ABSTRACT
Spermiogenesis is the longest phase of spermatogenesis, with dramatic morphological changes and a final step of spermiation, which involves protein degradation and the removal of excess cytoplasm; therefore, we hypothesized that macroautophagy/autophagy might be involved in the process. To test this hypothesis, we examined the function of ATG5, a core autophagy protein in male germ cell development. Floxed Atg5 and Stra8− iCre mice were crossed to conditionally inactivate Atg5 in male germ cells. In Atg5flox/flox; Stra8− iCre mutant mice, testicular expression of the autophagosome marker LC3A/B-II was significantly reduced, and expression of autophagy receptor SQSTM1/p62 was significantly increased, indicating a decrease in testicular autophagy activity. The fertility of mutant mice was dramatically reduced with about 70% being infertile. Sperm counts and motility were also significantly reduced compared to controls. Histological examination of the mutant testes revealed numerous, large residual bodies in the lumen of stages after their normal resorption within the seminiferous epithelium. The cauda epididymal lumen was filled with sloughed germ cells, large cytoplasmic bodies, and spermatozoa with disorganized heads and tails. Examination of cauda epididymal sperm by electron microscopy revealed misshapen sperm heads, a discontinuous accessory structure in the mid-piece and abnormal acrosome formation and loss of sperm individualization. Immunofluorescence staining of epididymal sperm showed abnormal mitochondria and acrosome distribution in the mutant mice. ATG5 was shown to induce autophagy by mediating multiple signals to maintain normal developmental processes. Our study demonstrated ATG5 is essential for male fertility and is involved in various aspects of spermiogenesis.
Abbreviations: AKAP4: a-kinase anchoring protein 4; ATG5: autophagy-related 5; ATG7: autophagy-related 7; ATG10: autophagy-related 10; ATG12: autophagy-related 12; cKO: conditional knockout; DDX4: DEAD-box helicase 4; MAP1LC3/LC3/tg8: microtubule-associated protein 1 light chain 3; PBS: phosphate-buffered saline; PIWIL2/MILI: piwi like RNA-mediated gene silencing 2; RT-PCR: reverse transcription-polymerase chain reaction; SQSTM1/p62: sequestosome 1; TBC: tubulobulbar complexes; WT: wild type.
Introduction
Autophagy is an intracellular degradation process by which dysfunctional or unnecessary proteins and damaged organelles are transported to the lysosomes for degradation to maintain metabolism and energy homeostasis in cells [Citation1,Citation2]. Three types of autophagy mechanisms have been identified in mammalian cells: macroautophagy, microautophagy and chaperone-mediated autophagy. Macroautophagy/autophagy is the most widely characterized among the three pathways and is evolutionarily conserved from yeast to mammals [Citation1,Citation2]. Autophagy is initiated from the development of an isolated membrane (or phagophore) that then sequesters cytoplasmic materials and elongates to form a double-membrane autophagosome. The outer membrane of an autophagosome subsequently fuses with the lysosome to form an autolysosome, in which the sequestered contents are degraded by lysosomal enzymes [Citation3,Citation4]. Two ubiquitin-like conjugation systems, ATG12 (autophagy related 12)–ATG5 and MAP1LC3/LC3/Atg8 (microtubule-associated protein 1 light chain 3)–phosphatidylethanolamine (PE) conjugates, are tightly associated with autophagosome formation [Citation5,Citation6]. ATG12 is activated by an ubiquitin-activating E1-like enzyme ATG7 (autophagy-related 7), transferred to E2-like enzyme ATG10 (autophagy-related 10) and finally attached to ATG5, forming an ATG12–ATG5 conjugate [Citation4,Citation7]. This conjugate further recruits ATG16L1 (autophagy-related 16 like 1) to form a multimeric complex that can enhance the formation of MAP1LC3–PE conjugates [Citation8,Citation9]. MAP1LC3 is activated by ATG7 and sequentially conjugated to PE-containing membranes. The MAP1LC3–PE conjugate mediates membrane expansion and maturation of autophagosomes [Citation10,Citation11]. In mammals, autophagy is involved in many physiological processes, such as cell growth/apoptosis, anti-aging regulation, pre-implantation development, adaptation to stress conditions, response to infection, and biogenesis of sperm flagella and acrosome [Citation12,Citation13].
Mammalian spermatogenesis is a complex, step-wise process in seminiferous tubules, during which spermatogonia undergo mitotic proliferation to generate spermatocytes that programmatically undergo meiotic divisions to form round spermatids that differentiate by elongation over an extended period of time [Citation14]. The final phase is spermiogenesis, during which the round, haploid spermatids undergo structural reorganization with dramatic morphological changes. This phase of spermatogenesis involves condensation and remodeling of nuclear chromatin and shape, formation of the acrosome that covers much of the nuclear membrane, disposal of residual cytoplasm as the residual body and assembly of the sperm flagellum [Citation15]. Finally, the mature, elongated spermatids are released as spermatozoa from the Sertoli cells into the lumen of seminiferous tubules via a process known as spermiation [Citation16]. As spermiogenesis involves the removal of excess cytoplasm and protein degradation, it is hypothesized that autophagy may play an important role in the formation of the cytoplasmic lobe and residual body during the development of spermatozoa, which is supported by recent findings. Autophagy has been shown to be required during reproductive cell development. Impaired autophagic flux in the medaka fish results in reduced cytoplasmic clearance during sperm specification, causing reduced fertility [Citation17]; in the moss Physcomitrella patens, autophagy is required for gamete differentiation. ATG5- and ATG7-mediated autophagy is essential for the differentiation and cytoplasmic reduction of the flagellated motile sperm and hence for sperm fertility [Citation18].
In birds, autophagy core components are enriched in the elongated spermatids [Citation19]. In the sea urchin, Strongylocentrotus intermedius, autophagy is involved in formation of residual bodies and removal of excessive cytoplasm by spermatids during spermatogenesis in this species [Citation20]. In the human testis, key autophagy components were found on the acrosome, and the equatorial segment of sperm heads and autophagy was increased in sperm cells from patients with a cryptorchid history [Citation21].
In mice, germ cell-specific disruption of Atg7 causes almost complete infertility with aberrant acrosome formation, which is similar to human globozoospermia, a severe fertility disorder characterized by abnormal round-headed spermatozoa [Citation13]. ATG5 is another autophagy core component. Global knockout of Atg5 in mice results in neonatal lethality [Citation22], thus preventing analysis of its functional roles post-natally. The roles of ATG5 in Sertoli cells and Leydig cells have been studied. Both studies revealed essential roles for ATG5 in these somatic cells in the testis [Citation23,Citation24]. However, the function of ATG5 in mouse germ cells is unknown. To elucidate the role of ATG5 in male germ cell development and fertility, we generated a male germ cell-specific atg5 knockout mouse line by crossing floxed Atg5 mice with Stra8-iCre transgenic mice. The conditional atg5-deficient male mice showed dramatically reduced fertility associated with reduced sperm numbers and abnormal sperm morphology, including misshapen heads, aberrant acrosome formation, enlarged residual bodies, and mitochondrial rearrangement. Most importantly, spermiation or release of sperm into the lumen was inhibited, presumably due to failure to remove intercellular bridge components, as a result of disrupted autophagy activity. The study demonstrated that ATG5 is required for normal spermiogenesis and male fertility by maintaining normal autophagy function in germ cells.
Results
ATG5 is abundant in mouse testis and is present in the cytoplasm of spermatocytes and round spermatids
ATG5 protein level was compared between mouse testis and selective somatic tissues by western blot analysis. Five commercial anti-ATG5 antibodies were used in the study, but only one antibody was successful (Cell Signaling Technology, 12994S). ATG5 protein was highly abundant in the testis. It was also detected in liver, kidney and spleen in our experiment (). Immunofluorescence staining was also conducted on testis sections using the five commercial antibodies. However, none of the antibodies worked on either paraffin sections or cryosections, even though several other antibodies worked, namely antibodies against PIWIL2/MILI (piwi like RNA-mediated gene silencing 2), DDX4 (DEAD-box helicase 4) and AKAP4 (a-kinase anchoring protein 4), which were highly abundant in germ cells (Fig.S1).
