ABSTRACT
The genomic arrangement of most picornavirus of the Picornaviridae family shares a similar monocistronic genomic pattern and a defining organizational feature. A defining feature of picornavirus is the presence of evolutionarily conserved and highly-structured RNA elements in untranslated regions (UTRs) at the genome’ 5’and 3’ ends, essential for viral replication and translation. Given the diversity and complexity of RNA structure and the limitations of molecular biology techniques, the functional characterization and biological significance of UTRs remain to be fully elucidated, especially for 5’ UTR. Here, we summarize the current knowledge of the 5’ UTR of picornavirus. This review focuses on the structural characterization and the biological function of the RNA secondary and tertiary structures in the 5’ UTR of picornavirus. Understanding the role of the 5’ UTR of picornavirus can provide a deep insight into the viral replication cycle and pathogenic mechanisms.
1. Introduction
The members of the Picornaviridae family consist of many highly contagious pathogens. Based on the 10th International Committee on Taxonomy of Viruses (ICTV), the latest taxonomy and classification of picornaviruses contains 158 species grouped into 68 genera (https://www.picornaviridae.com/). The Aphthovirus, Cardiovirus, Enterovirus, Hepatovirus, Parechovirus, and Teschovirus of picornaviruses have been intensely studied until now (). As shown in , some members of picornavirus have been known to exist for centuries, including poliovirus (PV), encephalomyocarditis virus (EMCV), enterovirus 71 (EV71), and the foot-and-mouth disease virus (FMDV). Additionally, several emerging aquatic animal viruses, such as limnipivirus and potamipivirus, were recently divided into this family [Citation50] (). These picornaviruses infect a wide range of hosts, from humans to wildlife and domestic animals, and cause a series of diseases ranging from mild respiratory illness to severe symptoms, such as poliomyelitis, encephalitis, myocarditis, meningitis, and hepatitis [Citation50–52].
Table 1. Natural host ranges and associated diseases of picornaviruses.
Except for cadicivirus A, genus Dicipivirus, which has a bicistronic genome, the genomic arrangement of the majority of picornavirus maintains the monocistronic genomic pattern [Citation17]. The majority of picornavirus shared a similar genomic organizational pattern and a defining feature (). The arrangement of the picornavirus genome is evolutionarily conserved, and the viral genome as mRNA enables direct protein synthesis. The linear genomic lengths vary from 6.7 kb for seal picornavirus to 10.1 kb for goose megrivirus [Citation53]. As shown in , the viral genome encodes a large open reading frame (ORF) with a 5' untranslated region and a 3 untranslated region at either end of the ORF, and the viral genome is covalently linked to a terminal protein termed 3B or VPg at the 5' end (). After the translation of the single ORF, newly synthesized polyproteins undergo cascade cleavage by viral-encoded proteases to produce capsid and nonstructural proteins [Citation54,Citation55]. Meanwhile, all picornavirus genomes encode at least one proteinase (e.g. 3Cpro/3CDpro), and some encode the Leader proteinase (e.g. Lpro). Notably, the initiation of picornavirus replication is featured by the uridylylation of VPg [Citation56]. Given the absence of a proofreading mechanism in the RNA-dependent RNA polymerase (RdRp), the viral genome of picornavirus has a highly variable mutation rate, which has led to rapid proliferation and evolution. Viral RdRp catalyzes uridine monophosphate (UMP) transfer to the hydroxyl group of the tyrosine residue of VPg to form VPg-pUpU, which subsequently acts as a primer to initiate genome replication [Citation56–58]. Noteworthy, both FMDV and seal picornavirus consist of more than one VPg encoded in the genome [Citation9,Citation59]. In contrast, other picornaviruses member encodes a copy of VPg ranging from 22 to 24 amino acid residues [Citation9,Citation60].
Figure 1. The viral genome structure of poliovirus and the 5’ UTR of poliovirus and FMDV. (a) The diagram of the viral genome structure of poliovirus. The viral genome is covalently linked to a terminal protein termed VPg. After the translation of the single ORF, newly synthesized polyproteins undergo cascade cleavage by viral-encoded proteases to produce capsid and non-structural proteins, including the VP4, VP2, VP3, VP1, 2Apro, 2B, 2C, 3A, 3B, 3C, and 3Dpol. (b) The diagram of the 5’ UTR structure of poliovirus. The 5’ end of the PV genome is covalently linked to the VPg, and the terminal structure at the 5’ end is a cloverleaf structure followed by an IRES element consisting of five domains, including I, II, III, IV, V, and VI. (c) The diagram of the 5’ UTR structure of FMDV. The 5’ end of the FMDV genome is covalently linked to the VPg, and the terminal structure at the 5’ end folds into a large stem-loop called the S fragment. The S fragment is followed by the poly (C) tract, the tandem PK, the Cre, and the IRES consisting of four domains (II, III, IV, and V). Meanwhile, FMDV contains two AUGs and generally utilizes the second AUG.
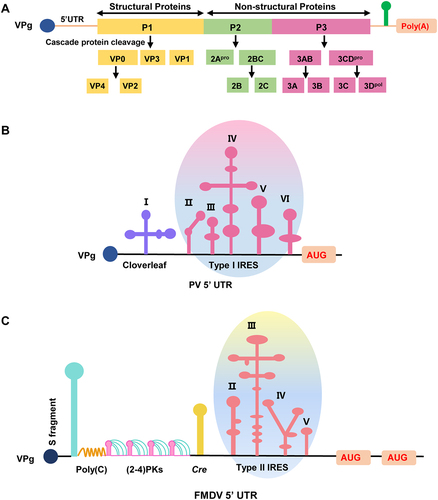
Each viral genome of picornaviruses is a positive-sense RNA with a genus-specific RNA element, including 5' UTR and 3' UTR. Proper folding of RNA elements helps untranslated regions to function correctly to facilitate the viral life cycle. High-order structures of the 3' UTR are generally related to regulating viral negative-strand synthesis [Citation61]. For instance, disruption of intramolecular interaction of RNA elements in the 3' UTR does not affect the translation of the coxsackie B virus (CBV) but adversely affects viral genome replication [Citation61]. Compared with 3' UTR elements, the biological function of the other RNA cis-acting elements enriched in the 5' UTR and their role in viral replication or translation have yet to be fully elucidated. Here, we review the research progress on the structural diversity and biological role of the 5' UTR and briefly summarize the biological significance of the viral 5' UTR of picornavirus on viral replication and pathogenicity.
2. The structure characterization of representative 5’ UTR elements of picornavirus
Just as proteins form structural motifs (e.g. beta-hairpin and EF-hand), the 5' UTR of picornaviruses contains many spatially-ordered and variable-length structural elements. Noteworthy, the RNA structures in the 5' UTR consist of stem-loop, homo-polymeric tract, tandem pseudoknot (PK), and some high-order functional domains composed of these structures [Citation62–64]. Multiple complex functional elements perform essential viral genome replication and translation functions, such as the cloverleaf and internal ribosome entry site (IRES) (). The viral negative-strand RNA synthesis initiation requires a poly (A) tail and a cloverleaf structure at the 5' end [Citation65]. It is worth noting that the largest and most common RNA structural unit in the 5' UTR is the IRES, which confers cap-independent translation properties [Citation66]. Different from the ubiquity of IRES, the poly (C) tract is considered unique to Cardioviruses and Aphthoviruses because it is absent from other genera of the picornavirus family [Citation67,Citation68]. Additionally, PKs are a reasonably complicated RNA structure element found mainly in the 5' UTR of FMDV and other specialized types of IRES [Citation69,Citation70].
2.1. The structural diversity and biological role of 5’ UTR stem-loop element
The stem-loop is a double-stranded structure consisting of tens to hundreds of bases. It follows the complementary base-pairing principle of intramolecular sequences, a low free energy structure form [Citation71]. The majority of terminal structural elements at the 5' UTR of picornaviruses (e.g. Aphthovirus, Cardiovirus, Enterovirus, and Kobuvirus) are folded into a branched or unbranched stem-loop of varying sizes, including the S fragment, a cloverleaf-shaped structure, and so on [Citation72]. FMDV has a sizable single stem-loop, an S fragment, which is longer than other picornaviruses (). Noteworthy, the length of the S fragment varies from different FMDV isolates. Partial nucleotide deletion and truncations of the S fragment were able to alter the host tropism and affect innate immune response in host cells, showing that structural integrity is essential for functional performance [Citation73,Citation74]. Meanwhile, the Aichi virus, a member of the Kobuvirus genus, contains three independent stem-loops (e.g. SL-A, SL-B, and SL-C) at the 5' end of the genome, which is required for viral RNA synthesis [Citation75]. Mutation of the sequence at the bottom of SL-A will damage the synthesis of the positive strand without affecting the synthesis of the negative strand. In addition, SL-A is involved in RNA encapsidation [Citation75]. Significantly, the terminal element of the Enterovirus genus takes the form of a cloverleaf-shaped structure with three stem-loops (e.g. stem-loop b, stem-loop c, and stem-loop d) emanating from a single stem, which plays a crucial part in the stability of the viral genome and the synthesis of viral negative-strand RNA [Citation72]. Subsequently, the RNP complex around the cloverleaf element interacts with the poly (A) binding protein of the 3' poly(A) tail, thereby linking the ends of the viral genome and successfully circularizing it through a protein-protein bridge [Citation65]. The cloverleaf structure was also demonstrated as a multifunctional shield for protecting uncapped genome RNA from degradation by cellular nucleases [Citation72]. Analogous to the cloverleaf structure, the S fragment is speculated to have a similar function. Still, there are few studies on the S fragment, so the role in these aspects has yet to be confirmed. In addition to the above stem-loop elements located at the very end of the genome, the cis-acting replication element (Cre) is another functional stem-loop element in the middle of FMDV 5' UTR, which contains a conserved AAACA motif that serves as a template for the addition of U residues to the peptide primer 3B [Citation76]. However, the Cre is usually located in the protein-coding region of other picornavirus genomes. For example, the Cre of Enterovirus is generally located in the 2C region, while the Cre of Cardiovirus is in the VP2 region [Citation77]. The variable position of Cre in different picornavirus suggests that its localization in the genome may not be critical.
