with behavioral resilience to stress exposure in an animal model of post-traumatic stress disorder. Neuropsychopharmacology. 2012 Jan;37(2):350–363.
- Cohen H, Vainer E, Zeev K, et al. Neuropeptide S in the basolateral amygdala mediates an adaptive behavioral stress response in a rat model of posttraumatic stress disorder by increasing the expression of BDNF and the neuropeptide YY1 receptor. Eur Neuropsychopharmacol. 2018 Jan;28(1):159–170.
- Katsetos CD, Del Valle L, Geddes JF, et al. Aberrant localization of the neuronal class III beta-tubulin in astrocytomas. Arch Pathol Lab Med. 2001 May;125(5):613–624.
- O’Donnell D, Ahmad S, Wahlestedt C, et al. Expression of the novel galanin receptor subtype GALR2 in the adult rat CNS: distinct distribution from GALR1. J Comp Neurol. 1999 Jul 5;409(3):469–481. doi: 10.1002/(SICI)1096-9861(19990705)409:3<469:AID-CNE10>3.0.CO;2-Q
- Lu X, Mazarati A, Sanna P, et al. Distribution and differential regulation of galanin receptor subtypes in rat brain: effects of seizure activity. Neuropeptides. 2005 Jun;39(3):147–152.
- Barr AM, Kinney JW, Hill MN, et al. A novel, systemically active, selective galanin receptor type-3 ligand exhibits antidepressant-like activity in preclinical tests. Neurosci Lett. 2006 Sep 11;405(1–2):111–115. doi: 10.1016/j.neulet.2006.06.033
- Kuteeva E, Wardi T, Hokfelt T, et al. Galanin enhances and a galanin antagonist attenuates depression-like behaviour in the rat. Eur Neuropsychopharmacol. 2007 Jan;17(1):64–69.
- Kuteeva E, Wardi T, Lundstrom L, et al. Differential role of galanin receptors in the regulation of depression-like behavior and monoamine/stress-related genes at the cell body level. Neuropsychopharmacology. 2008 Oct;33(11):2573–2585.
- Luo H, Liu Z, Liu B, et al. Virus-mediated overexpression of ETS-1 in the ventral hippocampus counteracts depression-like behaviors in rats. Neurosci Bull. 2019 Dec;35(6):1035–1044.
- Lu X, Ross B, Sanchez-Alavez M, et al. Phenotypic analysis of GalR2 knockout mice in anxiety- and depression-related behavioral tests. Neuropeptides. 2008 Aug;42(4):387–397.
- Yun S, Reyes-Alcaraz A, Lee YN, et al. Spexin-based galanin receptor type 2 agonist for comorbid mood disorders and abnormal body weight. Front Neurosci. 2019;13:391. doi: 10.3389/fnins.2019.00391
- Sharma A, Ren X, Zhang H, et al. Effect of depression and suicidal behavior on neuropeptide Y (NPY) and its receptors in the adult human brain: a postmortem study. Prog Neuropsychopharmacol Biol Psychiatry. 2022 Jan 10;112:110428. doi: 10.1016/j.pnpbp.2021.110428
- Juhasz G, Hullam G, Eszlari N, et al. Brain galanin system genes interact with life stresses in depression-related phenotypes. Proc Natl Acad Sci USA. 2014 Apr 22;111(16):E1666–73. doi: 10.1073/pnas.1403649111
- Narvaez M, Millon C, Borroto-Escuela D, et al. Galanin receptor 2-neuropeptide Y Y1 receptor interactions in the amygdala lead to increased anxiolytic actions. Brain Struct Funct. 2015 Jul;220(4):2289–2301.
- Narvaez M, Borroto-Escuela DO, Millon C, et al. Galanin receptor 2-neuropeptide Y Y1 receptor interactions in the dentate gyrus are related with antidepressant-like effects. Brain Struct Funct. 2016 Nov;221(8):4129–4139.