Figure 1. ATG5 is abundant in mouse testis and is present in the cytoplasm of spermatocytes and round spermatids. (A) Analysis of tissue distribution of mouse ATG5 protein by western blot. ATG5 protein was abundant in the testis. It was also detected in the liver, kidney and spleen in our experiment (upper panel). GAPDH was analyzed as a loading control (bottom panel). (B) Examination of ATG5 localization in isolated male germ cells by immunofluorescence staining. Isolated germ cells were stained with the ATG5 antibody (red). The ATG5 signal was present as cytoplasmic vesicles in spermatocytes and round spermatids
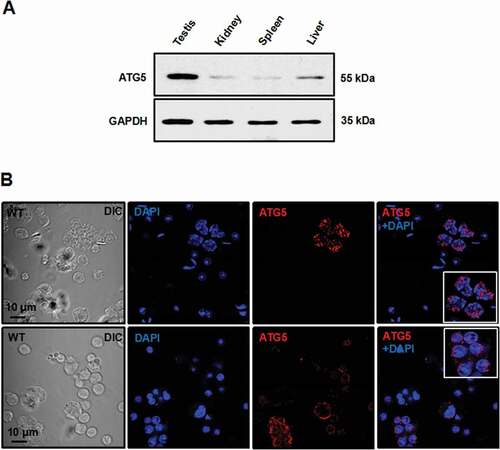
Immunofluorescence was successful for examining testicular germ cells that were isolated from adult mice, and the expression and cellular localization of ATG5 could be analyzed. The ATG5 signal was observed predominantly in cytoplasmic regions of spermatocytes and round spermatids when the antibody from Thermo Fisher Scientific was used ().
Generation of male germ cell-specific atg5 knockout mice and the disruption of autophagic flux in the conditional atg5 knockout (cKO) mice
To study the role of ATG5 during spermatogenesis in vivo, the floxed Atg5 mice were crossed with Stra8-iCre mice so that the Atg5 gene was specifically disrupted in male germ cells. Exon 3 of the Atg5 gene was targeted in this model. The heterozygous floxed atg5 and homozygous cKO mice were identified by PCR-based genotyping (Fig.S2). Disruption of the Atg5 gene was further examined by RT-PCR using testicular cDNA and primers as illustrated in . The primer pair amplified a ~ 250 bp PCR product encompassing exons 2 to 4 in the control (WT) mouse; however, this primer pair only amplified a smaller PCR product with ~150 bp in the atg5 cKO mouse (). To compare testicular Atg5 mRNA expression levels between the control and atg5 cKO mice, real-time PCR was performed using a primer pair capable of amplifying exon 2–3. Compared to that of the control mouse, the expression of Atg5 was barely detected in the conditional knockout mouse (). Testicular ATG5 protein was further examined. The protein was detected in all the four control mice; however, only a trace amount of ATG5 protein was detected in the atg5 conditional knockout mice, which should come from the Sertoli and Leydig cells in the testis (). Immunofluorescence staining of the germ cells isolated from control and the atg5 conditional knockout mice showed that the ATG5 signal was missing in the atg5 cKO testicular germ cells (Fig. S3). The results demonstrate that the atg5 conditional knockout mouse model was successfully generated.
Figure 2. Generation of Atg5 conditional knockout (cKO) mice and the decreased autophagy activity in the testis of the Atg5 cKO mice. (A) Schematic representation of the mouse Atg5 gene structure, strategy to target exon 3 and locations of primers used in the study. (B) Representative RT-PCR results using primers that amplify exons 2 to 4 of Atg5 cDNA. a DNA band with smaller size was detected in the mutant mice, indicating that exon 3 was spliced out. (C) Real-time PCR results showed that Atg5 mRNA expression level was significantly reduced in the knockout mice. (D) Representative western blot result showing testicular ATG5 was absent in the atg5 cKO mice. (E) Expression of LC3A/B and SQSTM1/p62 as examined by western blot in the control and conditional atg5 knockout mice (n = 3). The level of 14 kDa LC3A/B-II, an indicator of active autophagy, was hardly detected in the mutant mice; SQSTM1, an autophagy substrate, was increased, indicating decreased autophagy activity in the testis of atg5 cKO mice. (F) Quantification of the relative LC3A/B-I (i) and SQSTM1 (ii) protein expression. Error bars represent the standard deviation (n = 3). (*), p < 0.05
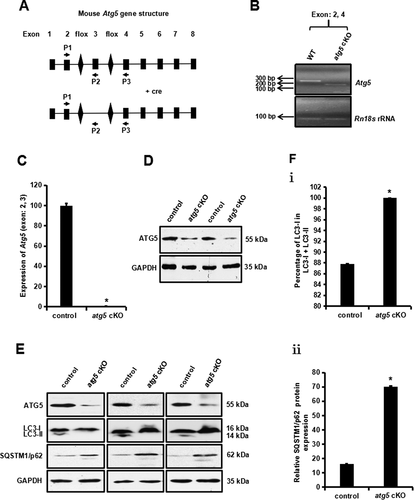
ATG5 is a core autophagy component and thus we examined the effect of Atg5 disruption on testicular expression of MAP1LC3/LC3, an autophagosome marker [Citation25], and SQSTM1/p62, autophagy receptor and substrate [Citation26]. In the control mice, both the 16-kDa LC3-I and the 14-kDa protein LC3-II were present (). However, in the atg5 cKO mice, LC3-II was hardly detected in our experiment. Given that no LC3-II was detected, the LC3-I:(LC3-I+ LC3-II) ratio was compared between control and atg5 cKO mice. The ratio was significantly increased in the atg5 cKO mice ( i). SQSTM1 was detected in both control and atg5 cKO mice (). Compared with the control mice, SQSTM1 level was significantly increased in the mutant mice ( ii).
Reduced fertility associated with reduced abnormal sperm formation and sperm motility of the germ cell-specific knockout of atg5 mice
Homozygous atg5 cKO mice did not exhibit any gross abnormalities and survived to adulthood, but the testis-to-body weight ratio of mutant mice was slightly lower than that of control mice (). Fertility of the atg5 cKO mice at different ages was examined by mating with 3- to 4-month-old wild-type females over a one-month period. To test the younger mice, six 2-month-old atg5 cKO mice and six age-matched control mice were analyzed. All six control males showed normal fertility and produced a total of 46 litters during the one month of matting. Although three of the atg5 cKO mice did not sire any pups, while the other three sired 5, 1, and 8 pups respectively, with a total 14 pups (). To test the older mice, seven 3- to 5-month-old and four 5- to 7-month-old atg5 cKO mice and eleven age-matched control mice were analyzed. All eleven control males were fertile and produced a total of 86 pups. Nine of the eleven atg5 cKO did not sire pups, while one 3- to 5-month-old atg5 cKO male sired ten pups and one 5- to 7-month-old atg5 cKO male sired one pup respectively through three months of breeding ().
Table 1. Fertility and sperm characters of control and atg5 cKO
To determine whether reduced male fertility in the atg5 cKO males was caused by altered sperm production and function, cauda epididymal sperm were examined. Sperm density was significantly reduced in the atg5 cKO mice compared to control mice, as examined by light microscopy ( and S4). Compared with sperm in the control mice, morphologically abnormal sperm were mostly present in the atg5 cKO mice ( and S4). Under high magnification, about 50% abnormalities in the sperm head were observed. Sperm from the mutant mice had round, swollen or bent heads, and some sperm had two heads. 33.29% sperm aggregated into bundles, and 23.12% sperm had unsheathed flagella at the principal piece ( ii-viii, and 3C). To better illustrate the abnormal sperm morphology, scanning electron microscopy (SEM) was conducted. Consistently, sperm from the control mice displayed normally developed heads and flagella ( i). However, sperm from the atg5 cKO mice showed morphological abnormalities in head and tails as observed under light microscopy. Moreover, some mutant sperm showed a short flagellum with a bulb at the tip of the tail, or a large vesicle at the mid-piece ( ii-viii).