2.2. The biological function and structure characterization of 5’ UTR poly (C) element
Nucleotide sequencing analysis of the Cardiovirus and Aphthovirus genera has identified a distinctive poly (C) tract at the 5' UTR. The length of cytosine residues varies considerably depending on the species [Citation26,Citation78]. On the one hand, whether the length of poly (C) affects the virulence and pathogenicity of picornavirus is still controversial. For instance, the attenuated virulence of the SAT1–82 vaccine strain of FMDV is associated with a reduction in poly (C) length [Citation79], which is in contrast to the conclusion of the highly attenuated R100 strain of FMDV still has 420 cytosine residues [Citation80]. On the other hand, the molecular basis of poly (C) function in virus replication, pathogenicity, and host tropism is also an unwinding enigma. FMDV has been identified to maintain a long poly (C) tract, up to 250 nucleotides in some field isolates, while EMCV poly (C) is shorter [Citation79,Citation81,Citation82]. Transfection of FMDV RNA transcripts with different lengths of poly (C) tract into BHK21 cells showed similar infectivity. Besides, the recombinant FMDV with a poly (C) tract of two cytidines was viable in BHK21 cells, even at low growth levels, which suggests that a long poly (C) tract is optional for FMDV replication [Citation83]. Compared with wild-type viruses, the Cardiovirus also has identified that substantial or total poly (C) deletion can still generate viable viruses and have similar replicate kinetics and titers in vitro [Citation84–86]. Mengovirus, a member of the Cardiovirus genus, contains a poly (C) tract with the sequence of C50UC10. Interestingly, the truncation or deletion of poly(C) of the Mengovirus does not impair the growth of the virus in cell culture but resulted in a 106 109 fold decrease in pathogenicity in mice [Citation67]. Furthermore, the results of the animal challenge experiment suggested that these recombinant Mengovirus strains produced higher levels of neutralizing antibodies and acquired more long-term protective immunity than wild-type, providing the possibility for genetic engineering vaccine application [Citation67]. However, more comprehensive data from other animal experiments suggested that both short- and long poly(C)-tract variants of Mengovirus were highly virulent to newborn mice [Citation87]. Therefore, whether the poly(C) length affects virulence for picornaviruses remains to be explored.
2.3. The structure and biological function of 5’ UTR PK element
The PKs element in 5' UTR was initially discovered in the turnip yellow mosaic virus (TYMV) [Citation88] and is now considered to be a common motif with versatile functions in biological activities. A typical PK structure formed by complementary base-pairing of single-stranded RNA regions within hairpin loops with nucleotides outside the loop is known as H-type PKs [Citation89]. Besides, PK can also be formed when single-stranded bulge, interior and multi-branched loops base-pairing with complementary regions [Citation90]. Based on the chemical probing combined with high-throughput sequencing techniques, PKs in different viruses were identified to enable multiple roles in viral genomic replication. The PK of Human immunodeficiency virus (HIV) and Influenza viruses can regulate RNA splicing [Citation91–93], while the PK of coronaviruses is associated with ribosomal frameshifting [Citation94]. Unexpectedly, the PK of porcine reproductive and respiratory syndrome virus (PRRSV) furthermore acts as a pathogen-associated molecular pattern (PAMP) to regulate antiviral signal transduction [Citation95]. Many picornaviruses encode two to five tandem PKs within their genomes, such as EMCV [Citation12], Equine rhinitis A virus (ERAV) [Citation96], FMDV [Citation69,Citation97], and Seneca virus A (SVA) [Citation98]. The structural features of PKs determine their biological properties. The PK of both FMDV and Cricket paralysis virus (CrPV) can be involved in the composition of complex domains [Citation97,Citation99]. Two IRES in the CrPV genome, the IRES5’UTR and the IRESIGR, work synergically to shut off host translation and promote viral protein translation. The IRES5’UTR contains a three-way junction structure with a pseudoknot necessary for ribosome recruitment on the viral AUG initiation codon without scanning step [Citation99]. Generally, almost all FMDV wild-type isolates contain two or more PKs [Citation100,Citation101]. Based on the sub-genomic replicons competition experiment, the FMDV replicons with less than two PKs were outcompeted by wild-type replicons with four PKs, indicating the tendency of at least two PKs in the viral genome, which is consistent with the replicative advantage provided by the additional PKs for FMDV [Citation97]. Furthermore, the pathogenicity of FMDV with 43-nt or 86-nt deletions of PK was less than that of the wild-type in cattle, suggesting that PK is also a vital host tropism factor of FMDV [Citation69]. In addition, two adjacent stem-loops (e.g. SL-B and SL-C) in the 5' UTR of the Aichi virus can also form a PK structure by base-pairing interaction, which is essential for virus replication, and the stability of the PK structure is also required for the synthesis of negative-strand RNA of Aichi virus [Citation75]. However, even if the viral genome contains multiple PKs, the importance of the role played by each PK varies. The Mengovirus contains two PKs (e.g. PKB and PKC). When PKC is mutated, the virus is viable but has a small plaque phenotype. PKB or the combined deletion of PKB and PKC is lethal to the virus [Citation102]. Furthermore, the alteration of the PK (PKB + PKc) sequence did not affect the amount of polyprotein translation in vitro, but viral RNA synthesis was substantially impaired [Citation102].
3. The biological function and structure characterization of IRES
mRNA translation is a complex multistep process in eukaryotes, including initiation, elongation, and termination [Citation66] (). The typical structure of eukaryotic mRNA is the m7G cap at the 5' end and the poly (A) tail at the 3' end, which play an essential role in the translation and stability of the mRNA. With the assistance of the translation initiation factors, the ternary complex (eIF2–GTP – Met-tRNAi) binds to the 40S ribosomal subunit to form the 43S preinitiation complex (PIC) [Citation103]. The mRNA activation begins the eIF4E (cap-binding protein) binding to the m7G cap. Then, the assembled 43S PIC binds to the activated mRNA. Subsequently, the 40S ribosomal subunit will scan the 5' UTR along the direction from 5' to 3' of the mRNA until it encounters the first initiation codon AUG of the ORFs. After a series of complex processes, the 60S subunit joins and assembles 80S initiation complex forms [Citation104].
Figure 2. The process diagram of the eukaryotic cap-dependent translation initiation and viral IRES-dependent translation. (a) The schematic diagram of the eukaryotic canonical Cap-dependent translation initiation. The translation initiation process is divided into the following stages: (i) the formation of the eIF2-GTP-Met-tRNAi ternary complex (not depicted); (ii) the formation of the 43S preinitiation complex through the eIfs (e.g. eIF1, eIF1A, eIF3, and eIF5) together with the ternary complex and 40S subunit; (iii) the activation of mRNA initiation via the binding of eIF4E (cap-binding protein) to the m7G cap. Then, the attachment of the 43S PIC to activated mRNA. Subsequently, the scanning process of the 5’UTR along the direction from 5’ to 3’ of the mRNA by 43S complexes; (iv) the AUG recognition and the formation of 48S initiation complex, while eIF5 mediates the hydrolysis of GTP and release of Pi. (v) the subunit joining of 60S subunits to 48S complexes; (vi) the elongation began with the formation of the 80S ribosome complex and the hydrolysis of eIF5B. (b) The schematic diagram of viral IRES-dependent translation initiation. During the virus infection, the IRES element can directly recruit the 40S subunit to the initiation codon, which is an intricate mRNA secondary structure usually located within the 5’ UTR of the genome. This binding does not require complete assistance from eIfs, happening with the help of just a few eIfs or some IRES trans-acting factors (ITAFs), RNA-binding proteins. After recognizing the initiation codon, ribosomal subunits are assembled and ready for elongation, thus leading to protein synthesis.
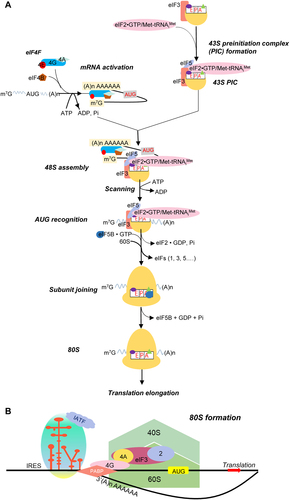
The translation initiation of picornavirus differs from eukaryotes’ mRNA translation process [Citation105,Citation106] (). For picornavirus, IRES-mediated translation confers cap-independent translation properties. Subsequently, virus-encoded protein reduces the efficiency of ribosomal subunits recruitment by removing translation initiation factors or inactivating eIFs (eukaryotic initiation factors), decreasing cellular protein synthesis. At the same time, viral mRNA efficiently expresses by its IRES. There are at least four types of IRES in the 5' UTR of the picornavirus genome. The different types of IRES differ significantly in their structural organization and the molecular mechanisms that direct translation initiation.
3.1. The type I IRES of Enterovirus
The typical Type I IRES was first identified in the poliovirus (PV) and has subsequently driven research into other types of IRES [Citation107]. Being one of the most representative members of the Enteroviruses, the PV IRES is the best-characterized model. The cloverleaf structure, also known as domain I, is followed by domains II to VI. These domains constitute a complete IRES, in which the function of IRES mainly depends on domains II to VI, and the entire structure is approximately 450 nt in length (). Some evolutionarily conserved motifs within IRES tend to retain sequences that impact RNA structure and RNA-protein interactions, ultimately determining the activity of IRES elements. Conserved pyrimidine tracts can be found in the basal part of domain II and the central region of domain V [Citation108]. Domain IV forms a cruciform structure with three loops (e.g. loop b, loop c, and loop d) emanating from the stem, providing binding sites for eIFs and IRES-transacting factors (ITAFs) for ribosome recruitment [Citation109]. The top region of Domain IV is a stem-loop with a C-rich sequence required for PCBP binding and is essential for virus protein synthesis [Citation110]. Domain V provides sites for eIF4G binding during 48S complex assembly. For PV and EV71, translation initiation requires translation initiation factors (e.g. eIF2, eIF3, eIF4A, eIF4G, eIF4B, and eIF1A) and PCBP2 (poly-r(C) binding protein 2). Subsequently, the translation initiation starts from the binding of eIF4G [Citation111]. Meanwhile, a conserved AUG in domain VI stimulates the attachment of 43S PIC and startup scan of the initiation codon. Based on the dicistronic reporter plasmid system, polypyrimidine tract-binding protein (PTB) overexpression promotes IRES-driven reporter protein expression, which reflects that PTB stimulates translation [Citation112].
Figure 3. The diagram of the secondary structure of typical four types of IRES elements in RNA viruses. (a) The structure diagram of type I IRES. Type I IRES consists of five domains, among which domain IV is the functional centre domain. Domain IV has a GNRA motif necessary for IRES activity, indicated by navy blue. Significant motifs are marked by navy blue that can maintain a secondary structure or be recognized by IRES trans-acting factors (ITAFs). G is guanine, N is any nucleotide, R is a purine (A or G), and A is adenine. (b) The structure diagram of type II IRES. Type II IRES consists of four domains, among which domain III plays a crucial role in IRES function. Domain IV has GNRA and RAAA motifs essential for IRES activity, indicated by navy blue. G is guanine, N is any nucleotide, R is a purine (A or G), and A is adenine. (c) The structure diagram of HCV/HCV-like IRES. AUG is the initiation codon, indicated by the yellow colour. (d) The structure diagram of IGR IRES. IGR IRES contains the L1.1, L1.2, PKI, PKII, PK III, SLIV, and SLV structures.