- Narvaez M, Borroto-Escuela DO, Santin L, et al. A novel integrative mechanism in anxiolytic behavior induced by galanin 2/Neuropeptide Y Y1 receptor interactions on medial paracapsular intercalated amygdala in rats. Front Cell Neurosci. 2018;12:119. doi: 10.3389/fncel.2018.00119
- Mirchandani-Duque M, Barbancho MA, Lopez-Salas A, et al. Galanin and neuropeptide y interaction enhances proliferation of granule precursor cells and expression of neuroprotective factors in the rat hippocampus with consequent augmented spatial memory. Biomedicines. 2022 Jun 1;10(6):1297. doi: 10.3390/biomedicines10061297
- Borroto-Escuela DO, Pita-Rodriguez M, Fores-Pons R, et al. Galanin and neuropeptide Y interactions elicit antidepressant activity linked to neuronal precursor cells of the dentate gyrus in the ventral hippocampus. J Cell Physiol. 2021 May;236(5):3565–3578.
- Borroto-Escuela DO, Fores R, Pita M, et al. Intranasal delivery of galanin 2 and neuropeptide Y1 agonists enhanced spatial memory performance and neuronal precursor cells proliferation in the dorsal hippocampus in rats. Front Pharmacol. 2022;13:820210. doi: 10.3389/fphar.2022.820210
- Diaz-Sanchez E, Lopez-Salas A, Mirchandani-Duque M, et al. Decreased medial prefrontal cortex activity related to impaired novel object preference task performance following GALR2 and Y1R agonists intranasal infusion. Biomed Pharmacother. 2023 May;161:114433. doi: 10.1016/j.biopha.2023.114433
- Fuzesi T, Wittmann G, Liposits Z, et al. Contribution of noradrenergic and adrenergic cell groups of the brainstem and agouti-related protein-synthesizing neurons of the arcuate nucleus to neuropeptide-y innervation of corticotropin-releasing hormone neurons in hypothalamic paraventricular nucleus of the rat. Endocrinology. 2007 Nov;148(11):5442–5450.
- Gelfo F, Tirassa P, De Bartolo P, et al. NPY intraperitoneal injections produce antidepressant-like effects and downregulate BDNF in the rat hypothalamus. CNS Neurosci Ther. 2012 Jun;18(6):487–492.
- Decressac M, Wright B, David B, et al. Exogenous neuropeptide Y promotes in vivo hippocampal neurogenesis. Hippocampus. 2011 Mar;21(3):233–238.
- Nahvi RJ, Tanelian A, Nwokafor C, et al. Intranasal neuropeptide Y as a potential therapeutic for depressive behavior in the rodent single prolonged stress model in females. Front Behav Neurosci. 2021;15:705579. doi: 10.3389/fnbeh.2021.705579
- Silveira Villarroel H, Bompolaki M, Mackay JP, et al. NPY induces stress resilience via downregulation of I(h) in principal neurons of rat basolateral amygdala. J Neurosci. 2018 May 9;38(19):4505–4520. doi: 10.1523/JNEUROSCI.3528-17.2018
- Morales-Medina JC, Juarez I, Venancio-Garcia E, et al. Impaired structural hippocampal plasticity is associated with emotional and memory deficits in the olfactory bulbectomized rat. Neuroscience. 2013 Apr 16;236:233–243. doi: 10.1016/j.neuroscience.2013.01.037
- Mechawar N, Saghatelyan A, Grailhe R, et al. Nicotinic receptors regulate the survival of newborn neurons in the adult olfactory bulb. Proc Natl Acad Sci USA. 2004 Jun 29;101(26):9822–9826. doi: 10.1073/pnas.0403361101
- Cohen H, Zohar J, Kaplan Z, et al. Adjunctive treatment with brexpiprazole and escitalopram reduces behavioral stress responses and increase hypothalamic NPY immunoreactivity in a rat model of PTSD-like symptoms. Eur Neuropsychopharmacol. 2018 Jan;28(1):63–74.
- Rapp A, Brandl N, Volpi N, et al. Evaluation of chondroitin sulfate bioactivity in hippocampal neurones and the astrocyte cell line U373: influence of position of sulfate groups and charge density. Basic Clin Pharmacol Toxicol. 2005 Jan;96(1):37–43.
- Narvaez M, Andrade-Talavera Y, Valladolid-Acebes I, et al. Existence of FGFR1-5-HT1AR heteroreceptor complexes in hippocampal astrocytes. Putative link to 5-HT and FGF2 modulation of hippocampal gamma oscillations. Neuropharmacology. 2020 Jun 15;170:108070. doi: 10.1016/j.neuropharm.2020.108070
- Alvarez-Contino JE, Diaz-Sanchez E, Mirchandani-Duque M, et al. GALR2 and Y1R agonists intranasal infusion enhanced adult ventral hippocampal neurogenesis and antidepressant-like effects involving BDNF actions. J Cell Physiol. 2023 Feb;238(2):459–474.