Figure 3. Abnormal sperm morphologies in the atg5 cKO mice. (A) Representative epididymal sperm of control (i) and atg5 cKO mice (ii–viii) examined by DIC microscopy. Sperm in the control mice showed normal morphology (i). Multiple abnormalities were observed in the atg5 cKO mice, including distorted (arrow) and round heads (arrowhead) (ii), abnormal flagella (ii–iv), double heads (v), and sperm bundle (vi–viii). (B) Representative epididymal sperm of control (i) and atg5 cKO mice (ii–Viii) examined by scanning electron microscopy. As observed under DIC microscope, well-developed head (H) and long and smooth flagella (F) were present in the control mice (i), the atg5 cKO sperm had abnormally developed sperm heads (arrowheads in ii, iii, iv, vi, vii), discontinuous accessory structure in the middle piece were also observed in some sperm (arrowheads, iii, iv, vii, viii); some sperm had distorted structure in the middle piece (v), short tail (vi), sperm bundle was also seen (viii). (C) Quantification of the percentage of different types of abnormal sperm in the control and atg5 cKO mice
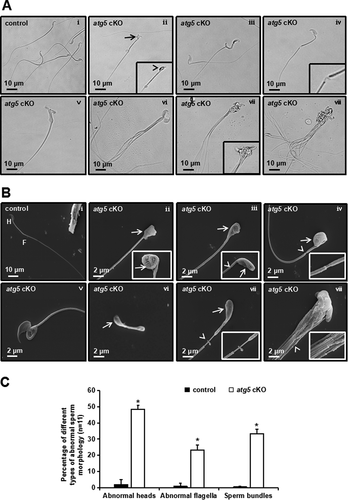
In addition, the number of epididymal sperm was reduced by about 60% in cKO mice as compared with control mice (). More than 80% of the sperm from control mice were motile, while only a small percentage of the mutant sperm were motile ( and Movie S1). The few sperm that were motile showed significantly reduced motility ( and Movie S2).
Disruption of Atg5 in male germ cells leads to spermatogenesis defects.
Reduced sperm number and aberrant sperm morphology in atg5 cKO mice suggest a defect in spermatogenesis. Therefore, testicular histology of control and atg5 cKO mice was examined. Periodic acid-schiff (PAS) staining of the seminiferous epithelium indicated that in all stages of the atg5 cKO testis, spermatogonia, spermatocytes and round spermatids appeared normal compared to control mice (, upper panel). However, abnormalities began to appear in steps 10–14 elongating spermatids of the atg5 cKO testes. Abnormal nuclear shapes began to appear with mixing of step 9 and 12 spermatids in stage XII (, lower panel). Residual bodies were reabsorbed by Sertoli cells rapidly in stage IX of control testes ( iii), but in the atg5 cKO, large residual bodies were sloughed into the lumen and found even in stages XI–XII ( viii, and x). In the atg5 cKO testes, there was also evidence of spermiation failure to a limited extent, as step 16 spermatids were still present in stages IX–XII (). Other abnormal changes included the fusion of some elongating spermatids into giant cells and sloughing of cytoplasm or cytoplasm with attached immature elongating spermatids into the lumen. Evidence of sloughing from the seminiferous epithelium in the atg5 cKO testes was seen in their respective cauda epididymides lumen, where round germ cells and cytoplasm resembling residual bodies were present ().
Figure 4. Histology of testis and epididymis from the control and atg5 cKO mice. (A) Testis histology from control (WT) and atg5 cKO mice showing sections of the seminiferous epithelium. Images correspond to the respective stages of spermatogenesis from control testis (top row) and the atg5 cKO testis (bottom row). The numbers represent the various steps of round and elongated spermatids. M1 labels the pachytene spermatocytes in meiotic division in stage XII. In all stages of the atg5 cKO testis, spermatogonia, spermatocytes and round spermatids appear normal compared to controls. However, the cytoplasm of elongating spermatids begins to appear abnormal in step 14–16, with the sloughing of cells into the lumen along with round bodies of cytoplasm and the fusion of some elongating cells into giant bodies. Scale bar = 20 μm for all photos. (I) WT stage II–III showing bundles of elongating step 14 and round step 2–3 spermatids. (ii) WT stage V–VI, with bundles of step 15 spermatids resting above the round spermatids. (iii) WT stage IX. Step 9 spermatid nuclei have begun to show the angling of the nucleus at the start of elongation. Step 16 spermatids have matured and released into the lumen as sperm. Residual bodies have been reabsorbed rapidly by the Sertoli cells in this stage and are rarely seen in stages IX–X. (iv) WT stage XI, with an abundance of step 11 elongating spermatids lining the lumen. (V) WT stage XII. Bundles of step 12 spermatids sit above the dividing pachytene spermatocytes that exhibit meiotic division (M1). (vi) atg5 cKO stage II–III. Step 2–3 round spermatids appear normal; however, the cytoplasm of elongating step 14 spermatids appear abnormal, with the sloughing of cells into the lumen along with round bodies of cytoplasm (Cy). The elongating spermatid bundles have fewer numbers of cells. (vii) atg5 cKO stage V–VI. The elongating step 15 spermatids have abnormal heads and there is fusion of some elongating cells into giant bodies. (viii) atg5 cKO stage IX, with step 16 spermatids show retention in the epithelium, rather than being released by spermiation. Abnormally large bodies of fused residual bodies (Rb) are seen sloughing into the lumen and subsequently appear in the epididymis. In the KO testis, the fused residual bodies were often found in stages XI–XII, along with the retained step 16 spermatids. (ix) atg5 cKO stage XI, showing residual bodies (Rb) at the lumen, but also with attached step 16 spermatids being phagocytosed by the epithelium and near the basement membrane. (X) atg5 cKO stage XI, with large residual bodies (Rb) near the lumen along with step 16 spermatids being retained. (xi) atg5 cKO stage XII. Abnormal step 12 spermatids are lacking bundle formation, indicating that there has been the loss of elongating spermatids due to sloughing and phagocytosis. Step 16 spermatid heads are seen near the basement membrane, indicating phagocytosis. (xii) atg5 cKO stage XII. Meiotic division (M1) of the pachytene spermatocytes appears to be normal, but the elongating spermatids show abnormalities and fewer numbers near the lumen. Step 9 spermatids are also abnormally present near the lumen. (B) Significant number of residual bodies were present in the epididymis and seminiferous tubule lumen of the atg5 cKO mice. (i) Control cauda epididymis showing the epithelium (Ep) lining the lumen that is filled with normal sperm aligned with their heads (Hd) and tails (T). (ii) atg5 cKO cauda epididymis showing a lumen filled with numerous, large cytoplasmic bodies (Cy), sloughed germ cells (Gc) and spermatozoa with heads (Hd) and tails (T) that are less aligned than those in the control. Ep, epithelium. (iii) TEM of lumen area of seminiferous tubules of control mice. Normally developing spermatids in a control mouse with well-developed heads (arrows). (iv) TEM of lumen area of seminiferous tubules of atg5 cKO mice. Residual bodies (arrows) were present, and abnormally formed chromatin (arrowheads) was throughout. The dashed arrows may represent lipid droplets and RNAs (leftover ribosomes)
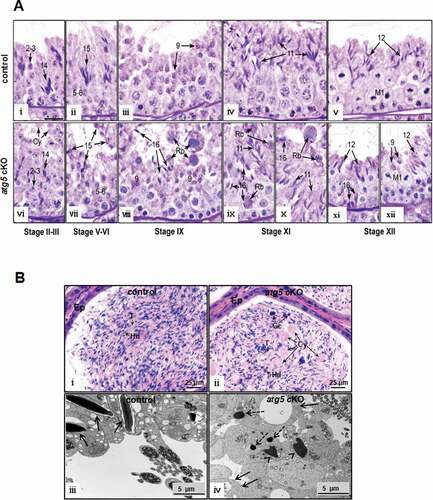
Disruption of Atg5 in male germ cells causes abnormal acrosome formation.