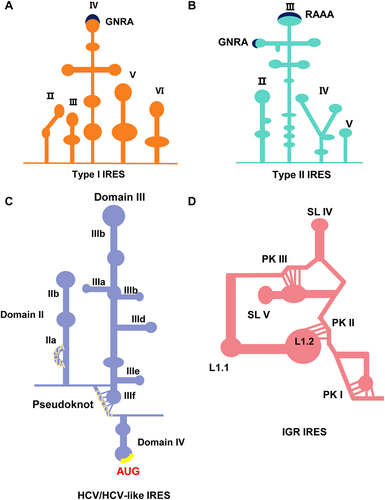
3.2. The type II IRES of Aphthovirus and Cardiovirus
Type II IRES has a highly structured organization divided into four modular domains (e.g. H to L or II – V) (). The 5' UTR of the FMDV has a Cre, a stem-loop that initiates viral genome replication, located in the upstream region of the IRES, also known as domain I. Domain II folds into a single stem-loop with a functional UCUUU motif that provides a site for PTB binding, and substituting U residues will impair IRES activity [Citation113]. Domain III occupies most of the IRES structure and plays a vital role in the function of Type II IRES. It forms a cruciform structure and has evolutionarily conserved motifs such as GNRA, RAAA, and ACCC (N stands for any nucleotide and R for purine) in different branched loops. Noteworthy, the GNRA motif is found in the IRES sequences of all picornavirus, but RAAA is only conserved in Cardiovirus and Aphthovirus. The ribonuclease and dimethyl sulphate accessibility assay revealed that the GNRA motif was necessary to maintain the structure of adjacent stem-loops, where A-G substitution would result in RNA structural recombination [Citation114]. In particular, the bifurcated stem-loops in domain IV form a Y-shaped structure. Substitutions in the internal AA loop of domain IV impaired both eIF4G-RNA binding in vitro and translation initiation in vivo for FMDV. However, substitutions close to the AA loop did not affect IRES activity. They retained their capacity to interact with eIF4G, suggesting that RNA-protein interaction of the eIF4G with a specific motif of IRES is a vital determinant of translation initiation [Citation115]. Finally, domain V comprises a hairpin structure that can bind eIF3, eIF4B, and other ITAFs to promote efficient translation [Citation116–118]. The Yn (Yn; n = 8–10 nt) – Xm (Xm; m = 18–20 nt)-AUG motif is located at the 3' end boundary of the IRES. Significantly, there are two in-frame initiation codons (AUG1 and AUG2) in FMDV. The IRES-proximal AUG cannot initiate translation efficiently, and AUG2, 84 nt away from the AUG1, guides polyprotein translation initiation. Meanwhile, eIF1 is indispensable for forming the 48S initiation complex at AUG2, and nucleotide substitution in AUG2 abrogates protein translation [Citation119,Citation120].
3.3. The hepatitis C (HCV) -like IRES of Hepatovirus and Teschovirus
As the name implies, this type of IRES is found in HCV, a member of the Flaviviridae family. HCV-like IRES was subsequently found in a few Picornaviridae viruses, such as porcine teschovirus-1 (PTV-1) and simian Picornavirus (SPV) [Citation121,Citation122]. HCV-like IRES adopt different structural organizations that show multiple strategies to promote translation initiation (). Unlike the two types of IRES mentioned above, the HCV-like IRES is composed of three domains (II, III, and IV), with domains II and III playing a more prominent role, and the overall structure of the HCV IRES is more compact than other types of IRES [Citation123,Citation124]. Domain III is mainly a stem-loop structure with a branch. Specifically, the basal region of domain III is a four-way junction structure and includes a pseudoknot [Citation125]. Sequence alignment identified that the UUGGGU motif at the apical loop in stem-loop IIId was conserved in all HCV genotypes [Citation126]. Then, all nucleotide mutations of GAUA tetra-loop in hairpin IIIe severely impair IRES activity, demonstrating that the change of motif can affect the conformation of RNA, thus affecting the function of IRES [Citation127]. Interestingly, domain IV forms a small stem-loop structure, where the start codon AUG is located [Citation123]. Notably, HCV IRES requires only eIF2 and eIF3 to form the 48S PIC, and HCV IRES promotes eIF2 function by interacting with eIF3 and 40S ribosomal subunits [Citation128].
3.4. The involvement of host proteins in the regulation of IRES activity
Except for individual types of IRES that require only a small amount of typical initiation factors (e.g. HCV-like IRES) or none (e.g. cricket paralysis virus (CrPV)- or CrPV-like IRES), most other IRES additionally require other host proteins to assist in their function [Citation129]. Now that we have mentioned CrPV, we will briefly describe its unique and rare IRES (IGR-IRES) (). IGR IRES is shorter than other picornaviruses, almost 190-nt in length [Citation130]. Crystallography and Cryo-EM techniques identified that IGR IRES consists of three PKs (PK I, PK II, and PK III), where PK II and PK III form the central ribosome binding domain to recruit the 40S and 60S ribosomal subunits [Citation131–133]. At the same time, PK I place the 80S ribosome at the initiation codon. The distinguishing feature of IGR IRES is that it does not need a translation initiation factor or Met-tRNAi to recruit ribosomes and initiate translation from non-AUG codons [Citation134], providing fresh insights into the fundamental translation mechanisms.
Unlike IGR IRES, viral mRNA translation directed by IRES requires eIFs and IRES-transacting factors (ITAFs), which can affect the recruitment of ribosomes or modify the structure of IRES, then regulate the activity of IRES. Several RNA-binding proteins (RBPs) for picornavirus infection have been identified by modulating the IRES activity of 5' UTR. For example, a peptide factor named p52 in HeLa cells was first identified through binding to IRES of PV to regulate the activity [Citation135]. Subsequently, p52 was further determined to be La autoantigen, which stimulates mRNA translation of PV [Citation136]. A series of heterogeneous nuclear ribonucleoprotein (hnRNP) family members have been identified as RBPs in recent years. These proteins are involved in nucleic acid metabolisms processes, such as mRNA splicing and regulation of transcription or translation [Citation137]. Although hnRNPs share some commonalities, the regulatory mechanisms vary between picornavirus. Such as, HnRNP I is the first reported ITAF with four RBDs that bind to EMCV IRES [Citation138]. The RBDs of hnRNP I bind to EMCV IRES in different orientations, with RBD 1 and 2 binding at the 3' end and RBD 3 preferring to bind to the 5' end of IRES, and this specificity of binding orientation contributes to stabilizing the IRES fold conformation [Citation139]. Both hnRNP L [Citation140] and hnRNP K [Citation141] show subcellular localization changes during infection with FMDV. HnRNP L inhibits viral replication by binding to FMDV IRES, and this inhibitory effect is also seen in Bovine enterovirus (BeV) [Citation140]. However, hnRNP L does not affect EMCV replication, suggesting that regulatory effect varies between picornavirus. Interestingly, the negative effect of hnRNP L on FMDV replication was not through translational inhibition but rather by staying in the replication complex and interacting non-directly with 3Dpol to inhibit viral RNA synthesis [Citation140]. Furthermore, the KH2 and KH3 domains of hnRNP K can directly bind to the IRES, inhibiting the translation by interfering with the recognition of the IRES by hnRNP I, resulting in a ~ 10-fold decrease in the production of infectious progeny. However, this inhibition can be antagonized by the 3C protease [Citation141]. In addition, hnRNP A1 acts as an ITAF for EV71 IRES, interacts explicitly with stem-loop II of IRES, and localizes to the cytoplasm to recruit 40S ribosomal subunits [Citation142]. Further studies revealed that AUAGC in stem-loop II is a bulge sequence. When mutated to ACCCC, eliminating the bulge resulted in a conformational change in IRES that completely impaired viral translation and inhibited viral replication [Citation143].
Except for the hnRNP family members, other host proteins such as SRp20 [Citation144], Gemin5 [Citation145], DDX21 [Citation146], and nucleolin [Citation147] have also been shown to be involved in regulating IRES. SRp20 can interact with PCBP2, the known ITAF of PV IRES, and interference with SRp20 expression can correspondingly reduce the translation of PV IRES. The interaction of Gemin5 with IRES reveals that the orientation of protein binding also affects the function of IRES, with Gemin5 binding to IRES primarily through the C-terminus. The regulation of NCL on IRES is conservative. NCL regulates not only FMDV but also the translation and infection of SVA and classical swine fever virus (CSFV). Additionally, NCL affects FMDV replication by directly regulating the production of viral proteins and indirectly regulating the synthesis of viral RNA. Interference with the expression of NCL can reduce the replication efficiency of FMDV replicators by about 50%. Meanwhile, several eIFs (e.g. eIF2, eIF3, eIF4A, eIF4G, eIF4B, and eIF1A) are also involved in regulating IRES [Citation111]. It is essential to mention that the GNRA motif on the bulge loop of IRES also plays an essential role in IRES activity. When GAGA is mutated to CAGU or GAAA, it alters the local RNA structure of this region and reduces the interaction with trans-acting factors, like La autoantigen, thus reducing the IRES activity [Citation148].
4. The biological significance of viral 5' UTR element
The function of the untranslated regions of the picornavirus varies depending on the virus. Usually, it includes the following aspects: (i) Regulation of viral gene expression. Specific motifs in the 5' UTR can regulate the transcription and translation of viral genes. (ii) Maintain the stability of the viral genome. Considering that the picornavirus genome is a naked and uncapped positive-stranded RNA, the RNA structure at the 5' end of the genome typically protects the viral genome from nuclease degradation. (iii) Specific motifs of 5' UTR can interact with host proteins to affect virus pathogenicity and even evade antiviral immunity.