- Porsolt RD, Le Pichon M, Jalfre M. Depression: a new animal model sensitive to antidepressant treatments. Nature. 1977 Apr 21;266(5604):730–732. doi: 10.1038/266730a0
- Planchez B, Surget A, Belzung C. Animal models of major depression: drawbacks and challenges. J Neural Transm (Vienna). 2019 Nov;126(11):1383–1408. doi: 10.1007/s00702-019-02084-y
- Yankelevitch-Yahav R, Franko M, Huly A, et al. The forced swim test as a model of depressive-like behavior. J Vis Exp. 2015 Mar 2;(97). doi: 10.3791/52587.
- Kalynchuk LE, Gregus A, Boudreau D, et al. Corticosterone increases depression-like behavior, with some effects on predator odor-induced defensive behavior, in male and female rats. Behav Neurosci. 2004 Dec;118(6):1365–1377.
- Morales-Medina JC, Dumont Y, Benoit CE, et al. Role of neuropeptide Y Y(1) and Y(2) receptors on behavioral despair in a rat model of depression with co-morbid anxiety. Neuropharmacology. 2012 Jan;62(1):200–208.
- Ribeiro ACR, Zhu J, Kronfol MM, et al. Molecular mechanisms for the antidepressant-like effects of a low-dose ketamine treatment in a DFP-based rat model for gulf war illness. Neurotoxicology. 2020 Sep;80:52–59. doi: 10.1016/j.neuro.2020.06.011
- Cryan JF, Page ME, Lucki I. Differential behavioral effects of the antidepressants reboxetine, fluoxetine, and moclobemide in a modified forced swim test following chronic treatment. Psychopharmacol (Berl). 2005 Nov;182(3):335–344. doi: 10.1007/s00213-005-0093-5
- Paxinos G, Watson C. The rat brain in stereotaxic coordinates: hard cover edition. Elsevier; 2006.
- Kim KK, Adelstein RS, Kawamoto S. Identification of neuronal nuclei (NeuN) as fox-3, a new member of the fox-1 gene family of splicing factors. J Biol Chem. 2009 Nov 6;284(45):31052–31061. doi: 10.1074/jbc.M109.052969
- Yagi S, Splinter JEJ, Tai D, et al. Sex differences in maturation and attrition of adult neurogenesis in the hippocampus. eNeuro. 2020 Jul;7(4):ENEURO.0468–19.2020.
- Planchez B, Lagunas N, Le Guisquet AM, et al. Increasing adult hippocampal neurogenesis promotes resilience in a mouse model of depression. Cells. 2021 Apr 21;10(5):972. doi: 10.3390/cells10050972
- Walker AK, Rivera PD, Wang Q, et al. The P7C3 class of neuroprotective compounds exerts antidepressant efficacy in mice by increasing hippocampal neurogenesis. Mol Psychiatry. 2015 Apr;20(4):500–508.
- Bauman MD, Schumann CM, Carlson EL, et al. Neuroprotective efficacy of P7C3 compounds in primate hippocampus. Transl Psychiatry. 2018 Sep 26;8(1):202. doi: 10.1038/s41398-018-0244-1
- Colucci-D’Amato L, Speranza L, Volpicelli F. Neurotrophic factor BDNF, physiological functions and therapeutic potential in depression, neurodegeneration and brain cancer. Int J Mol Sci. 2020 Oct 21;21(20):7777. doi: 10.3390/ijms21207777
- Miranda M, Morici JF, Zanoni MB, et al. Brain-derived neurotrophic factor: a key molecule for memory in the healthy and the pathological brain. Front Cell Neurosci. 2019;13:363. doi: 10.3389/fncel.2019.00363
- Castren E, Kojima M. Brain-derived neurotrophic factor in mood disorders and antidepressant treatments. Neurobiol Dis. 2017 Jan;97(Pt B):119–126. doi: 10.1016/j.nbd.2016.07.010
- Murawska-Cialowicz E, Wiatr M, Cialowicz M, et al. BDNF impact on biological markers of depression—role of physical exercise and training. Int J Environ Res Public Health. 2021 Jul 15;18(14):7553. doi: 10.3390/ijerph18147553
- Dwivedi Y. Brain-derived neurotrophic factor in suicide pathophysiology. In: Dwivedi Y, editor. The neurobiological basis of suicide. Boca Raton (FL): CRC Press/Taylor & Francis; 2012. Chapter 8.
