ABSTRACT
Muscle development is a complex process that was regulated by many factors, among which non-coding RNAs (ncRNAs) play a vital role in regulating multiple life activities of muscle cells. Circular RNA (circRNA), a type of non-coding RNA with closed-loop structure, has been reported to affect multiple life processes. However, the roles of circRNAs on muscle development have not been fully elucidated. The present study aimed to determine whether and how circRIMKLB affects muscle development. Our study revealed that circRIMKLB promoted myoblast proliferation and inhibited differentiation. Besides, miR-29c was proved as a downstream target of circRIMKLB using dual-luciferase reporter assay and RNA-binding protein immunoprecipitation (RIP) assay. Also, potassium inwardly rectifying channel subfamily J member 12 (KCNJ12) was identified as a novel target of miR-29c via dual-luciferase reporter assay, quantitative reverse transcriptase-polymerase chain reaction (qRT-PCR), and western blot. CircRIMKLB and KCNJ12 were both proved to regulate cell cycle on muscle regeneration after injury in vivo. In conclusion, we demonstrated that circRIMKLB sponged miR-29c, releasing KCNJ12 to regulate myoblast proliferation and differentiation and regulating cell cycle during muscle regeneration after injury in vivo.
Introduction
Skeletal muscles, which consist of muscle fibres, neurons, vasculature, and connective tissue, are one of the most abundant tissues in the body and perform multiple functions, such as generating movements, producing heat, and storing protein reserves [Citation1–3]. Skeletal muscle originates from the progenitor cells of the somatic mesoderm, except for the oesophageal and facial muscles, during embryonic development [Citation4]. Progenitor cells continue to proliferate and eventually differentiate into mononuclear myoblasts via mesenchymal stem cells. Mononuclear myoblasts fuse into myotubes and eventually differentiate into various muscle fibres. Adult mammalian skeletal muscle is stable under normal conditions, with only the sporadic fusion of satellite cells to compensate for muscle turnover induced by daily wear and tear [Citation5]. However, when an injury occurs, satellite cells are activated, proliferate, and differentiate into myoblasts. Myoblasts then fuse with each other to form multinucleated myotubes, which eventually mature into adult muscle fibres through a process that resembles the myogenesis observed during the embryonic period, suggesting that studying muscle development can provide insights into muscle damage repair [Citation6]. Muscle growth, development, and regeneration are important components of human and animal health. The substantial loss or reduced function of skeletal muscles often leads directly or indirectly to increased morbidity and mortality, promoting the development of secondary diseases, such as diabetes, obesity, and cardiovascular and respiratory diseases [Citation7]. Thus, understanding the underlying mechanisms that regulate skeletal muscle function and development is of critical importance.
Non-coding RNAs (ncRNAs) have been reported to regulate both myogenesis and muscle regeneration. MicroRNAs (miRNAs), a key factor of ceRNA, are a class of small non-coding RNAs that could cause cleavage and degrade mRNAs through binding to the 3'-untranslated region (3'-UTR) [Citation8,Citation9]. MiRNAs are involved in multiple muscular life activities, such as muscle cell proliferation, differentiation, apoptosis, and regeneration [Citation9–11]. MiRNAs have emerged to regulate skeletal muscle function and the aberrant expression of miRNAs is thought to be associated with various muscle diseases [Citation12]. The miR-29 family, containing miR-29a, b, and c, play important roles in regulating skeletal muscle functions. For instance, the miR-29 participated in sarcopenia, and miR-29b participated in regulating multiple types of muscle atrophy [Citation13,Citation14].