4.1. The 5' UTR elements are involved in the viral genomic replication and synthesis
Upon picornavirus infection, the various RNA structures of the 5' UTR act as scaffolds to organize multiple factors, regulating the initiation of RNA synthesis. The 5' UTR of poliovirus (PV) was initially found to affect viral replication and translation using a well-established cell-free system [Citation149]. A typical example is that the 3' UTR of PV alone cannot recruit proteins for negative-stranded RNA synthesis. In contrast, the cloverleaf in the 5' UTR is required to form a functional pre-initiation RNA replication complex and initiate negative-strand synthesis [Citation72,Citation150]. In addition to the two ends of the viral genome, several viral and host proteins are involved in forming the RNP complex. The proteins bound to the two ends of the genome likely interact to form a protein bridge that can be used to form the circular RNP complex [Citation72]. For instance, PCBP can bind to 5' cloverleaf, and PABP can bind to the 3' poly (A) tail, both of which, in turn, can bind to each other in an RNA-dependent manner [Citation72,Citation151,Citation152]. This interaction may contribute to stabilizing the RNP complex and the cyclization of the genome. Additionally, 3CD can bind to the 5' UTR and 3' UTR, respectively. It may also have a role in the formation and stabilization of the RNP complex [Citation72], suggesting 5' UTR and 3' UTR synergistically and spatially mediate the genomic replication of viral RNA.
Disruption of the cloverleaf structure affects the stability of viral RNA and subsequent translation and replication. The viral polymerase alone cannot remove the translation ribosome from the RNA template and directly mediate the translation switch to negative-strand RNA synthesis [Citation153]. PCBP binds both cloverleaf and IRES, and PCBP binds more preferentially to cloverleaf in 3CD [Citation154,Citation155]. This binding preference suggests that it may inhibit the translation initiation process and play a vital role in this step of the switch to the RNA replication process. As expected, mutations in cloverleaf could affect the feedback of translation initiation inhibition, indirectly affecting viral negative-strand RNA synthesis [Citation156]. The intact cloverleaf is also required for positive-strand synthesis as it is the template used to generate the complementary negative strand 3' cloverleaf during negative-strand RNA synthesis, the site where positive-strand RNA synthesis begins [Citation157]. Considering the similarity of the localization of different RNA elements in the genome, the S fragment of FMDV may have an analogous function, but it is yet to be confirmed. In addition, a conserved cis-acting element in the coxsackievirus B3 (CVB3) genome, which known as a cryptic AUG. Since 48S ribosomal assembly occurs just upstream of the cryptic AUG, it is expected that mutations in cryptic AUG would affect the translation efficiency of IRES [Citation158]. The introduction of a substitution mutation at position 585C-A to generate additional cryptic AUG to re-establish IRES function or a mutation at position 617A-U to restore complementary base-pairing did not restore the translation efficiency of IRES, although both mutations were structurally back-compensated. All these data suggest that the integrity of the cryptic AUG is pivotal and that this integrity may correlate with the interactions of ITAFs required for ribosome assembly [Citation158]. During infection by group -B enteroviruses (EV-B), domain I (also known as cloverleaf) in the 5' UTR interacts with specific proteins to form RNP complexes that regulate viral genome replication. Therefore, losing some secondary structures in domain I can impair the binding of untranslated regions by viral or host proteins, thus affecting EV-B replication and infectivity. The natural nucleotide deletion of domain I may be a crucial link between domain I-cellular protein interactions, which can lead to acute or persistent enterovirus infection injury in patients with viral infection [Citation159]. The underlying mechanism of dilated cardiomyopathy caused by persistent infection of cardiac tissue by enterovirus is also associated with genomic 5' end deletions. It has been found that cardiac pathogenesis is associated with viral protease 2A and that deletion of the 5' terminal elements reduces the level of 2A protease activity [Citation160,Citation161]. These genomic RNAs with a deficient 5' terminal element are associated with a low level of the full-length enterovirus genome, promoting infection persistence and limiting viral particle production. However, even without infectious viral particle production, the viral protease levels expressed by these incomplete RNA genomes can still cause viral pathology [Citation161].
4.2. The 5' UTR element contribute to viral virulence, pathogenicity, and tropism
The accumulated evidence identified that the alterations in motif or structure of 5' UTR may impact tissue and even host tropism, indicating that the 5' UTR element is also a genetic determinant for virulence and pathogenicity. IRES is an RNA element common to picornavirus. Mutations in IRES also affect virulence and tropism rather than attracting ribosomal subunits and directing them to the correct translation initiation codon. A classic example is a C-to-U mutation at position 472 of the IRES of poliovirus Sabin 3 vaccine strains results in the decrease of neuro-virulence and the restriction of the virus histophilicity [Citation162]. Opposite mutation results were found in coxsackievirus B1 (CVB1). The synergistic mutations in both A579G and U573A of stem-loop H of CVB1 led to a significant increase in virulence and pathogenesis in mice, indicating that this domain of IRES is a determinant of virulence [Citation163]. Additionally, nucleotide insertion in the stem-loop H of the 5' UTR can attenuate neuro-virulence for Theiler's murine encephalomyelitis viruses (TMEV), implying that nucleotide insertion may have inhibited the ability to synthesize viral proteins [Citation164]. The chimeric PV with HRV2 IRES eliminated the neuro-pathogenic phenotype in CD155 transgenic mice (Poliomyelitis model mice) and Cynomolgus monkeys [Citation165]. Furthermore, the structural analysis showed that domains V and VI of IRES cooperated to determine the neuro-pathogenicity of the chimeric PV [Citation165]. Interestingly, mutations in linking sequences between different RNA elements can also affect virulence. For instance, the substitution of 27 nt introduced as a genetic marker in a synthetic PV1(M) [sPV1(M)] strain where the UA-to-GG exchanges of nucleotides 102/103 maps to a region between the cloverleaf and IRES generate a phenotype with highly attenuated neuro-virulence in mice [Citation166].
In addition to affecting virulence, deleting some nucleotides in the 5' UTR may also lead to changes in viral host tropism. Compared to the wild-type FMDV strain, chimeric FMDV, which has the IRES of bovine rhinitis B virus (BRBV), showed less replication characterization in a porcine-derived cell model [Citation167]. Furthermore, the 43-nt and 86-nt deletion of pseudoknots significantly reduced the pathogenicity to cattle, consistent with a porcinophilic phenotype of field-isolated Pan-Asian FMDV strains carrying an 86-nt deletion [Citation69]. Similarly, 70-nt nucleotide deletions in the terminal S fragment at the FMDV 5' UTR made the pigs more susceptible than cattle [Citation69,Citation73].
4.3. The reciprocal interaction among 5' UTR elements of picornavirus and host immunity modules
The host immunity response triggered by pathogen infections is an essential defense system of the host against pathogens. Type I interferon (IFN-I) signaling is the core of the host's innate antiviral immune response. Once the receptor senses the pathogen-associated PAMPs, IFN-I is activated and induces the expression of IFN-stimulated genes (ISGs), which activates the host immunity response. To successfully produce the progeny, RNA viruses utilize various mechanisms to interfere with or escape the host immunity. Previous studies have shown that the 5' UTR of picornavirus is also involved in the reciprocal interaction with host immunity molecules. For instance, the 5' UTR and 3' UTR transcripts of FMDV induced an innate immune response in SK-6 cells, with the 3' UTR having the more potent effect, followed by the S fragment and IRES [Citation168]. The S fragment induced about a 10-fold increase in the transcription level of IFN-β, while IRES induced only slightly higher levels than poly (I:C). Meanwhile, the S fragment also plays an essential role in the overall 5' UTR-induced IFN response, and the effect of induction is more substantial when S is present alone [Citation168]. Mechanistically, S fragment transcripts can activate the promoter of IFN-β via a RIG-I-dependent pathway, suggesting that the RLR pathway is involved in sensing ncRNA (non-coding RNA) by RIG-I signaling in cells. IRES also efficiently induced an antiviral response in PBMC cells, and Mx1 mRNA transcription was readily detected in PBMCs transfected with IRES transcripts. Additionally, the supernatant of PBMCs transfected with IRES transcripts was also found to significantly inhibit FMDV infection when tested for antiviral activity against IBRS-2 cells. Moreover, high TNF-α, IL-10, and IL-12 expression levels were also detected [Citation169]. Meanwhile, compared to wild-type FMDV, the infection of mutant viruses with S fragment-specific internal deletion (104–267 nt) results in the mRNA levels upregulation of IFN and two ISGs (Mx1 and IRF7) [Citation74], indicating that the different S-fragment recombinant FMDV confer the distinct ability to regulate the host innate immune response. Importantly, partial deletion of the S fragment exhibited a highly attenuated phenotype. Nevertheless, inoculated animals produced protective antibodies and did not develop viremia when exposed to the lethal dose of wild-type FMDV.
Additionally, the MDA5-specific patterns of type-I antiviral signaling antagonized recombinant PV (PVSRIPO) infection by binding of host double-stranded RNA-activated protein kinase (PKR) to the viral IRES [Citation170]. Mechanistically, PKR negatively regulates viral early viral translation by interacting with domains V and VI of IRES, which also can directly recruit dsRNA receptors such as ADAR1. The binding of PKR to IRES would induce auto-phosphorylation of its activation loop, and PKR could displace the recruitment of eIF4G:eIF4A, a possible reason for the early disruption of PVSRIPO translation [Citation170].
5. Conclusions and perspectives
Picornavirus is a class of highly pathogenic viruses with a small diameter and broad host range. Although poliovirus has been eradicated in most countries except Africa, viruses such as EV71, EMCV, and FMDV continue to cause health problems for humans and livestock. In recent years, due to the constant breakthrough of molecular biology techniques, the research on the structural organization and function of the viral genome has brought about an insightful understanding of viral RNA synthesis, protein translation, and viral assembly. Compared with the coding region of the viral genome, untranslated regions have been less clarified, but they usually contain essential RNA structural elements that determine viral replication and translation. Given the vital significance of the 5'UTR elements of picornavirus, we present a brief overview of the structural characterization and biological significance of 5' UTR in the current review.
Throughout the current research on viral UTR of picornavirus, it is easy to find that there is still room for improvement due to various limitations of the research subjects and experimental methods. Several vital scientific questions remain to be further elucidated. First, what is the specific molecular mechanism of picornavirus' UTR in host adaptation? Second, what functional structures are involved in coordinating the transfer and transmission of the picornavirus among different hosts? Third, given that the absence of some elements in the 5' UTR of picornavirus cause viral virulence attenuation, what is the underlying mechanism? Could these viral elements be applied to the design of attenuated vaccines for picornavirus? Finally, the viral positive- and negative-strand RNA synthesis is tightly associated with the 5' UTR-terminal RNA elements, making them another possible target for virus control. For instance, IRES-mediated translation initiation mediated differs from the eukaryotic cap-dependent translation mechanism. Moreover, translation initiation is the pivotal step of gene expression after viral RNA enters the host cell, suggesting that IRES is a potential therapeutic or drug target. Studying these issues sheds light on understanding the sophisticated picornavirus life cycle and provides potential targets for designing gene and antisense nucleic acid drugs.