A significant number of rounded head sperm were observed in atg5 cKO mice; therefore, the acrosome was examined with peanut-lectin. A well-formed acrosome was developed over the anterior part of the sperm head in control mice. However, in the mutant sperm, peanut-lectin aggregated around the nucleus and showed abnormal acrosome localization (). Acrosome formation was also examined in the developing spermatids by TEM. Well-developed acrosomes were observed in the control testis ( i and ii). However, abnormally shaped acrosomes that followed abnormal shapes of the forming nuclei were discovered in the spermatids of atg5 cKO mice ( iii and iv).
Figure 5. Abnormal acrosome biogenesis in the atg5 cKO mice. (A) Immunofluorescence staining was performed on cauda epididymal sperm from control (left panel) and atg5 cKO mice (right three panels). Peanut-lectin was used as a marker for acrosome. The images show the abnormal acrosome formation in the atg5 cKO mice. (B) Examination of spermatid acrosome in the testis seminiferous tubules of control (i, ii) and atg5 cKO mice (iii, iv) by electronic microscopy. In the control mouse, normal acrosome development can be seen in the round and elongating spermatids (arrows in i and ii). However, in the atg5 cKO mice, abnormal acrosome development was present (dashed arrows in iii and iv)
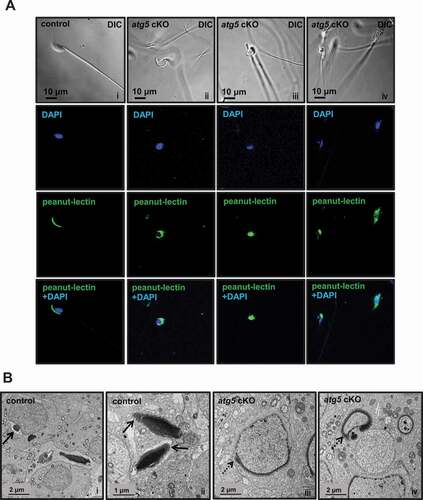
Abnormal mitochondria distribution in sperm of the atg5 cKO mice.
Due to a defect in the middle piece of the flagella and significantly reduced motility, we further investigated the effect of atg5 knockout on sperm mitochondria. In the control mice, staining of sperm mitochondria showed normal midpiece distribution ( i). However, the pattern was changed in the mutant mice, with significant staining present at the head region of some cells, suggesting that the middle pieces and tails were coiling around the heads, possibly due to the failure to form a normal cytoplasmic lobe and residual body in stage VII–VIII (see ). Discontinuous and bundle forms of mitochondrial signals were seen in the atg5 cKO mouse ( ii-iv). TEM of epididymal sperm from control mice showed well-aligned mitochondria surrounding the axoneme at the midpiece ( i). In atg5 cKO mice, cross-sections of two or more axonemes could be seen in one cell, some of which were surrounded with disconnected mitochondria ( ii-iv). The results are consistent with the DIC microscope and SEM results.
Figure 6. Abnormal sperm mitochondrial sheath formation in the atg5 cKO mice. (A) Representative images of mitochondria staining of cauda epididymal sperm from control and atg5 cKO mice. Normal sperm mitochondria staining (i) can be seen in the control mouse. Discontinuous (arrowhead, ii) and bundle (arrows, iii, iv) mitochondria signal was seen in the atg5 cKO mouse. (B) Representative TEM images of epididymal sperm from control and atg5 cKO mice. a control mouse showed the separate axoneme formed around with connected mitochondria (arrowheads and the insert, i). In the atg5 cKO mouse, several axonemes were included in one member (arrows, ii, iii) and some of them were surrounded with disconnected mitochondria (arrowheads, iii, iv)
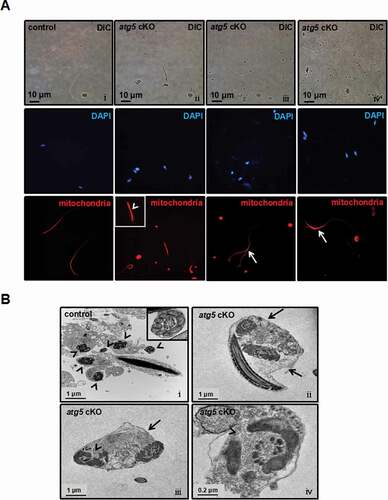
Defective sperm nuclear structure and disrupted individualization in the atg5 cKO mice as revealed by TEM study
In order to investigate the structural base of reduced sperm number and motility, TEM was conducted in epididymal sperm and testis. In the control mice, well-developed epididymal sperm were displayed, and chromatin was normally formed ( i). However, sperm in the atg5 cKO mice had several abnormalities, including abnormal development of the elongating nuclei ( ii-iv), and two or more axonemes wrapped by one cell membrane were frequently observed ( iv-vi). In control seminiferous tubules, normal spermatid development was observed, with each elongating spermatid being wrapped by its own cell membrane and thus displaying individualization ( i). However, in the atg5 knockout mice, multiple elongating spermatids were found wrapped by a single cell membrane, indicating a failure of maintaining the intercellular bridges between individual spermatids ( ii).
Figure 7. Defective sperm chromatin structure and disrupted individualization in the atg5 cKO mice as revealed by TEM study. (A) Epididymal sperm. (i) a representative image showing a large number of sperm collected from cauda epididymis. Normal chromatin structure was present (insert) in a control mouse. (ii, iii, iv) The arrows point to the abnormally formed chromatin of epididymal sperm from the atg5 cKO mouse; (iv, v, vi) multiple sperm were wrapped in one cell membrane (arrowheads). (B) Seminiferous tubules. (i) a representative image showing normally separated elongating spermatids in the seminiferous tubules of a control mouse. The arrow points to the cell membrane; (ii) multiple elongating spermatids in the seminiferous tubule were wrapped in one cell membrane in the atg5 cKO mouse, indicating failure of individualization. The arrowheads point to the elongating spermatids sharing the same cell membrane
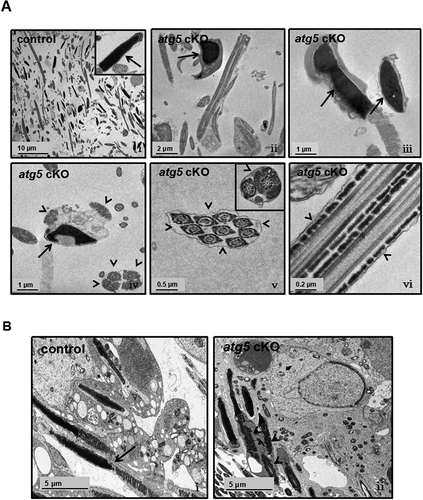
Discussion
Autophagy, a lysosome-mediated pathway that is regulated by multiple proteins, is found in spermatogenesis [Citation27]. ATG5 is one of the core autophagy proteins that, in the mouse testis, can modulate Sertoli cell and Leydig cell functions and regulate spermatogenesis [Citation23,Citation24]. In this study, we generated a male germ cell-specific atg5 knockout mouse model and studied its direct role in germ cell development. Compared with other selective somatic tissues, ATG5 is highly abundant in the testis, indicating a specific role of the protein in male germ cell development. However, immunofluorescence staining on testis sections never worked using all the five commercial antibodies. Using one of the five anti-ATG5 antibodies, ATG5 was found to be abundant in the cytoplasmic vesicles of spermatocytes and round spermatids. Western blot analysis, real-time PCR and RT-PCR using multiple primer sets confirmed deletion of the floxed exon, and dramatically reduced ATG5 protein in the testis of the atg5 cKO mice, indicating that the model was successful. ATG5 has been reported to be expressed in Leydig cells and Sertoli cells in the testis [Citation23,Citation24]. In our western blot analysis, only a trace amount of ATG5 protein was detected in this germ cell-specific knockout mice, indicating that ATG5 was much more abundant in germ cells instead of somatic cells. The model was also supported by the significantly reduced autophagy product and increased autophagic substrate [Citation6] in the conditional knockout mice, and the reduced autophagy activity was consistent with previous findings in other tissues of atg5-deficient mice [Citation28,Citation29].