Circular RNAs (CircRNAs), which have no 5' caps and 3'tails, are a category of endogenous ncRNAs with covalent, closed-loop structures, increasing their stability and ability to tolerate RNase R compared with other ncRNAs [Citation15–17]. Increasing studies have revealed multiple mechanisms through which circRNAs regulate gene expression. For example, circRNAs have been shown to act as sponges for miRNAs, and to bind proteins as a form of scaffolding. In addition, some circRNAs can be translated into peptides [Citation18–20]. Circular RNAs can be divided into three types, exonic circRNAs, intronic circRNAs, and exonic-intronic circRNAs [Citation21a; Citation22]. In general, most exonic circRNAs are localized in the cytoplasm and are thought to function as miRNA sponges, affecting post-transcriptional regulation, categorizing circRNAs as a type of ceRNA [Citation23]. For example, ciRS-7/CDR1 is thought to inhibit miR-7 during the regulation of hepatocellular carcinoma [Citation24]. Some intronic circRNAs and exonic-intronic circRNAs have been shown to regulate transcription in the nucleus. For example, ci-ankrd52 is concentrated near the transcription site of its host gene and acts together with RNA polymerase II to promote the transcription of its own coding gene, similar to a cis-regulator [Citation22]. In addition, EIciRNAs interact with the U1 small nuclear ribonucleoprotein (snRNP) to enhance the transcription of their parental genes [Citation21b]. CircRNAs are widely involved in various life activities, such as cellular proliferation, differentiation, tissue development, cancer occurrence, and immune responses [Citation25–27]. Although circRNAs have attracted increasing attention in regulating biological processes, there is still a great number of circRNAs whose functions remain unknown. CircRNAs are highly enriched in striated muscle, indicating that circRNAs might represent a novel regulatory network that contributes to skeletal muscle development, myogenesis, and regeneration [Citation23]. However, existing research regarding the effects of circRNA during muscle development is relatively rare, and additional studies on the effects of circRNA are necessary to obtain a deeper understanding of the regulatory network involved in muscle development.
Our previous study found that circRNA ribosomal modification protein rimK-like family member B (circRIMKLB) was abundant in skeletal muscle [Citation28]. However, the function of circRIMKLB remains unclear. Here, this study focused on the regulatory function(s) of circRIMKLB during myogenesis. In this study, circRIMKLB was identified as a true circRNA. This study revealed that circRIMKLB promoted myoblast proliferation, inhibited myoblast differentiation by sponging miR-29c in vitro, and participated in cell cycle regulation during muscle regeneration after injury in vivo. In addition, the potassium inwardly rectifying channel subfamily J member 12 (KCNJ12) was proved to be a novel target of miR-29c. Therefore, circRIMKLB appears to serve as a molecular sponge, absorbing miR-29c and enhancing the expression of KCNJ12 in muscle cells.
Materials and methods
Ethics
All experiments were performed according to the protocols approved by the Animal Care Committee of Northwest A&F University.
Animal experiment
Adult C57BL/6 mice, approximately five weeks old, were purchased from Air Force Medical University (Xi’an, China). All experiments were performed according to the protocols approved by the Animal Care Committee of Northwest A&F University (NWAFU-314020038). The tibialis anterior of the left leg of each mouse was injected with 10 µM cardiotoxin (CTX; Whiga) to induce a muscle injury according to previous study [Citation29]. To verify the successful induction of muscle injury, paraffin sections of the tibialis anterior were used for haematoxylin-eosin (HE) staining 24 h after the injection. Entranster™-in vivo transfection reagent (Engreen Biosystem Co., Ltd.) was used to inject 6.25 µg of pCD2.1-circRIMKLB or pCD2.1 empty plasmid, and pcDNA3.1-KCNJ12 or pcDNA3.1 (+) empty plasmid, into mice, following the manufacturer’s instructions, at 12 h and 48 h after cardiotoxin treatment. The tibialis anterior of the left leg was harvested at 72 h for HE staining, RNA extraction, and protein extraction. As for the cattle samples, the heart, liver, lung, spleen, kidney, muscle tissues were collected from three 90-day-old foetal Qinchuan cattle and three 24-month-old Qinchuan cattle from a local livestock farm of Xi’an (Shannxi, China).
RNase R treatment and actinomycin D assay
To identify the reality of circRIMKLB, 3.0 μg total RNA was incubated with or without 5 U/μg RNase R (D-Mark bioscience, Toronto, CA) at 37°C for 30 min. Then the RNA was purified and stored at −80°C to use. Bovine primary myoblasts were exposed to 2 μg/ml actinomycin D (Sigma, Darmstadt, Germany) for 0 h, 4 h, and 8 h, respectively. Then the total RNA of these cells was extracted by TRIzol reagent and stored at −80°C to use.