Extensive improvement of our understanding of the biological characteristics and function of picornavirus' UTR must depend intensely on the innovative application of new techniques and detective methods. Firstly, given the complexity of RNA folding, the functional study of 5' UTR should be closely integrated with interdisciplinary cooperation such as structural biology. For instance, the RdRp catalytic mechanism of EV71 has been further elucidated with the assistance of enzymology and crystal structure biology [Citation171]. Secondly, developing new RNA probing technology can also promote the discovery of novel RNA elements. The structural complexity of the 5' UTR limits the research depth, but the development of structure-probing techniques, such as SHAPE [Citation172], helps to compensate for this. Researchers should keep a closer eye on advanced technological breakthroughs and integrate these into a thorough understanding of RNA-protein interaction.
Author contributions
T.P. and F.Y. write the manuscript draft. F.Y., W.C., H.Z., and X.Z. helped revise the manuscript. All the authors contributed to the article and approved the submitted version.
Disclosure statement
No potential conflict of interest was reported by the author(s).
Additional information
Funding
References
- Li K, Wang C, Yang F, et al. Virus-host interactions in foot-and-mouth disease virus infection. Front Immunol. 2021;12:571509. doi: 10.3389/fimmu.2021.571509
- Zhang M, Hill JE, Fernando C, et al. Respiratory viruses identified in western Canadian beef cattle by metagenomic sequencing and their association with bovine respiratory disease. Transbound Emerg Dis. 2019;66(3):1379–1386. doi: 10.1111/tbed.13172
- Zhou Y, Chen X, Tang C, et al. Detection and genomic characterization of bovine rhinitis virus in China. Animals (Basel). 2023;13(2):312. doi: 10.3390/ani13020312
- Bhattarai S, Lin CM, Temeeyasen G, et al. Bovine rhinitis B virus is highly prevalent in acute bovine respiratory disease and causes upper respiratory tract infection in calves. J Gen Virol. 2022;103(2): doi: 10.1099/jgv.0.001714
- Zhai SL, Xie YL, Zhai Q, et al. Genome characterization and phylogenetic analysis of the first bovine rhinitis B virus isolate in China. Front Vet Sci. 2021;8:721284. doi:10.3389/fvets.2021.721284
- Diaz-Méndez A, Viel L, Shewen P, et al. Genomic analysis of a Canadian equine rhinitis a virus reveals low diversity among field isolates. Virus Genes. 2013;46(2):280–286. doi: 10.1007/s11262-012-0848-0
- Rossi TM, Moore A, O’ Sullivan TL, et al. Risk factors for duration of equine rhinitis a virus respiratory disease. Equine Vet J. 2020;52(3):369–373. doi: 10.1111/evj.13204
- Lynch SE, Gilkerson JR, Symes SJ, et al. Persistence and chronic urinary shedding of the aphthovirus equine rhinitis a virus. Comp Immunol Microbiol Infect Dis. 2013;36(1):95–103. doi: 10.1016/j.cimid.2012.10.003
- Kapoor A, Victoria J, Simmonds P, et al. A highly divergent picornavirus in a marine mammal. J Virol. 2008;82(1):311–320. doi: 10.1128/JVI.01240-07
- Yugo DM, Hauck R, Shivaprasad HL, et al. Hepatitis virus infections in poultry. Avian Dis. 2016;60(3):576–588. doi: 10.1637/11229-070515-Review.1
- Yehia N, Erfan AM, Omar SE, et al. Dual circulation of duck hepatitis A virus genotypes 1 and 3 in Egypt. Avian Dis. 2021;65(1):1–9. doi: 10.1637/aviandiseases-D-20-00075
- Carocci M, Bakkali-Kassimi L. The encephalomyocarditis virus. Virulence. 2012;3(4):351–367. doi: 10.4161/viru.20573
- Doysabas KCC, Oba M, Furuta M, et al. Encephalomyocarditis virus is potentially derived from eastern bent-wing bats living in East Asian countries. Virus Res. 2019;259:62–67. doi:10.1016/j.virusres.2018.10.020
- Liang Z, Kumar AS, Jones MS, et al. Phylogenetic analysis of the species theilovirus: emerging murine and human pathogens. J Virol. 2008;82(23):11545–11554. doi: 10.1128/JVI.01160-08
- Tan SZ, Tan MZ, Prabakaran M. Saffold virus, an emerging human cardiovirus. Rev Med Virol. 2017;27(1):27. doi: 10.1002/rmv.1908
- Himeda T, Ohara Y. Saffold virus, a novel human cardiovirus with unknown pathogenicity. J Virol. 2012;86(3):1292–1296. doi: 10.1128/JVI.06087-11
- Reuter G, Boros Á, Földvári G, et al. Dicipivirus (family Picornaviridae) in wild Northern white-breasted hedgehog (Erinaceus roumanicus). Arch Virol. 2018;163(1):175–181. doi: 10.1007/s00705-017-3565-0
- Yoshida H. Human enterovirus. Nihon Rinsho. 2003;61(Suppl 3):463–467.
- Nasri D, Bouslama L, Pillet S, et al. Basic rationale, current methods and future directions for molecular typing of human enterovirus. Expert Rev Mol Diagn. 2007;7(4):419–434. doi: 10.1586/14737159.7.4.419
- Mosena ACS, da Silva MS, Gularte JS, et al. Genome sequence of a Brazilian bovine enterovirus. Microbiol Resour Announc. 2022;11(2):e0120021. doi: 10.1128/mra.01200-21
- Ji C, Zhang Y, Sun R, et al. Isolation and identification of type F bovine enterovirus from clinical cattle with diarrhoea. Viruses. 2021;13(11):2217. doi: 10.3390/v13112217
- Moon HJ, Song D, Seon BH, et al. Complete genome analysis of porcine enterovirus B isolated in Korea. J Virol. 2012;86(18):10250. doi: 10.1128/JVI.01548-12
- Oberste MS, Maher K, Pallansch MA. Molecular phylogeny and proposed classification of the simian picornaviruses. J Virol. 2002;76(3):1244–1251. doi: 10.1128/JVI.76.3.1244-1251.2002
- Rollinger JM, Schmidtke M. The human rhinovirus: human-pathological impact, mechanisms of antirhinoviral agents, and strategies for their discovery. Med Res Rev. 2011;31(1):42–92. doi: 10.1002/med.20176
- Jacobs SE, Lamson DM, St George K, et al. Human rhinoviruses. Clin Microbiol Rev. 2013;26(1):135–162. doi: 10.1128/CMR.00077-12
- Huang JA, Ficorilli N, Hartley CA, et al. Equine rhinitis B virus: a new serotype. J Gen Virol. 2001;82(11):2641–2645. doi: 10.1099/0022-1317-82-11-2641
- Pintó RM, Pérez-Rodríguez FJ, Costafreda MI, et al. Pathogenicity and virulence of hepatitis a virus. Virulence. 2021;12(1):1174–1185. doi: 10.1080/21505594.2021.1910442
- Feinstone SM. History of the discovery of hepatitis a virus. Cold Spring Harb Perspect Med. 2019;9(5):a031740. doi: 10.1101/cshperspect.a031740
- Abi KM, Yang C, Tang C, et al. Aichivirus C isolate is a diarrhoea-causing pathogen in goats. Transbound Emerg Dis. 2022;69(5):e2268–e75. doi: 10.1111/tbed.14566
- Abi KM, Yu Z, Jing ZZ, et al. Identification of a novel Aichivirus D in sheep. Infect Genet Evol. 2021;91:104810. doi:10.1016/j.meegid.2021.104810
- Barbknecht M, Sepsenwol S, Leis E, et al. Characterization of a new picornavirus isolated from the freshwater fish Lepomis macrochirus. J Gen Virol. 2014;95(3):601–613. doi: 10.1099/vir.0.061960-0
- Lange J, Groth M, Fichtner D, et al. Virus isolate from carp: genetic characterization reveals a novel picornavirus with two aphthovirus 2A-like sequences. J Gen Virol. 2014;95(1):80–90. doi: 10.1099/vir.0.058172-0
- Phelps NB, Mor SK, Armien AG, et al. Isolation and molecular characterization of a novel picornavirus from baitfish in the USA. PLoS One. 2014;9(2):e87593. doi: 10.1371/journal.pone.0087593
- Kabuga AI, Nejati A, Soheili P, et al. Human parechovirus are emerging pathogens with broad spectrum of clinical syndromes in adults. J Med Virol. 2020;92(12):2911–2916. doi: 10.1002/jmv.26395
- Olijve L, Jennings L, Walls T. Human parechovirus: an increasingly recognized cause of sepsis-like illness in young infants. Clin Microbiol Rev. 2018;31(1):31. doi: 10.1128/CMR.00047-17
- Lundstig A, McDonald SL, Maziarz M, et al. Neutralizing Ljungan virus antibodies in children with newly diagnosed type 1 diabetes. J Gen Virol. 2021;102(5): doi: 10.1099/jgv.0.001602
- Salisbury AM, Begon M, Dove W, et al. Ljungan virus is endemic in rodents in the UK. Arch Virol. 2014;159(3):547–551. doi: 10.1007/s00705-013-1731-6
- Fichtner D, Philipps A, Groth M, et al. Characterization of a novel picornavirus isolate from a diseased European eel (Anguilla). J Virol. 2013;87(19):10895–10899. doi: 10.1128/JVI.01094-13
- Hahn MA, Dheilly NM, Pfeiffer JK. Genome characterization, prevalence, and transmission mode of a novel picornavirus associated with the threespine stickleback fish (Gasterosteus aculeatus). J Virol. 2019;93(9). doi: 10.1128/JVI.02277-18
- Ibrahim YM, Zhang W, Werid GM, et al. Isolation, characterization, and molecular detection of porcine sapelovirus. Viruses. 2022;14(2):14. doi: 10.3390/v14020349