The phenotypic analysis of the atg5 cKO mice suggested that ATG5 had multiple roles in sperm formation and function, including proper nuclear shape formation, acrosome formation, removal of residual bodies, mitochondria rearrangement, and sperm individualization or separation at the intercellular bridges, all of which are unique biological processes in spermiogenesis. No major defects in mitosis and meiosis were discovered. It has been shown that autophagy core components are more enriched in the latter steps of germ cell development [Citation19], indicating that autophagy might play a more important role in spermiogenesis and in particular the elongating spermatids. The present phenotype was in line with the distribution pattern of these core proteins.
It is not surprising that acrosome biogenesis was affected in the conditional atg5 knockout mice, as this phenotype was also reported in germ cell-specific atg7 knockout mice [Citation13]. The acrosome is a unique organelle formed during spermiogenesis [Citation30]. Golgi-derived vesicles contribute to the formation of acrosome [Citation31], which contains numerous enzymes that in somatic cells are associated with lysosomes. Thus, the acrosome is also regarded as a modified lysosome or a novel lysosome-related organelle (LRO) [Citation32,Citation33]. More recently, it was hypothesized that the acrosome originates from an autolysosome rather than a mere lysosome, and this was supported by the findings from the germ cell-specific atg7 knockout mice [Citation13]. Therefore, like ATG7, ATG5 is likely involved in the same pathway as ATG7 in the formation of acrosome, modulating autolysosome-derived acrosome biogenesis.
Failure of spermiation, although not as extensive as in certain toxicant exposures [Citation16], was a significant finding in the atg5 cKO mice. Spermiation is the process of germ cell individualization and disengagement from the Sertoli cell for release into the seminiferous tubule lumen [Citation16]. The process involves numerous steps, including the movement of excess cytoplasm from around the flagellum to form the cytoplasmic lobe and residual body, as well as formation and resorption of the specialized junctions between Sertoli cells and elongating spermatids. Thus, loss of ATG5 appears to have disrupted one or more of the steps in this delicate process of disengagement, which likely contributes to the abnormal formation of large residual bodies that slough into the lumen. Failure of spermiation was not reported previously in the germ cell-specific atg7 knockout mice, nor alterations in mitochondrial alignment [Citation13].
The sloughing of a large number of residual bodies into the lumen in the atg5 cKO mice is likely related to the failure of spermiation, due to the disruption of potentially overlapping anatomical steps, such as the formation and resorption of the tubulobulbar complexes (TBC) that interact between the Sertoli cells and cytoplasm of the ventral curvatures of spermatid heads [Citation34,Citation35]. TBCs are specialized, finger-like structures between Sertoli cells and elongating spermatids that form in the final steps of spermiation [Citation17,Citation36] and have been suggested to be a modified form of the endocytic machinery [Citation35,Citation37]. These tubular complexes are believed to be involved in reduction in the volume of the spermatid cytoplasm during spermiation, but more recently have been shown to be involved with the internalization of intercellular junctions as part of sperm release [Citation38,Citation39]. Given that disruption of Atg5 in Sertoli cells caused a spermiation defect [Citation23], and loss of AMPH (amphiphysin), a specific TBC protein, also induces failure of spermiation [Citation40], it is likely that the germ cell-specific loss of Atg5 disrupts the germ cell component of the TBC interaction with Sertoli cells. These studies demonstrate that ATG5 might be a major germ cell factor associated with Sertoli cell’s formation of TBCs and indirectly ensuring spermiation of mature sperm and phagocytosis of residual bodies.
Disruption of spermatid individualization (separation during spermiation) was a distinguishing feature observed in the germ cell-specific atg5 knockout mice. During spermatogenesis, spermatogonia undergo mitosis with incomplete cytokinesis to produce interconnected chains of spermatocytes that synchronously transition into meiosis [Citation41]. Cytokinesis during meiosis also is incomplete, such that cytoplasmic channels remain between sister spermatids after each division [Citation42]. This allows for the sister spermatids to share cytoplasm, which synchronizes their development and protects them from the genetic effects of haploid [Citation39]. After terminal differentiation, the cytoplasmic contents are removed and the cytoplasmic bridges connecting sister spermatids are dissolved [Citation16]. This last process is dependent on the actin cytoskeleton and is essential for proper sperm function [Citation43]. Given that multinucleated cells were not found among the atg5 knockout spermatocytes, and only in the elongating spermatids, ATG5 does not seem to have a role in cytokinesis during meiosis, which differs from the function of TEX14 (testis-expressed gene 14), a protein localized in the intercellular bridges [Citation44]. Instead, ATG5 function is focused specifically in the elongation phase of spermiogenesis.
Individualization takes place once sperm heads and tails have completed elongation. In Drosophila, this requires three successive steps: 1) recruitment of a specialized actin-based complex, the individualization complex [Citation45], 2) migration of this individualization complex associated with membrane remodeling, and 3) elimination of excess material [Citation46,Citation47]. Genetic studies have identified at least 70 genes that are involved in individualization, and several molecular pathways contribute to the process, including actin and microtubule dynamics, plasma membrane reorganization, and apoptotic elimination of the cytoplasmic contents [Citation48]. Many ubiquitin-proteasome pathway components have also been identified [Citation49], and new genetic factors were uncovered recently [Citation50,Citation51].
Sperm individualization failure has also been reported in other mice models. Targeted inactivation of DCUN1D1 (defective in cullin neddylation 1 domain containing 1 [S. cerevisiae]), a component of the E3 for neddylation in mice results in a similar phenotype as described here [Citation52]. This suggests that protein neddylation/degradation is also required for mammalian sperm individualization, and findings from our model further support that the normal autophagy pathway in male germ cells is essential for this process. It is important to point out that autophagy and ubiquitin-proteasome pathways are tightly interconnected [Citation53]. Thus, disruption of Atg5 in germ cells might also affect the ubiquitin-proteasome pathway, secondary to failure of autophagy and damage to related protein degradation processes.
Recent studies suggest that autophagy is one of the main players in the regulation of ciliogenesis [Citation54,Citation55]. However, even though sperm number was reduced in the conditional atg5 knockout mice, core axoneme “9 + 2” structure, fibrous sheath, and outer dense fiber were not affected, indicating that ATG5 was not involved in assembling core sperm structure. The disrupted mitochondria rearrangement might be a secondary defect of unknown origin due to the loss of ATG5 in germ cells, which could explain the observed reduction in sperm motility.