Vector construction
The full-length bovine circRIMKLB was cloned into the pCD2.1 plasmid, and the coding sequence (CDS) of bovine KCNJ12 was cloned into the pcDNA3.1 plasmid. A segment of pri-bta-miR-29c, containing pre-bta-miR-29c, was inserted into pcDNA3.1 (+). The 3'-UTR fragments of bovine KCNJ12 (WT-KCNJ12) and the bovine circRIMKLB fragment (WT-circRIMKLB) that contained the bta-miR-29c-binding site were amplified and inserted into the psiCHECK-2 vector (Promega, Madison, WI, USA). The mutant psiCHECK2-KCNJ12-3'UTR-Mut (Mut-KCNJ12) and psiCHECK2-circRIMKLB-Mut (Mut-circRIMKLB) were generated by mutating the sequence complementary to the seed region of miR-29c using a mutagenic primer. The primers used can be found in Supplementary Table 1.
Cell culture and transfections
Bovine primary myoblast cells were isolated from bovine longissimus muscle, as previously described [Citation30]. HEK293T cells were cultured in high-glucose Dulbecco’s modified Eagle’s medium (DMEM), containing 10% foetal bovine serum (FBS; 20% FBS for bovine primary myoblasts) and 1% antibiotics (100 U/ml penicillin, 0.1 mg/L streptomycin), at 37°C in a 5% CO2 environment. The bovine KCNJ12 expression plasmid (pcDNA3.1) was constructed by GENEWIZ (Nanjing, China) using HindIII and EcoRI. KCNJ12-siRNA (5'-ACATCGAGTTCGCCAACAT-3' and circRIMKLB-siRNA(5'-GCTGAACCTCTACAACACT-3') were purchased from Ribo (Guangzhou, China). Plasmids and siRNAs were transfected into bovine primary myoblasts using XfectTM Transfection Reagent (Takara, Dalian, China) or XfectTM RNA Transfection Reagent (Takara, Dalian, China) for plasmid or siRNA following the manufacturer’s instructions.
Cell proliferation assay
Cell Counting Kit-8 (Solarbio, Beijing, China) and Cell-Light EdU DNA cell proliferation kit (RiboBio, Guangzhou, China) were used to investigate the effects of KCNJ12 and circRIMKLB on bovine primary myoblasts. Cells were plated in 96-well plates at a density of 1 × 104, to which were added 10 µL CCK-8 reagent (Solarbio, Beijing, China) 20 h after transfection. After a 4-h incubation, the absorbance values of all samples were detected using an automatic microplate reader (Molecular Devices, Sunnyvale, USA) at 450 nm. Cell proliferation was also detected using the Cell-Light EdU DNA cell proliferation kit (RiboBio, Guangzhou, China), in 96-well plates, at a density of 5 × 103 cells/well, according to the manufacturer’s instructions.
Flow cytometry for the cell cycle assay and apoptosis assay
Bovine primary myoblasts were grown in 60-mm plates at approximately 2 × 106 cells per plate. After 24 h transfection, myoblasts were collected and washed in PBS buffer. Then, 1 mL PI/RNase Staining Buffer (BD Pharmingen™, USA) was added, and 15 min later, the cell suspension was subjected to flow cytometry (BD FACSAria, BD BioSciences, USA).
Cell apoptosis was detected by annexin V-FITC/PI staining assay. Cells were treated based on the manufacturer’s protocol described by the Cell Cycle Testing Kit (BestBio, Shanghai, China), and the cell suspension was analysed by flow cytometry (FACS CantoTM II, BD BioSciences, USA).