- Ray PK, Desingu PA, Kumari S, et al. Porcine sapelovirus among diarrhoeic piglets in India. Transbound Emerg Dis. 2018;65(1):261–263. doi: 10.1111/tbed.12628
- Zhang W, Kataoka M, Doan HY, et al. Characterization of a novel simian sapelovirus isolated from a cynomolgus monkey using PLC/PRF/5 cells. Sci Rep. 2019;9(1):20221. doi: 10.1038/s41598-019-56725-z
- Zhang X, Zhu Z, Yang F, et al. Review of Seneca Valley virus: a call for increased surveillance and research. Front Microbiol. 2018;9:940. doi:10.3389/fmicb.2018.00940
- Ray PK, Desingu PA, Anoopraj R, et al. Identification and genotypic characterization of porcine teschovirus from selected pig populations in India. Trop Anim Health Prod. 2020;52(3):1161–1166. doi: 10.1007/s11250-019-02114-7
- Sawant PM, Atre N, Kulkarni A, et al. Detection and molecular characterization of porcine enterovirus G15 and teschovirus from India. Pathog Dis. 2020;78(5). doi: 10.1093/femspd/ftaa039
- Salles MW, Scholes SF, Dauber M, et al. Porcine teschovirus polioencephalomyelitis in western Canada. J Vet Diagn Invest. 2011;23(2):367–373. doi: 10.1177/104063871102300231
- Hauck R, Sentíes-Cué CG, Wang Y, et al. Evolution of avian encephalomyelitis virus during embryo-adaptation. Vet Microbiol. 2017;204:1–7. doi:10.1016/j.vetmic.2017.04.005
- Goto Y, Yaegashi G, Kumagai Y, et al. Detection of avian encephalomyelitis virus in chickens in Japan using RT-PCR. J Vet Med Sci. 2019;81(1):103–106. doi: 10.1292/jvms.18-0550
- Welchman Dde B, Cox WJ, Gough RE, et al. Avian encephalomyelitis virus in reared pheasants: a case study. Avian Pathol. 2009;38(3):251–256. doi: 10.1080/03079450902912168
- Zell R, Delwart E, Gorbalenya AE, et al. ICTV virus taxonomy profile: picornaviridae. J Gen Virol. 2017;98(10):2421–2422. doi: 10.1099/jgv.0.000911
- Hargitai R, Pankovics P, Boros Á, et al. Novel picornavirus (family Picornaviridae) from freshwater fishes (Perca fluviatilis, Sander lucioperca, and Ameiurus melas) in Hungary. Arch Virol. 2021;166(9):2627–2632. doi: 10.1007/s00705-021-05167-y
- Gorbalenya AE, Krupovic M, Mushegian A. The new scope of virus taxonomy: partitioning the virosphere into 15 hierarchical ranks. Nat Microbiol. 2020;5(5):668–674. doi: 10.1038/s41564-020-0709-x
- Zell R. Picornaviridae—the ever-growing virus family. Arch Virol. 2018;163(2):299–317. doi: 10.1007/s00705-017-3614-8
- Jacobson MF, Baltimore D. Polypeptide cleavages in the formation of poliovirus proteins. Proc Natl Acad Sci U S A. 1968;61(1):77–84. doi: 10.1073/pnas.61.1.77
- Kiehn ED, Holland JJ. Synthesis and cleavage of enterovirus polypeptides in mammalian cells. J Virol. 1970;5(3):358–367. doi: 10.1128/jvi.5.3.358-367.1970
- Sun Y, Guo Y, Lou Z. Formation and working mechanism of the picornavirus VPg uridylylation complex. Curr Opin Virol. 2014;9:24–30. doi: 10.1016/j.coviro.2014.09.003
- Paul AV, Wimmer E. Initiation of protein-primed picornavirus RNA synthesis. Virus Res. 2015;206:12–26. doi: 10.1016/j.virusres.2014.12.028
- Pathak HB, Oh HS, Goodfellow IG, et al. Picornavirus genome replication: roles of precursor proteins and rate-limiting steps in oriI-dependent VPg uridylylation. J Biol Chem. 2008;283(45):30677–30688. doi: 10.1074/jbc.M806101200
- Rodrigues TCS, Nielsen O, Burek-Huntington KA, et al. Genomic characterization of picornaviruses isolated from ribbon (Histriophoca fasciata) and harbor (Phoca vitulina) seals. Front Vet Sci. 2020;7:554716. doi:10.3389/fvets.2020.554716
- Nayak A, Goodfellow IG, Belsham GJ. Factors required for the uridylylation of the foot-and-mouth disease virus 3B1, 3B2, and 3B3 peptides by the RNA-dependent RNA polymerase (3Dpol) in vitro. J Virol. 2005;79(12):7698–7706. doi: 10.1128/JVI.79.12.7698-7706.2005
- Melchers WJ, Hoenderop JG, Bruins Slot HJ, et al. Kissing of the two predominant hairpin loops in the coxsackie B virus 3’ untranslated region is the essential structural feature of the origin of replication required for negative-strand RNA synthesis. J Virol. 1997;71(1):686–696. doi: 10.1128/jvi.71.1.686-696.1997
- Duque H, Palmenberg AC. Phenotypic characterization of three phylogenetically conserved stem-loop motifs in the mengovirus 3′ untranslated region. J Virol. 2001;75(7):3111–3120. doi: 10.1128/JVI.75.7.3111-3120.2001
- Penza V, Russell SJ, Schulze AJ, et al. The long-lasting enigma of polycytidine (polyC) tract. PLOS Pathog. 2021;17(8):e1009739. doi: 10.1371/journal.ppat.1009739
- Staple DW, Butcher SE. Pseudoknots: RNA structures with diverse functions. PLoS Biol. 2005;3(6):e213. doi: 10.1371/journal.pbio.0030213
- Herold J, Andino R. Poliovirus RNA replication requires genome circularization through a protein–protein bridge. Mol Cell. 2001;7(3):581–591. doi: 10.1016/S1097-2765(01)00205-2
- Lee KM, Chen CJ, Shih SR. Regulation mechanisms of viral IRES-driven translation. Trends Microbiol. 2017;25(7):546–561. doi: 10.1016/j.tim.2017.01.010
- Palmenberg AC, Osorio JE. Cardioviral poly(C) tracts and viral pathogenesis. Arch Virol Suppl. 1994;9:67–77.
- Bae YS, Kang Y, Ohtsuka E, et al. Development of a recombinant RNA technique for the construction of chimeric RNA with a long poly(C) tract. Nucleic Acids Res. 1993;21(11):2703–2708. doi: 10.1093/nar/21.11.2703
- Zhu Z, Yang F, Cao W, et al. The pseudoknot region of the 5′ untranslated region is a determinant of viral tropism and virulence of foot-and-mouth disease virus. J Virol. 2019;93(8): doi: 10.1128/JVI.02039-18
- Hertz MI, Thompson SR. Mechanism of translation initiation by dicistroviridae IGR IRESs. Virology. 2011;411(2):355–361. doi: 10.1016/j.virol.2011.01.005
- Leppek K, Das R, Barna M. Functional 5′ UTR mRNA structures in eukaryotic translation regulation and how to find them. Nat Rev Mol Cell Biol. 2018;19(3):158–174. doi: 10.1038/nrm.2017.103
- Barton DJ, O’ Donnell BJ, Flanegan JB. 5′ cloverleaf in poliovirus RNA is a cis-acting replication element required for negative-strand synthesis. Embo J. 2001;20(6):1439–1448. doi: 10.1093/emboj/20.6.1439
- Yang F, Zhu Z, Cao W, et al. Genetic determinants of altered virulence of type O foot-and-mouth disease virus. J Virol. 2020;94(7): doi: 10.1128/JVI.01657-19
- Kloc A, Diaz-San Segundo F, Schafer EA, et al. Foot-and-mouth disease virus 5' -terminal S fragment is required for replication and modulation of the innate immune response in host cells. Virology. 2017;512:132–143. doi:10.1016/j.virol.2017.08.036
- Nagashima S, Sasaki J, Taniguchi K. The 5′-terminal region of the Aichi virus genome encodes cis -acting replication elements required for positive- and negative-strand RNA synthesis. J Virol. 2005;79(11):6918–6931. doi: 10.1128/JVI.79.11.6918-6931.2005
- Mason PW, Bezborodova SV, Henry TM. Identification and characterization of a cis-acting replication element (cre) adjacent to the internal ribosome entry site of foot-and-mouth disease virus. J Virol. 2002;76(19):9686–9694. doi: 10.1128/JVI.76.19.9686-9694.2002
- Steil BP, Barton DJ. Cis-active RNA elements (CREs) and picornavirus RNA replication. Virus Res. 2009;139(2):240–252. doi: 10.1016/j.virusres.2008.07.027
- Hollister JR, Vagnozzi A, Knowles NJ, et al. Molecular and phylogenetic analyses of bovine rhinovirus type 2 shows it is closely related to foot-and-mouth disease virus. Virology. 2008;373(2):411–425. doi: 10.1016/j.virol.2007.12.019
- Harris TJ, Brown F. Biochemical analysis of a virulent and an avirulent strain of foot-and-mouth disease virus. J Gen Virol. 1977;34(1):87–105. doi: 10.1099/0022-1317-34-1-87
- Escarmís C, Toja M, Medina M, et al. Modifications of the 5' untranslated region of foot-and-mouth disease virus after prolonged persistence in cell culture. Virus Res. 1992;26(2):113–125. doi: 10.1016/0168-1702(92)90151-X
- Black DN, Stephenson P, Rowlands DJ, et al. Sequence and location of the poly C tract in aphtho- and cardiovirus RNA. Nucleic Acids Res. 1979;6(7):2381–2390. doi: 10.1093/nar/6.7.2381
- Brown F, Newman J, Stott J, et al. Poly(c) in animal viral RNAs. Nature. 1974;251(5473):342–344. doi: 10.1038/251342a0
- Rieder E, Bunch T, Brown F, et al. Genetically engineered foot-and-mouth disease viruses with poly(C) tracts of two nucleotides are virulent in mice. J Virol. 1993;67(9):5139–5145. doi: 10.1128/jvi.67.9.5139-5145.1993
- Hahn H, Palmenberg AC. Encephalomyocarditis viruses with short poly(C) tracts are more virulent than their mengovirus counterparts. J Virol. 1995;69(4):2697–2699. doi: 10.1128/jvi.69.4.2697-2699.1995