About 30% of atg5 cKO mice were still fertile, suggesting some spermatozoa were still functional. The male subfertility might be explained by at least two possibilities. First, ATG5-independent alternative autophagy may occur during spermatogenesis in the atg5 cKO mice. This ATG5-independent autophagy was originally detected in etoposide-treated atg5-/- mouse embryonic fibroblasts [Citation56] and then found to regulate mitochondrial clearance during erythrocyte differentiation and in the transformation of differentiated cells to induced pluripotent stem cells [Citation57,Citation58]. Second, the autophagosome-like structures generated from endoplasmic reticulum were detected in atg5 knockout mouse embryonic fibroblasts, suggesting that the conventional autophagy still occurs slowly [Citation59]. Therefore, the ATG5-independent autophagy or the residual autophagic activity may enable some germ cells to complete spermatogenesis and form functional spermatozoa. Impaired fertility seems to be age-dependent. In two-to-three months old mice, fertility was affected in half of the mutant mice (three of six) examined. However, in older mice, fertility was affected in most mutant mice (nine of eleven). It has been reported that during aging, cellular factors suggested as the cause of aging have been reported to be associated with progressively compromised autophagy [Citation60]. It is believed that the autophagy process is affected more in the older mice than that in the younger mice in the absence of ATG5.
atg5 null mice are neonatal lethal [Citation22]. Restoration of ATG5 in the brain neuron cells is sufficient to rescue atg5 null mice from neonatal lethality [Citation61,Citation62]. The rescued atg5 null mice develop multiple organ abnormalities, including reduced pituitary gland hormones and hypogonadism. In the global atg5 null mice, ATG5 protein was missing in all cells, including the germ cells. Even though ATG5 protein was recovered in the neuron cells when rescued, the protein was still absent in other cells. These phenotypes were not expected to be present in the germ cell-specific atg5 knockout mice, as ATG5 was only absent in male germ cells and its expression was unaffected in other cells.
In conclusion, we studied the role of ATG5 in mouse germ cells and discovered that germ cell-specific disruption of Atg5 impaired autophagic flux, leading to defects in acrosome biogenesis, mitochondrial rearrangement, retention of excess cytoplasm and residual bodies and failure of individualization. All these defects caused impaired sperm formation and function and therefore gave rise to reduced fertility.
Materials and methods
Ethics statement
All mice were maintained in a pathogen-free stage, and the animal research was approved by Wayne State University Institutional Animal Care and Use Program Advisory Committee (Protocol number: IACUC-18-02-0534) in accordance with Federal and local regulations regarding the use of non-primate vertebrates in scientific research.
Generation of atg5 conditional knockout mice
Atg5flox/flox mice (exons 3 were floxed) were kindly provided by Noboru Mizushima [Citation63], the University of Tokyo, and Stra8-iCre mice were purchased from the Jackson Laboratory (008208). Cre recombinase is only active in male germ cells [Citation64]. 3-4-month-old Stra8-iCre males were mated with same-age Atg5flox/flox females to obtain Stra8-iCre; Atg5flox/+ mice. The Stra8-iCre; Atg5flox/+ males were backcrossed with Atg5flox/flox females again, and the Stra8-iCre; Atg5flox/flox were considered to be the homozygous cKO mice, and Stra8-iCre; Atg5flox/+ mice were used as the controls. All mice were genotyped using the following primers to differentiate heterozygotes and homozygotes: Stra8-iCre forward: 5ʹ-GTGCAAGCTGAACAACAGGA-3ʹ; Stra8-iCre reverse: 5ʹ-AGGGACACAGCATTGGAGTC-3ʹ; Atg5 WT forward: 5ʹ-GAATATGAAGGCACACCCCTGAAATG-3ʹ; Atg5 WT reverse: 5ʹ-GTACTGCATAATGGTTTAACTCTTGC-3ʹ; Atg5 flox forward: 5ʹ-GTACTGCATAATGGTTTAACTCTTGC-3ʹ; Atg5 flox reverse: 5ʹ-ACAACGTCGAGCACAGCTGCGCAAGG-3ʹ.
RT-PCR and real-time PCR
Total testicular RNA was isolated from the control and atg5 cKO mice with TRIzol (Invitrogen, 15596026) and reversely transcribed using RevertAid first strand cDNA synthesis kit (Thermo Fisher Scientific, k1622). The cDNAs were used for RT-PCR or real-time PCR. The forward primer (5ʹ-CATCCACTGGAAGAATGACAG-3ʹ) for RT-PCR was located in exon 2 of Atg5, and the reverse primer (5ʹ- TGATGCAAGAAGATCAAATAG-3ʹ) was located in exon 4. Real-time PCRs were performed using 2× SYBR Green master mix (Bio-Rad, 1708880). The same forward primer in RT-PCR was also used for real-time PCR and the reverse primer (5ʹ- GTCAAATAGCTGACTCTTGGC-3ʹ) was located in the exon 3 of Atg5. Rn18 s rRNA level was used to normalize expression level of target genes [Citation65].
Western blot analysis
3–4 month-old mice tissue extracts were obtained after lysis with buffer containing 50 mM Tris-HCl (Sigma-Aldrich, 10812846001), pH 8.0, 170 mM NaCl (Sigma-Aldrich, s7653), 1% NP40 (Sigma-Aldrich, 127087–87-0), 5 mM EDTA (Sigma-Aldrich, 324504), 1 mM DTT (Thermo Fisher Scientific, r0862) and protease inhibitors (Roche diagnostics GmbH, 11836170001). Protein concentration for lysates was determined using Bio-Rad DCTM protein assay kit (Bio-Rad, 5000121). Loads were adjusted, and proteins were resolved by SDS-PAGE and analyzed by western blot. The following antibodies were used: anti-LC3A/B (1:1000; Cell Signaling Technology, 4108); SQSTM1/p62 (1:1000; Cell Signaling Technology, 5114); ACTB/β-actin (1:2000; Cell Signaling Technology, 4967s). Five ATG5 antibodies were used in this study (1:1000, Thermo Fisher Scientific, pa5-35201; 1:1000, Proteintech, 60061-1-lg; 1:500, Santa Cruz Biotechnology, sc-515347; 1:500, Santa Cruz Biotechnology, sc-133158; 1:1000, Cell Signaling Technology, 12994 s;), but only the one from Cell Signaling Technology worked in our experiments. Images were analyzed using ImageJ (National Institutes of Health, Bethesda, MD).
Spermatozoa counting
Sperm were collected from the cauda epididymides in warm phosphate-buffered saline (PBS) solution (Thermo Fisher Scientific, 10010023) and fixed for 10 min at room temperature with 2% formaldehyde (Sigma-Aldrich, 252549). Counts were made using a hemocytometer chamber (Daigger Scientific, ef16034 f) under a light microscope (Tokyo, Japan), and sperm number was calculated by standard methods.
Spermatozoa motility assay
Sperm were collected after swimming out from the cauda epididymides in warm PBS. Sperm motility was observed using an inverted microscope (Tokyo, Japan) equipped with 10× objective. Movies were recorded at 15 frames/s with a SANYO (Osaka, Japan) color charge-coupled device, high-resolution camera (vcc-3972) and Pinnacle Studio HD (version 14.0) software. For each sperm sample, 10 fields were analyzed. Individual spermatozoa were tracked using ImageJ and the plug-in MTrackJ. Sperm motility was calculated as curvilinear velocity (VCL), which is equivalent to the curvilinear distance (DCL) traveled by each individual spermatozoon in 1 s (VCL = DCL/t).
Tissue histology
Testes and epididymides of adult mice were fixed in 4% formaldehyde and PBS solution, paraffin-embedded, and sectioned for histology into 5 μm. Sections were stained with hematoxylin and eosin (HE, Abcam, ab245880) and periodic acid-schiff (PAS, Thermo Fisher Scientific, 88017). The sections were examined using a BX51 Olympus microscope (New York, USA) and photographed with a ProgRes C14 camera (Jenoptik Laser, Germany).