Polymerase chain reaction (PCR) and quantitative real-time polymerase chain reaction (qRT-PCR)
To identify circRIMKLB, PCR was performed at 95°C for 2 min, then 30 cycles of 95°C for 10 sec, 62°C for 10 sec, and 72°C for 12 sec, and finally 72°C for 10 min, with or without RNase R treated RNA, respectively. Total RNA in bovine primary myoblasts and mice tibialis anterior muscles was extracted via using RNAiso Plus (TaKaRa, Dalian, China). Total RNA was reverse-transcribed into cDNA using the Prime Script RT reagent Kit (TaKaRa, Dalian, China) which contained PrimeScript RT Enzyme Mix I, according to the manufacturer’s instructions. The SYBR Green Master Mix (Genstar, Beijing, China) was used to perform qRT-PCR. GAPDH was used to normalize gene expression data and U6 was used to normalize miRNA expression data. The relative expression levels of all genes or miRNAs were calculated by the 2−ΔΔCt method. All primers used are listed in Supplementary Table 1.
Western blot analysis
Total proteins were extracted from bovine primary myoblasts using radioimmunoprecipitation assay (RIPA) protein lysis buffer containing 1 mM phenylmethylsulfonyl fluoride (PMSF, Solarbio, Beijing, China). Total proteins were extracted from mouse tibialis anterior muscles using RNAiso Plus reagent (Takara, Dalian, China). A BCA Kit (Takara, Dalian, China) was used to determine protein concentrations. Then, protein samples were boiled with 5× sodium dodecyl sulphate (SDS) loading buffer (Heart, Xian, China) for 10 min at 98°C, and 20 µg total proteins were separated using 10% SDS-polyacrylamide gel electrophoresis (PAGE) gels, and the separated protein samples were transferred onto a 0.2 µM polyvinylidene fluoride (PVDF) membrane (Millipore, Billerica, MA, USA). Membranes were blocked in 5% skim milk powder in Tris-buffered saline containing Tween (TBST) for approximately 2 h at room temperature, followed by incubation with primary antibodies specific for anti-p27 (WL04174, 1:1000, Wanleibio, China), anti-CDK2 (ab64669, 1:1000, Abcam, England), anti-MyoD (ab64159, 1:1000, Abcam, England), anti-MyoG (ab103924, 1:1000, Abcam, England), anti-MyHC (1:1000; GTX20015, GeneTex, USA), anti-KCNJ12 (OM107262, 1:1000, OmnimAbs, USA) anti-β-actin (KM9001T, 1:3000, Sungene Biotech, China) at 4°C overnight. The PVDF membrane was incubated with a secondary antibody for 1.5 h after washing with TBST three times. The membranes were exposed to ECL Plus (Sangon, Shanghai, China) and quantified with the ChemiDoc XRS+ system (Bio-Rad Laboratories, Shanghai, China). All antibodies used are listed in Supplementary Table 2.
Fluorescence in situ hybridization (FISH)
RNA FISH assay was used to localize circRIMKLB using a Dig-labelled probe (5'ATATAGACTCCTCCCAGGTTTCCTTGTTCA-3'), and bta-miR-29c was localized using a Cy3-labelled probe (5'-TAACCGATTTCAGATGGTGCTA-3'). Cells were first treated with 4% paraformaldehyde for 20 min and digested with proteinase K (20 µg/ml) for 8 minutes. After washing with PBS three times, cells on glass coverslips were hybridized with the circRIMKLB probe at 37°C overnight. Then, the cells were washed with 2× SCC, 1× SCC, and 0.5× SCC for 10 min each. Coverslips were blocked in BSA for 30 min, and the cells were then incubated with anti-DIG-HRP and FITC-TSA, followed by the bta-miR-29c probe and 4',6-diamidino-2-phenylindole (DAPI). Cells were observed with an inverted fluorescence microscope (ECLIPSE TI-SR, NIKON, Japan).
Immunocytochemical analysis
Muscle primary myoblasts were induced to differentiate for 4 days, washed with PBS, and fixed with 4% paraformaldehyde for 20 min. Then, 0.5% Triton X-100 dissolved in PBS was used to treat the myoblasts for 20 min. After incubation with 5% BSA for 30 min, myoblasts were incubated with anti-MyHC antibody (1:250; GTX20015, GeneTex, USA) overnight. Next, myoblasts were incubated with Alexa Fluor 594-conjugated goat anti-mouse IgG for 2 h, followed by DAPI for 15 min. Images were then acquired.