- Pandey GN, Ren X, Rizavi HS, et al. Brain-derived neurotrophic factor and tyrosine kinase B receptor signalling in post-mortem brain of teenage suicide victims. Int J Neuropsychopharmacol. 2008 Dec;11(8):1047–1061.
- Hoshaw BA, Malberg JE, Lucki I. Central administration of IGF-I and BDNF leads to long-lasting antidepressant-like effects. Brain Res. 2005 Mar 10;1037(1–2):204–208. doi: 10.1016/j.brainres.2005.01.007
- Corvino V, Marchese E, Giannetti S, et al. The neuroprotective and neurogenic effects of neuropeptide Y administration in an animal model of hippocampal neurodegeneration and temporal lobe epilepsy induced by trimethyltin. J Neurochem. 2012 Jul;122(2):415–426.
- Croce N, Dinallo V, Ricci V, et al. Neuroprotective effect of neuropeptide Y against beta-amyloid 25-35 toxicity in SH-SY5Y neuroblastoma cells is associated with increased neurotrophin production. Neurodegener Dis. 2011;8(5):300–309. doi: 10.1159/000323468
- Park SW, Nhu le H, Cho HY, et al. p11 mediates the BDNF-protective effects in dendritic outgrowth and spine formation in B27-deprived primary hippocampal cells. J Affect Disord. 2016 May 15;196:1–10. doi: 10.1016/j.jad.2016.02.010
- Kim HI, Lim J, Choi HJ, et al. ERRγ ligand regulates adult neurogenesis and depression-like behavior in a LRRK2-G2019S-associated young female mouse model of parkinson’s disease. Neurotherapeutics. 2022 May 25;19(4):1298–1312. doi: 10.1007/s13311-022-01244-5
- Sabban EL, Serova LI. Potential of intranasal neuropeptide Y (NPY) and/or melanocortin 4 receptor (MC4R) antagonists for preventing or treating PTSD. Mil Med. 2018 Mar 1;183(suppl_1):408–412. doi: 10.1093/milmed/usx228
- Serova L, Mulhall H, Sabban E. NPY1 receptor agonist modulates development of depressive-like behavior and gene expression in hypothalamus in SPS rodent PTSD model. Front Neurosci. 2017;11:203. doi: 10.3389/fnins.2017.00203
- Mathe AA, Michaneck M, Berg E, et al. A randomized controlled trial of intranasal neuropeptide y in patients with major depressive disorder. Int J Neuropsychopharmacol. 2020 Dec 29;23(12):783–790. doi: 10.1093/ijnp/pyaa054
- Detke MJ, Rickels M, Lucki I. Active behaviors in the rat forced swimming test differentially produced by serotonergic and noradrenergic antidepressants. Psychopharmacol (Berl). 1995 Sep;121(1):66–72. doi: 10.1007/BF02245592
- Polis AJ, Fitzgerald PJ, Hale PJ, et al. Rodent ketamine depression-related research: finding patterns in a literature of variability. Behav Brain Res. 2019 Dec 30;376:112153. doi: 10.1016/j.bbr.2019.112153
- Kuipers A, Balasko M, Petervari E, et al. Intranasal delivery of a methyllanthionine-stabilized galanin receptor-2-selective agonist reduces acute food intake. Neurotherapeutics. 2021 Oct;18(4):2737–2752.
- Heilig M, Soderpalm B, Engel JA, et al. Centrally administered neuropeptide Y (NPY) produces anxiolytic-like effects in animal anxiety models. Psychopharmacol (Berl). 1989;98(4):524–529. doi: 10.1007/BF00441953
- Redrobe JP, Dumont Y, Fournier A, et al. Role of serotonin (5-HT) in the antidepressant-like properties of neuropeptide Y (NPY) in the mouse forced swim test. Peptides. 2005 Aug;26(8):1394–1400.