Isolation of spermatogenic cells and epididymal sperm and immunofluorescence analysis
Spermatogenic cells were prepared as previously described [Citation66]. Briefly, testes from an adult wild-type mouse were decapsulated and placed in 5 mL DMEM (Sigma-Aldrich, d5030) containing 0.5 mg/mL collagenase IV (Sigma-Aldrich, c1889) and 1.0 mg/mL DNase I (Sigma-Aldrich, dn25), and was incubated for 30 min at 32°C to dissociate testicular cells, then centrifuged for 5 min at 160 × g. Dispersed, mixed testicular cells were fixed by 15 min incubation in 4% paraformaldehyde/PBS (containing 4% sucrose (Sigma-Aldrich, s7903)) at room temperature, then washed three times with PBS. Prior to plating, cells were resuspended in PBS and 100 µl of cell suspension was spread on SuperFrost/Plus microscope slides (Thermo Fisher Scientific, 22–037-246) and allowed to air-dry. For immunofluorescence on sperm samples, sperm were obtained from cauda epididymis of control and conditional atg5 knockout mice, centrifuged at 3,000 × g, washed and resuspended in PBS, and layered onto polylysine-coated slides (Sigma-Aldrich, p0425-72ea). The preparations were fixed with 4% paraformaldehyde, permeabilized with 0.1% Triton X-100 (Sigma-Aldrich, 9002–93-1), and then washed with PBS three times. The slides were incubated with the indicated primary antibody at 4°C overnight: anti-ATG5 (1:100, Thermo Fisher Scientific, pa5-35201), Mito TrackerR Red CMXRos Mitochondria (100 nM, Molecular Probes Life Technologies, m7512), Peanut-lectin (2 µg/ml, Invitrogen, l21409). Slides were washed with PBS and incubated with Cy3-conjugated anti-rabbit IgG secondary antibody (1:5000; Jackson ImmunoResearch Laboratories, 111–165-003) for 1 h at room temperature. The slides were then washed with PBS three times, mounted using VectaMount with DAPI (Vector Laboratories, h-1800), and sealed with a coverslip. Images were captured by confocal microscopy at the Wayne State University Microscopy Core facility.
Immunofluorescence was also conducted on paraffin sections and cryosections double-stained using the above five anti-ATG5 antibodies and anti-PIWIL2/MILI [Citation67], anti-DDX4 (1:200, Abcam, ab13840) [Citation68], and anti-AKAP4 [Citation69] antibodies.
Transmission electron microscopy
Mouse testes and epididymal sperm were fixed with 3% glutaraldehyde (Sigma-Aldrich, g5882) and 1% paraformaldehyde in 0.1 M sodium cacodylate (Sigma-Aldrich, 70114) at 4°C and a pH of 7.4 overnight and processed for electron microscopy. Images were taken with a Jeol JEM-1230 transmission electron microscope.
Statistical analysis
Graphs were created using Microsoft Excel. Statistical analyses were performed using Student’s t-test. P > 0.05 was considered as not significant and by convention *P < 0.05.
Supplemental Material
Download Zip (51 MB)Acknowledgments
We thank Dr. Scott C. Henderson for his assistance and the staff of the Microscopy Core Facility of Virginia Commonwealth University. We also thank Dr. Ramesh S Pillai at European Molecular Biology Laboratory for providing the anti-PIWIL2/MILI antibody, and Dr. George L Gerton at the University of Pennsylvania for providing the anti-AKAP4 antibody.
Disclosure statement
There is no conflict of interest that could be perceived as prejudicing the impartiality of the research reported.
Supplementary material
Supplemental data for this article can be accessed here.
Additional information
Funding
References
- Feng Y, He D, Yao Z, et al. The machinery of macroautophagy. Cell Res. 2014;24:24.
- Yoshii SR, Mizushima N. Monitoring and measuring autophagy. Int J Mol Sci. 2017;18:1865.
- Mizushima N, Levine B. Autophagy in mammalian development and differentiation. Nat Cell Biol. 2010;12:823.
- Noda NN, Inagaki F. Mechanisms of autophagy. Annu Rev Biophys. 2015;44:101–122.
- Nakatogawa H. Two ubiquitin-like conjugation systems that mediate membrane formation during autophagy. Essays Biochem. 2013;55:39–50.
- Katsuragi Y, Ichimura Y, Komatsu M. p62/SQSTM 1 functions as a signaling hub and an autophagy adaptor. Fed European Biochem Soc J. 2015;282:4672–4678.
- Mizushima N, Noda T, Yoshimori T, et al. a protein conjugation system essential for autophagy. Nature. 1998;395:395.
- Hanada T, Noda NN, Satomi Y, et al. The Atg12-Atg5 conjugate has a novel E3-like activity for protein lipidation in autophagy. J Biol Chem. 2007;282:37298–37302.
- Mizushima N, Noda T, Ohsumi Y. Apg16p is required for the function of the Apg12p–Apg5p conjugate in the yeast autophagy pathway. European Mol Biol Organ J. 1999;18:3888–3896.
- Xie Z, Nair U, Klionsky DJ. Atg8 controls phagophore expansion during autophagosome formation. Mol Biol Cell. 2008;19:3290–3298.
- Y-s S, Waguri S, Iwata J-I, et al. The Atg8 conjugation system is indispensable for proper development of autophagic isolation membranes in mice. Mol Biol Cell. 2008;19:4762–4775.
- Wirawan E, Berghe TV, Lippens S, et al. Autophagy: for better or for worse. Cell Res. 2012;22:43.
- Wang H, Wan H, Li X, et al. Atg7 is required for acrosome biogenesis during spermatogenesis in mice. Cell Res. 2014;24:852.
- Griswold MD. Spermatogenesis: the commitment to meiosis. Physiol Rev. 2015;96:1–17.
- O’Donnell L. Mechanisms of spermiogenesis and spermiation and how they are disturbed. Spermatogenesis. 2014;4:e979623.
- O’Donnell L, Nicholls PK, O’Bryan MK, et al. Spermiation: the process of sperm release. Spermatogenesis. 2011;1:14–35.
- Herpin a, Englberger E, Zehner M, et al. Defective autophagy through epg5 mutation results in failure to reduce germ plasm and mitochondria. Fed American Soc Exp Biol J. 2015;29:4145–4161.
- Sanchez-Vera V, Kenchappa CS, Landberg K, et al. Autophagy is required for gamete differentiation in the moss physcomitrella patens. Autophagy. 2017;13:1939–1951.
- Haseeb a, Bai X, Vistro W, et al. Characterization of in vivo autophagy during avian spermatogenesis. Poult Sci. 2019;98:5089–5099.
- Kalachev AV, Yurchenko OV, Kiselev KV. Macroautophagy is involved in residual bodies formation during spermatogenesis in sea urchins, strongylocentrotus intermedius. Tissue Cell. 2019;56:79–82.
- Yefimova MG, Buschiazzo a, Burel a, et al. Autophagy is increased in cryptorchid testis resulting in abnormal spermatozoa. Asian J Androl. 2019;21:570.
- Kuma a, Hatano M, Matsui M, et al. The role of autophagy during the early neonatal starvation period. Nature. 2004;432:1032.
- Liu C, Wang H, Shang Y, et al. Autophagy is required for ectoplasmic specialization assembly in sertoli cells. Autophagy. 2016;12:814–832.
- Gao F, Li G, Liu C, et al. Autophagy regulates testosterone synthesis by facilitating cholesterol uptake in Leydig cells. J Cell Biol. 2018;217:2103–2119.
- Kabeya Y, Mizushima N, Yamamoto a, et al. LC3, GABARAP and GATE16 localize to autophagosomal membrane depending on form-II formation. J Cell Sci. 2004;117:2805–2812.
- Bjørkøy G, Lamark T, Johansen T. p62/SQSTM1: a missing link between protein aggregates and the autophagy machinery. Autophagy. 2006;2:138–139.
- Yin J, Ni B, Z-q T, et al. Regulatory effects of autophagy on spermatogenesis. Biol Reprod. 2017;96:525–530.