Dual-luciferase activity assay
For dual-luciferase activity assays, pcDNA3.1-miR-29c or pcDNA3.1 (+) containing WT- circRIMKLB/Mut-circRIMKLB were co-transfected into HEK293T cells using XFECTTM (Takara, Dalian, China) when cells approached approximately 80% confluency in a 96-well dish. pcDNA3.1-miR-29c or pcDNA3.1 (+) and Wt-KCNJ12 /Mut-KCNJ12 were co-transfected into HEK293T cells using the same method. After incubation for 24 h, the cells were harvested, and luciferase activities were measured using the Dual-Luciferase Reporter Assay System (Promega, Madison, AL) on an MPPC luminescence analyser (HAMAMATSU, Beijing, China).
RNA-binding protein immunoprecipitation (RIP)
To explore whether circRIMKLB functions as a molecular sponge through Argonaute RISC catalytic component 2 (Ago-2) protein, an RNA-binding protein immunoprecipitation assay of the Ago-2 protein was performed with anti-ago2 (ab186733, 1:100, Abcam, England) using an RNA-binding Protein Immunoprecipitation Kit (17–701, Merck, Millipore) with bovine primary myoblasts according to the manufacturer’s instructions. qRT-PCR was performed on the Ago-2 protein-associated RNA mixture, absorbed by the magnetic beads.
Statistical analysis
All experiments were performed in triplicate, and the data are presented as the mean ± standard deviation (SD). Significant differences between groups were determined using the Student’s t-test in SPSS statistical software. A value of p < 0.05 was considered significant.
Results
Identification of the circular structure and expression profile of circRIMKLB
With Sanger sequencing, the back-splicing junction of circRIMKLB was confirmed by using a pair of back-to-back primers (Primer 1) (), and circRIMKLB was formed by independent cyclization of exon 2 (the sequences of circRIMKLB were listed in Supplementary Fig. 1A). Then, a PCR assay was performed with Primer 1 or Primer 2 (convergent primers) that only amplified the linear RIMKLB mRNA to detect the tolerance of circRIMKLB for RNase R. The results showed that circRIMKLB was more stable than linear RIMKLB when treated with RNase R (). Also, the qRT-PCR results suggested a strong tolerance for RNase R of circRIMKLB as there was no significant difference of circRIMKLB expression levels between RNase R treatment group (R+) and control group (R-) while the expression level of linear RIMKLB decreased significantly with RNase R treatment (). Additionally, actinomycin D assay showed that circRIMKLB survived better than linear RIMKLB under actinomycin D treatment conditions at different time points (). The secondary structure of circRIMKLB was also analysed (). These results confirmed that circRIMKLB is a true circRNA. The expression profile for circRIMKLB indicated that it expressed in the heart, liver, spleen, lung, kidney, and skeletal muscle during the foetal stage and had relatively higher expression levels in the lung, kidney, and skeletal muscle (). At the adult stage, circRIMKLB also expressed in the heart, liver, spleen, lung, kidney, and skeletal muscle, and had a relatively lower expression level in the adult skeletal muscle ().
Figure 1. CircRIMKLB is a real circular RNA. (a) The back-splicing site of circRIMKLB was found by Sanger sequencing. (b) PCR product of circRIMKLB was detected by using divergent and convergent primers while RNA was treated RNase R or not. (c) The level of circRIMKLB mRNA was detected by qPCR with divergent primers. (d) The level of linear RIMKLB mRNA was detected by qPCR with convergent primers. (e) circRIMKLB and liner RIMKLB were detected by qRT-PCR after cells were treated with actinomycin D (a transcription inhibitor). (f) The secondary structure of circRIMKLB was analysed via using RNAfold (http://rna.tbi.univie.ac.at/). (g) The expression of circRIMKLB in foetal stage (90 days) was analysed by using qRT-PCR. (h) The expression of circRIMKLB in adult stage (24 months) was analysed by using qRT-PCR. The relative expression levels were normalized to GAPDH.