- Martin LR, Duke GM, Osorio JE, et al. Mutational analysis of the mengovirus poly(C) tract and surrounding heteropolymeric sequences. J Virol. 1996;70(3):2027–2031. doi: 10.1128/jvi.70.3.2027-2031.1996
- Duke GM, Palmenberg AC. Cloning and synthesis of infectious cardiovirus RNAs containing short, discrete poly(C) tracts. J Virol. 1989;63(4):1822–1826. doi: 10.1128/jvi.63.4.1822-1826.1989
- Martin LR, Neal ZC, McBride MS, et al. Mengovirus and encephalomyocarditis virus poly(C) tract lengths can affect virus growth in murine cell culture. J Virol. 2000;74(7):3074–3081. doi: 10.1128/JVI.74.7.3074-3081.2000
- Rietveld K, Van Poelgeest R, Pleij CW, et al. The tRNA-Uke structure at the 3′ terminus of turnip yellow mosaic virus RNA. Differences and similarities with canonical tRNA. Nucleic Acids Res. 1982;10(6):1929–1946. doi: 10.1093/nar/10.6.1929
- Brierley I, Pennell S, Gilbert RJ. Viral RNA pseudoknots: versatile motifs in gene expression and replication. Nat Rev Microbiol. 2007;5(8):598–610. doi: 10.1038/nrmicro1704
- Mans RM, Pleij CW, Bosch L. tRNA-like structures. Structure, function and evolutionary significance. Eur J Biochem. 1991;201(2):303–324. doi: 10.1111/j.1432-1033.1991.tb16288.x
- Gultyaev AP, Olsthoorn RC. A family of non-classical pseudoknots in influenza a and B viruses. RNA Biol. 2010;7(2):125–129. doi: 10.4161/rna.7.2.11287
- Moss WN, Dela-Moss LI, Priore SF, et al. The influenza a segment 7 mRNA 3′ splice site pseudoknot/hairpin family. RNA Biol. 2012;9(11):1305–1310. doi: 10.4161/rna.22343
- Kieft JS, Rabe JL, Chapman EG. New hypotheses derived from the structure of a flaviviral Xrn1-resistant RNA: conservation, folding, and host adaptation. RNA Biol. 2015;12(11):1169–1177. doi: 10.1080/15476286.2015.1094599
- Plant EP, Dinman JD. The role of programmed-1 ribosomal frameshifting in coronavirus propagation. Front Biosci. 2008;13(13):4873–4881. doi: 10.2741/3046
- Xie S, Chen XX, Qiao S, et al. Identification of the RNA pseudoknot within the 3′ end of the porcine reproductive and respiratory syndrome virus genome as a pathogen-associated molecular pattern to activate antiviral signaling via RIG-I and toll-like receptor 3. J Virol. 2018;92(12): doi: 10.1128/JVI.00097-18
- Wutz G, Auer H, Nowotny N, et al. Equine rhinovirus serotypes 1 and 2: relationship to each other and to aphthoviruses and cardioviruses. J Gen Virol. 1996;77(Pt 8):1719–1730. doi: 10.1099/0022-1317-77-8-1719
- Ward JC, Lasecka-Dykes L, Neil C, et al. The RNA pseudoknots in foot-and-mouth disease virus are dispensable for genome replication, but essential for the production of infectious virus. PLOS Pathog. 2022;18(6):e1010589. doi: 10.1371/journal.ppat.1010589
- Liu F, Wang N, Wang Q, et al. Motif mutations in pseudoknot stem I upstream of start codon in senecavirus a genome: impacts on activity of viral IRES and on rescue of recombinant virus. Vet Microbiol. 2021;262:109223. doi:10.1016/j.vetmic.2021.109223
- Gross L, Vicens Q, Einhorn E, et al. The IRES5′UTR of the dicistrovirus cricket paralysis virus is a type III IRES containing an essential pseudoknot structure. Nucleic Acids Res. 2017;45(15):8993–9004. doi: 10.1093/nar/gkx622
- Escarmís C, Dopazo J, Dávila M, et al. Large deletions in the 5′-untranslated region of foot-and-mouth disease virus of serotype C. Virus Res. 1995;35(2):155–167. doi: 10.1016/0168-1702(94)00091-P
- Mohapatra JK, Pawar SS, Tosh C, et al. Genetic characterization of vaccine and field strains of serotype a foot-and-mouth disease virus from India. Acta Virol. 2011;55(4):349–352. doi: 10.4149/av_2011_04_349
- Martin LR, Palmenberg AC. Tandem mengovirus 5' pseudoknots are linked to viral RNA synthesis, not poly(C)-mediated virulence. J Virol. 1996;70(11):8182–8186. doi: 10.1128/jvi.70.11.8182-8186.1996
- Haimov O, Sinvani H, Dikstein R. Cap-dependent, scanning-free translation initiation mechanisms. Biochim Biophys Acta. 2015;1849(11):1313–1318. doi: 10.1016/j.bbagrm.2015.09.006
- Fraser CS. Quantitative studies of mRNA recruitment to the eukaryotic ribosome. Biochimie. 2015;114:58–71. doi: 10.1016/j.biochi.2015.02.017
- La Teana A, Benelli D, Londei P, et al. Translation initiation in the crenarchaeon sulfolobus solfataricus: eukaryotic features but bacterial route. Biochem Soc Trans. 2013;41(1):350–355. doi: 10.1042/BST20120300
- Zhang H, Wang Y, Lu J. Function and evolution of upstream ORFs in Eukaryotes. Trends Biochem Sci. 2019;44(9):782–794. doi: 10.1016/j.tibs.2019.03.002
- Pelletier J, Sonenberg N. Internal initiation of translation of eukaryotic mRNA directed by a sequence derived from poliovirus RNA. Nature. 1988;334(6180):320–325. doi: 10.1038/334320a0
- Bailey JM, Tapprich WE. Structure of the 5′ nontranslated region of the coxsackievirus B3 genome: chemical modification and comparative sequence analysis. J Virol. 2007;81(2):650–668. doi: 10.1128/JVI.01327-06
- Niepmann M. Internal translation initiation of picornaviruses and hepatitis C virus. Biochim Biophys Acta. 2009;1789(9–10):529–541. doi: 10.1016/j.bbagrm.2009.05.002
- Gamarnik AV, Andino R. Interactions of viral protein 3CD and Poly(rC) binding protein with the 5′ untranslated region of the poliovirus genome. J Virol. 2000;74(5):2219–2226. doi: 10.1128/JVI.74.5.2219-2226.2000
- Sweeney TR, Abaeva IS, Pestova TV, et al. The mechanism of translation initiation on Type 1 picornavirus IRESs. Embo J. 2014;33(1):76–92. doi: 10.1002/embj.201386124
- Gosert R, Chang KH, Rijnbrand R, et al. Transient expression of cellular polypyrimidine-tract binding protein stimulates cap-independent translation directed by both picornaviral and flaviviral internal ribosome entry sites in vivo. Mol Cell Biol. 2000;20(5):1583–1595. doi: 10.1128/MCB.20.5.1583-1595.2000
- Kolupaeva VG, Hellen CU, Shatsky IN. Structural analysis of the interaction of the pyrimidine tract-binding protein with the internal ribosomal entry site of encephalomyocarditis virus and foot-and-mouth disease virus RNAs. RNA. 1996;2(12):1199–1212.
- Fernández-Miragall O, Martínez-Salas E. Structural organization of a viral IRES depends on the integrity of the GNRA motif. RNA. 2003;9(11):1333–1344. doi: 10.1261/rna.5950603
- de Quinto S L, Martínez-Salas E. Interaction of the eIF4G initiation factor with the aphthovirus IRES is essential for internal translation initiation in vivo. RNA. 2000;6(10):1380–1392. doi: 10.1017/S1355838200000753
- López de Quinto S, Lafuente E, Martínez-Salas E. IRES interaction with translation initiation factors: functional characterization of novel RNA contacts with eIF3, eIF4B, and eIF4GII. RNA. 2001;7(9):1213–1226. doi: 10.1017/S1355838201010433
- Pacheco A, López de Quinto S, Ramajo J, et al. A novel role for Gemin5 in mRNA translation. Nucleic Acids Res. 2009;37(2):582–590. doi: 10.1093/nar/gkn979
- Luz N, Beck E. Interaction of a cellular 57-kilodalton protein with the internal translation initiation site of foot-and-mouth disease virus. J Virol. 1991;65(12):6486–6494. doi: 10.1128/jvi.65.12.6486-6494.1991
- Andreev DE, Fernandez-Miragall O, Ramajo J, et al. Differential factor requirement to assemble translation initiation complexes at the alternative start codons of foot-and-mouth disease virus RNA. RNA. 2007;13(8):1366–1374. doi: 10.1261/rna.469707
- Cao X, Bergmann IE, Füllkrug R, et al. Functional analysis of the two alternative translation initiation sites of foot-and-mouth disease virus. J Virol. 1995;69(1):560–563. doi: 10.1128/jvi.69.1.560-563.1995
- Pisarev AV, Chard LS, Kaku Y, et al. Functional and structural similarities between the internal ribosome entry sites of hepatitis C virus and porcine teschovirus, a picornavirus. J Virol. 2004;78(9):4487–4497. doi: 10.1128/JVI.78.9.4487-4497.2004
- Hellen CU, de Breyne S. A distinct group of hepacivirus/pestivirus-like internal ribosomal entry sites in members of diverse picornavirus genera: evidence for modular exchange of functional noncoding RNA elements by recombination. J Virol. 2007;81(11):5850–5863. doi: 10.1128/JVI.02403-06
- Brown EA, Zhang H, Ping LH, et al. Secondary structure of the 5′ nontranslated regions of hepatitis C virus and pestivirus genomic RNAs. Nucleic Acids Res. 1992;20(19):5041–5045. doi: 10.1093/nar/20.19.5041
- Kieft JS, Zhou K, Jubin R, et al. The hepatitis C virus internal ribosome entry site adopts an ion-dependent tertiary fold. J Mol Biol. 1999;292(3):513–529. doi: 10.1006/jmbi.1999.3095
- Rijnbrand RC, Lemon SM. Internal ribosome entry site-mediated translation in hepatitis C virus replication. Curr Top Microbiol Immunol. 2000;242:85–116.