- Kurashige T, Nakajima Y, Shimamura M, et al. Basal autophagy deficiency causes thyroid follicular epithelial cell death in mice. Endocrinology. 2019;160:2085–2092.
- Nakai a, Yamaguchi O, Takeda T, et al. The role of autophagy in cardiomyocytes in the basal state and in response to hemodynamic stress. Nat Med. 2007;13:619.
- Khawar MB, Gao H, Li W. Mechanism of acrosome biogenesis in mammals. Front Cell Dev Biol. 2019;7:195.
- Berruti G, Paiardi C. Acrosome biogenesis: revisiting old questions to yield new insights. Spermatogenesis. 2011;1:95–98.
- Hartree E. The acrosome—lysosome relationship. Reproduction. 1975;44:125–126.
- Kang-Decker N, Mantchev GT, Juneja SC, et al. Lack of acrosome formation in Hrb-deficient mice. Science. 2001;294:1531–1533.
- J’Nelle SY, De Asis M, Guttman J, et al. Cortactin depletion results in short tubulobulbar complexes and spermiation failure in rat testes. Biol Open. 2012;1:1069–1077.
- Hess RA, Vogl AW. Sertoli cell anatomy and cytoskeleton. In Griswold MD, editor. Sertoli Cell Biology. Oxford: Elsevier Academic Press; 2015. p. 1–55.
- Lyon KR, Bosseboeuf E, Vogl AW. An alternative model of tubulobulbar complex internalization during junction remodeling in the seminiferous epithelium of the rat testis. Biol Reprod. 2015;93(12):1.
- Vogl AW, Du M, Wang XY, et al. Novel clathrin/actin-based endocytic machinery associated with junction turnover in the seminiferous epithelium. Semin Cell Dev Biol. 2014;30:55-64.
- Vogl AW, J’Nelle SY, Du M. New insights into roles of tubulobulbar complexes in sperm release and turnover of blood-testis barrier. Int Rev Cell Mol Biol. 2013;303:319–355.
- Braun RE, Behringer RR, Peschon JJ, et al. Genetically haploid spermatids are phenotypically diploid. Nature. 1989;337:373.
- Kusumi N, Watanabe M, Yamada H, et al. Implication of amphiphysin 1 and dynamin 2 in tubulobulbar complex formation and spermatid release. Cell Struct Funct. 2007;32:101–113.
- Wistuba J, Stukenborg J-B, Luetjens CM. Mammalian spermatogenesis. Funct Dev Embryol. 2007;1:99–117.
- Greenbaum MP, Iwamori T, Buchold GM, et al. Germ cell intercellular bridges. Cold Spring Harb Perspect Biol. 2011;3:a005850.
- Russell L, Saxena N, Turner T. Cytoskeletal involvement in spermiation and sperm transport. Tissue Cell. 1989;21:361–379.
- Greenbaum MP, Yan W, Wu M-H, et al. TEX14 is essential for intercellular bridges and fertility in male mice. Proc Nat Acad Sci. 2006;103:4982–4987.
- Tokuyasu K, Peacock W, Hardy R. Dynamics of spermiogenesis in drosophila melanogaster. Zeitschrift für Zellforschung und Mikroskopische Anatomie. 1972;127:492–525.
- Fuller M. Spermatogenesis. The development of drosophila. Martinez-Arias M, Bate M, ed. Cold Spring Harbor, New York: Cold Spring Harbor Press; 1993.
- Fabian L, Brill JA. Drosophila spermiogenesis: big things come from little packages. Spermatogenesis. 2012;2:197–212.
- Steinhauer J. Separating from the pack: molecular mechanisms of Drosophila spermatid individualization. Spermatogenesis. 2015;5:e1041345.
- Zhong L, Belote JM. The testis-specific proteasome subunit Prosα6T of D. melanogaster is required for individualization and nuclear maturation during spermatogenesis. Development. 2007;134:3517–3525.
- Laurinyecz B, Péter M, Vedelek V, et al. Reduced expression of CDP-DAG synthase changes lipid composition and leads to male sterility in drosophila. Open Biol. 2016;6:150169.
- Augière C, Lapart J-a, Duteyrat J-L, et al. Salto/CG13164 is required for sperm head morphogenesis in drosophila. Mol Biol Cell. 2019;30:636–645.
- Huang G, Kaufman AJ, Ryan RJ, et al. Mouse DCUN1D1 (SCCRO) is required for spermatogenetic individualization. Public Lib Sci One. 2019;14:e0209995.
- Kwon YT, Ciechanover a. The ubiquitin code in the ubiquitin-proteasome system and autophagy. Trends Biochem Sci. 2017;42:873–886.
- Tang Z, Lin MG, Stowe TR, et al. Autophagy promotes primary ciliogenesis by removing OFD1 from centriolar satellites. Nature. 2013;502:254.
- Pampliega O, Cuervo AM. Autophagy and primary cilia: dual interplay. Curr Opin Cell Biol. 2016;39:1–7.
- Nishida Y, Arakawa S, Fujitani K, et al. Discovery of Atg5/Atg7-independent alternative macroautophagy. Nature. 2009;461:654.
- Ma T, Li J, Xu Y, et al. Atg5-independent autophagy regulates mitochondrial clearance and is essential for iPSC reprogramming. Nat Cell Biol. 2015;17:1379.
- Honda S, Arakawa S, Nishida Y, et al. Ulk1-mediated Atg5-independent macroautophagy mediates elimination of mitochondria from embryonic reticulocytes. Nat Commun. 2014;5:4004.
- Tsuboyama K, Koyama-Honda I, Sakamaki Y, et al. The ATG conjugation systems are important for degradation of the inner autophagosomal membrane. Science. 2016;354:1036–1041.
- Cheon SY, Kim H, Rubinsztein DC, et al. Autophagy, cellular aging and age-related human diseases. Exp Neurobiol. 2019;28:643.
- Yoshii SR, Kuma a, Akashi T, et al. Systemic analysis of Atg5-null mice rescued from neonatal lethality by transgenic ATG5 expression in neurons. Dev Cell. 2016;39:116–130.
- Yoshii SR, Kuma a, Mizushima N. Transgenic rescue of Atg5-null mice from neonatal lethality with neuron-specific expression of ATG5: systemic analysis of adult Atg5-deficient mice. Autophagy. 2017;13:763–764.
- Hara T, Nakamura K, Matsui M, et al. Suppression of basal autophagy in neural cells causes neurodegenerative disease in mice. Nature. 2006;441:885.
- Sadate‐Ngatchou PI, Payne CJ, Dearth AT, et al. Cre recombinase activity specific to postnatal, premeiotic male germ cells in transgenic mice. Genesis. 2008;46:738–742.
- Silva C, Wood JR, Salvador L, et al. Expression profile of male germ cell‐associated genes in mouse embryonic stem cell cultures treated with all‐trans retinoic acid and testosterone. Mol Reprod Dev: Incorporating Gamete Res. 2009;76:11–21.
- Zhang Z, Li W, Zhang Y, et al. Intraflagellar transport protein IFT20 is essential for male fertility and spermiogenesis in mice. Mol Biol Cell. 2016;27:3705–3716.
- Reuter M, Chuma S, Tanaka T, et al. Loss of the Mili-interacting Tudor domain–containing protein-1 activates transposons and alters the Mili-associated small RNA profile. Nat Struct Mol Biol. 2009;16:639.
- Komiya T, Itoh K, Ikenishi K, et al. Isolation and characterization of a novel gene of the DEAD box protein family which is specifically expressed in germ cells of Xenopus laevis. Dev Biol. 1994;162:354–363.
- Johnson LR, Foster JA, Haig-Ladewig L, et al. Assembly of AKAP82, a protein kinase a anchor protein, into the fibrous sheath of mouse sperm. Dev Biol. 1997;192:340–350.