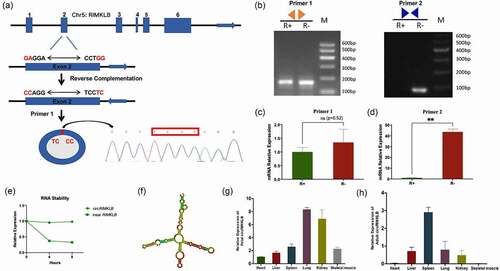
CircRIMKLB promotes proliferation and inhibits the differentiation of bovine primary myoblasts
To confirm the specific expression or inhibition of circRIMKLB plasmid or siRNA without affecting the expression of linear RIMKLB mRNA, the expression levels of circRIMKLB and linear RIMKLB mRNA were detected by qRT-PCR and the results showed that circRIMKLB was overexpressed or inhibited significantly and there was no significant change of linear RIMKLB mRNA expression levels (Supplementary Fig. 1B-F). To clarify the function of circRIMKLB during the development of skeletal muscle, Cell Counting Kit-8 (CCK-8) assay, 5-ethynyl-20-deoxyuridine (EdU) assay, cell phase assay, qRT-PCR, and western blot analyses were performed after 24 h of circRIMKLB overexpression or inhibition. The CCK-8 assay showed that the cell viability increased while circRIMKLB was overexpressed, and the inhibiting group indicated the opposite results ( a and b). The EdU assay showed that overexpressing circRIMKLB increased the percentage of proliferative cells and inhibiting circRIMKLB decreased the percentage of proliferative cells ( c, d). Cell cycle analysis revealed that circRIMKLB increased the proportion of myoblasts in the S phase and decreased the percentage of cells in the G1/G0 phase. However, no effect was observed for the proportion of cells in the G2 phase, suggesting that circRIMKLB may play a crucial role in cell proliferation ( e and b). In addition, qRT-PCR and western blot analyses showed that circRIMKLB promoted the expression levels of cell-proliferation-related genes, including cyclin-dependent kinase inhibitor 1B (CDKN1B or p27), cyclin D1 (CCND1), and cyclin-dependent-kinase 2 (CDK2), at both mRNA and protein levels ( g, h and i).
Figure 2. circRIMKLB promotes proliferation and inhibits differentiation of bovine primary myoblasts. (a) (b) Cell viability was detected by CCK-8 assay while circRIMKLB was overexpressed and inhibited 24 h later. (c) (d) EdU assay was used to detect the cell proliferation index and the percentage of EdU positive cells were counted. (e) (f) Cell cycle phase index was detected by flow cytometry and the percentage of different phases was analysed. (g) (h) The mRNA level of CDK2, CCND1 and p27 in bovine primary myoblasts were detected by qRT-PCR at 24 h post-transfection. (i) The protein levels of CDK2, CCND1 and p27 in bovine primary myoblasts were detected by western blot at 24 h post-transfection. (j) (k) Cell differentiation was measured by immunofluorescence assay, 400 × . (l) (m) The mRNA level of MyoD and MyoG were detected by qRT-PCR after bovine primary myoblasts were induced to differentiate for 4 days. (n) The protein level of MyoD and MyoG were detected by western blot after bovine primary myoblasts were induced to differentiate for 4 days. The relative expression levels were normalized to GAPDH. *p < 0.05; **p < 0.01.
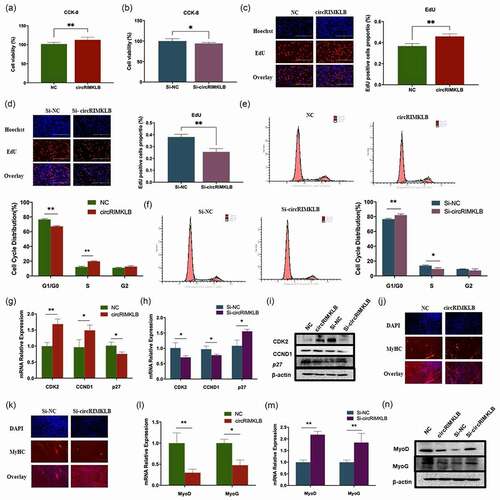
The effects of circRIMKLB on differentiation of myoblasts were explored and myosin heavy chain () immunofluorescence staining of myotubes showed that the number of myotubes reduced or increased when circRIMKLB was overexpressed or inhibited, respectively ( j and k). The qRT-PCR and western blot analyses also indicated that circRIMKLB could inhibit the expression of the differentiation-related genes-MyoD and MyoG ( l, m and n). These results indicated that circRIMKLB promoted the proliferation and inhibited the differentiation of bovine primary myoblasts.