- Jubin R, Vantuno NE, Kieft JS, et al. Hepatitis C virus internal ribosome entry site (IRES) stem loop IIId contains a phylogenetically conserved GGG triplet essential for translation and IRES folding. J Virol. 2000;74(22):10430–10437. doi: 10.1128/JVI.74.22.10430-10437.2000
- Psaridi L, Georgopoulou U, Varaklioti A, et al. Mutational analysis of a conserved tetraloop in the 5′ untranslated region of hepatitis C virus identifies a novel RNA element essential for the internal ribosome entry site function. FEBS Lett. 1999;453(1–2):49–53. doi: 10.1016/S0014-5793(99)00662-6
- Ji H, Fraser CS, Yu Y, et al. Coordinated assembly of human translation initiation complexes by the hepatitis C virus internal ribosome entry site RNA. Proc Natl Acad Sci U S A. 2004;101(49):16990–16995. doi: 10.1073/pnas.0407402101
- Andreev DE, Niepmann M, Shatsky IN. Elusive trans-acting factors which operate with Type I (Poliovirus-like) IRES elements. Int J Mol Sci. 2022;23(24):23. doi: 10.3390/ijms232415497
- Kerr CH, Jan E, Pfeiffer J. Commandeering the ribosome: lessons learned from dicistroviruses about translation. J Virol. 2016;90(12):5538–5540. doi: 10.1128/JVI.00737-15
- Schüler M, Connell SR, Lescoute A, et al. Structure of the ribosome-bound cricket paralysis virus IRES RNA. Nat Struct Mol Biol. 2006;13(12):1092–1096. doi: 10.1038/nsmb1177
- Fernández IS, Bai XC, Murshudov G, et al. Initiation of translation by cricket paralysis virus IRES requires its translocation in the ribosome. Cell. 2014;157(4):823–831. doi: 10.1016/j.cell.2014.04.015
- Pfingsten JS, Costantino DA, Kieft JS. Structural basis for ribosome recruitment and manipulation by a viral IRES RNA. Science. 2006;314(5804):1450–1454. doi: 10.1126/science.1133281
- Wilson JE, Powell MJ, Hoover SE, et al. Naturally occurring dicistronic cricket paralysis virus RNA is regulated by two internal ribosome entry sites. Mol Cell Biol. 2000;20(14):4990–4999. doi: 10.1128/MCB.20.14.4990-4999.2000
- Meerovitch K, Pelletier J, Sonenberg N. A cellular protein that binds to the 5' -noncoding region of poliovirus RNA: implications for internal translation initiation. Genes Dev. 1989;3(7):1026–1034. doi: 10.1101/gad.3.7.1026
- Meerovitch K, Svitkin YV, Lee HS, et al. La Autoantigen enhances and corrects aberrant translation of poliovirus RNA in reticulocyte lysate. J Virol. 1993;67(7):3798–3807. doi: 10.1128/jvi.67.7.3798-3807.1993
- Geuens T, Bouhy D, Timmerman V. The hnRNP family: insights into their role in health and disease. Hum Genet. 2016;135(8):851–867. doi: 10.1007/s00439-016-1683-5
- Hellen CU, Wimmer E. Translation of encephalomyocarditis virus RNA by internal ribosomal entry. Curr Top Microbiol Immunol. 1995;203:31–63.
- Kafasla P, Morgner N, Pöyry TA, et al. Polypyrimidine tract binding protein stabilizes the encephalomyocarditis virus IRES structure via binding multiple sites in a unique orientation. Mol Cell. 2009;34(5):556–568. doi: 10.1016/j.molcel.2009.04.015
- Sun C, Liu M, Chang J, et al. Heterogeneous nuclear ribonucleoprotein l negatively regulates foot-and-mouth disease virus replication through inhibition of viral RNA synthesis by interacting with the internal ribosome entry site in the 5′ untranslated region. J Virol. 2020;94(10): doi: 10.1128/JVI.00282-20
- Liu W, Yang D, Sun C, et al. hnRNP K is a novel internal ribosomal entry site-transacting factor that negatively regulates foot-and-mouth disease virus translation and replication and is antagonized by viral 3C protease. J Virol. 2020;94(17): doi: 10.1128/JVI.00803-20
- Levengood JD, Tolbert M, Li ML, et al. High-affinity interaction of hnRNP A1 with conserved RNA structural elements is required for translation and replication of enterovirus 71. RNA Biol. 2013;10(7):1136–1145. doi: 10.4161/rna.25107
- Tolbert M, Morgan CE, Pollum M, et al. HnRNP A1 alters the structure of a conserved enterovirus IRES domain to stimulate viral translation. J Mol Biol. 2017;429(19):2841–2858. doi: 10.1016/j.jmb.2017.06.007
- Bedard KM, Daijogo S, Semler BL. A nucleo-cytoplasmic SR protein functions in viral IRES-mediated translation initiation. Embo J. 2007;26(2):459–467. doi: 10.1038/sj.emboj.7601494
- Piñeiro D, Fernández N, Ramajo J, et al. Gemin5 promotes IRES interaction and translation control through its C-terminal region. Nucleic Acids Res. 2013;41(2):1017–1028. doi: 10.1093/nar/gks1212
- Abdullah SW, Wu J, Zhang Y, et al. DDX21, a host restriction factor of FMDV IRES-dependent translation and replication. Viruses. 2021;13(9):1765. doi: 10.3390/v13091765
- Han S, Wang X, Guan J, et al. Nucleolin promotes IRES-Driven translation of foot-and-mouth disease virus by supporting the assembly of translation initiation complexes. J Virol. 2021;95(13):e0023821. doi: 10.1128/JVI.00238-21
- Bhattacharyya S, Das S. An apical GAGA loop within 5' UTR of the coxsackievirus B3 RNA maintains structural organization of the IRES element required for efficient ribosome entry. RNA Biol. 2006;3(2):60–68. doi: 10.4161/rna.3.2.2990
- Molla A, Paul AV, Wimmer E. Cell-free, de novo synthesis of poliovirus. Science. 1991;254(5038):1647–1651. doi: 10.1126/science.1661029
- Lyons T, Murray KE, Roberts AW, et al. Poliovirus 5′-terminal cloverleaf RNA is required in cis for VPg uridylylation and the initiation of negative-strand RNA synthesis. J Virol. 2001;75(22):10696–10708. doi: 10.1128/JVI.75.22.10696-10708.2001
- Wang Z, Day N, Trifillis P, et al. An mRNA stability complex functions with poly(A)-binding protein to stabilize mRNA in vitro. Mol Cell Biol. 1999;19(7):4552–4560. doi: 10.1128/MCB.19.7.4552
- Wang Z, Kiledjian M. The poly(A)-binding protein and an mRNA stability protein jointly regulate an endoribonuclease activity. Mol Cell Biol. 2000;20(17):6334–6341. doi: 10.1128/MCB.20.17.6334-6341.2000
- Barton DJ, Morasco BJ, Flanegan JB. Translating ribosomes inhibit poliovirus negative-strand RNA synthesis. J Virol. 1999;73(12):10104–10112. doi: 10.1128/JVI.73.12.10104-10112.1999
- Andino R, Rieckhof GE, Baltimore D. A functional ribonucleoprotein complex forms around the 5′ end of poliovirus RNA. Cell. 1990;63(2):369–380. doi: 10.1016/0092-8674(90)90170-J
- Andino R, Rieckhof GE, Trono D, et al. Substitutions in the protease (3Cpro) gene of poliovirus can suppress a mutation in the 5' noncoding region. J Virol. 1990;64(2):607–612. doi: 10.1128/jvi.64.2.607-612.1990
- Gamarnik AV, Andino R. Switch from translation to RNA replication in a positive-stranded RNA virus. Genes Dev. 1998;12(15):2293–2304. doi: 10.1101/gad.12.15.2293
- Sharma N, O’ Donnell BJ, Flanegan JB. 3′-terminal sequence in poliovirus negative-strand templates is the primary cis -acting element required for VPgpUpU-Primed positive-strand initiation. J Virol. 2005;79(6):3565–3577. doi: 10.1128/JVI.79.6.3565-3577.2005
- Verma B, Ponnuswamy A, Gnanasundram SV, et al. Cryptic AUG is important for 48S ribosomal assembly during internal initiation of translation of coxsackievirus B3 RNA. J Gen Virol. 2011;92(10):2310–2319. doi: 10.1099/vir.0.032151-0
- Glenet M, Heng L, Callon D, et al. Structures and functions of viral 5' non-coding genomic RNA domain-I in Group-B enterovirus infections. Viruses. 2020;12(9):919. doi: 10.3390/v12090919
- Badorff C, Lee GH, Lamphear BJ, et al. Enteroviral protease 2A cleaves dystrophin: evidence of cytoskeletal disruption in an acquired cardiomyopathy. Nat Med. 1999;5(3):320–326. doi: 10.1038/6543
- Bouin A, Vu MN, Al-Hakeem A, et al. Enterovirus-cardiomyocyte interactions: impact of terminally deleted genomic RNAs on viral and host functions. J Virol. 2023;97(1):e0142622. doi: 10.1128/jvi.01426-22
- Kauder SE, Racaniello VR. Poliovirus tropism and attenuation are determined after internal ribosome entry. J Clin Invest. 2004;113(12):1743–1753. doi: 10.1172/JCI200421323
- Zhong Z, Li X, Zhao W, et al. Mutations at nucleotides 573 and 579 within 5′-untranslated region augment the virulence of coxsackievirus B1. Virus Res. 2008;135(2):255–259. doi: 10.1016/j.virusres.2008.04.012
- Bandyopadhyay PK, Pritchard A, Jensen K, et al. A three-nucleotide insertion in the H stem-loop of the 5' untranslated region of Theiler’s virus attenuates neurovirulence. J Virol. 1993;67(6):3691–3695. doi: 10.1128/jvi.67.6.3691-3695.1993
- Gromeier M, Bossert B, Arita M, et al. Dual stem loops within the poliovirus internal ribosomal entry site control neurovirulence. J Virol. 1999;73(2):958–964. doi: 10.1128/JVI.73.2.958-964.1999
- De Jesus N, Franco D, Paul A, et al. Mutation of a single conserved nucleotide between the cloverleaf and internal ribosome entry site attenuates poliovirus neurovirulence. J Virol. 2005;79(22):14235–14243. doi: 10.1128/JVI.79.22.14235-14243.2005
- Sun C, Yang D, Gao R, et al. Modification of the internal ribosome entry site element impairs the growth of foot-and-mouth disease virus in porcine-derived cells. J Gen Virol. 2016;97(4):901–911. doi: 10.1099/jgv.0.000406
- Rodríguez-Pulido M, Borrego B, Sobrino F, et al. RNA structural domains in noncoding regions of the foot-and-mouth disease virus genome trigger innate immunity in porcine cells and mice. J Virol. 2011;85(13):6492–6501. doi: 10.1128/JVI.00599-11
- Borrego B, Rodríguez-Pulido M, Revilla C, et al. Synthetic RNAs mimicking structural domains in the foot-and-mouth disease virus genome elicit a broad innate immune response in porcine cells triggered by RIG-I and TLR activation. Viruses. 2015;7(7):3954–3973. doi: 10.3390/v7072807
- Dobrikov MI, Dobrikova EY, McKay ZP, et al. PKR binds enterovirus IRESs, displaces host translation factors, and impairs viral translation to enable innate antiviral signaling. mBio. 2022;13(3):e0085422. doi: 10.1128/mbio.00854-22
- Li R, Wang M, Gong P. Crystal structure of a pre-chemistry viral RNA-dependent RNA polymerase suggests participation of two basic residues in catalysis. Nucleic Acids Res. 2022;50(21):12389–12399. doi: 10.1093/nar/gkac1133
- Smola MJ, Rice GM, Busan S, et al. Selective 2′-hydroxyl acylation analyzed by primer extension and mutational profiling (SHAPE-MaP) for direct, versatile and accurate RNA structure analysis. Nat Protoc. 2015;10(11):1643–1669. doi: 10.1038/nprot.2015.103