CircRIMKLB acts as a competing endogenous RNA for miR-29c
To explore how circRIMKLB functions, the intracellular location of circRIMKLB was examined with a FISH assay and the results showed circRIMKLB primarily located in the cytoplasm (). The PCR using cytoplasmic RNA or nuclear RNA also showed that circRIMKLB mainly existed in cytoplasm (Supplementary Fig. 2A). As the RNA-induced silencing complex (RISC) that contains the Ago-2 protein is the key for ncRNAs to function as molecular sponges, an RNA-binding protein immunoprecipitation (RIP) assay of Ago-2 was performed and the results showed that
Figure 3. CircRIMKLB functions as a sponge of miR-29c. (a) The location of circRIMKLB was detected in bovine primary myoblasts by FISH assay, 400 × . (b) RIP assay of Ago-2 was used to find whether circRIMKLB could function as a miRNA sponge. (c) MiR-29c and circRIMKLB folding energy was predicted by RNAhybrid.- (d)(e) The levels of miR-29c were detected by qRT-PCR while circRIMKLB was overexpressed or inhibited. (f) miR-29c with psi-CHECK2 plasmid of circRIMKLB-Wt or circRIMKLB-Mut were transfected into HEK293T cells, and Renilla luciferase activity was normalized to the firefly luciferase (hLuc) activity. (g) miR-29c sensor was constructed. (h) The miR-29c sensor was transfected into HEK293T cells together with miR-29c and increasing amounts of pCD2.1-circRIMKLB. Luciferase activities were measured 24 h after transfection. (i) The co-location of miR-29c and circRIMKLB were detected in bovine primary myoblasts by FISH assay.
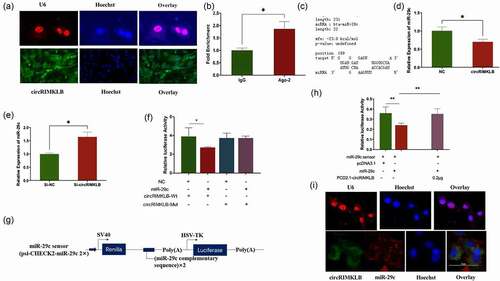
circRIMKLB was enriched in the Ago-2 IP fraction in comparison to the IgG control fraction (). Ago-2 occupancy on circRIMKLB implied circRIMKLB could be integrated into a RISC complex. And seven potential target miRNAs of circRIMKLB were predicted by miRanda (v3.3.a) and RNAhybrid (https://bibiserv.cebitec.uni-bielefeld.de/rnahybrid) [Citation31]. Among the predicted miRNAs, miR-29c was selected with a minimum free energy of −23.0 kcal/mol as it was reported to affect skeletal muscle development ( and Supplementary Table 3). The qRT-PCR results showed that circRIMKLB affected miR-29c expression levels passively as overexpressing circRIMKLB inhibited miR-29c expression level and inhibiting circRIMKLB enhanced miR-29c expression level ( d and e).
Dual-luciferase reporters assay showed that miR-29c was able to markedly suppress the Renilla luciferase (Rluc) reporter activity of wild-type circRIMKLB, which indicated that circRIMKLB could bind to miR-29c (). To verify the direct binding between circRIMKLB and miR-29c, a miR-29c sensor by inserting two copies that perfectly matched miR-29c fragments was constructed into the 3'-UTR of the Rluc gene in the psiCHECKTM-2 vector (). The decreased Rluc activity induced by miR-29c could be recovered by adding pCD2.1-circRIMKLB (), which indicated that circRIMKLB acted as a specific sponge for miR-29c, preventing the inhibition of Rluc expression. The FISH assay also suggested that circRIMKLB could bind to miR-29c in the cytoplasm as the locations of circRIMKLB and miR-29c were overlapped ().
KCNJ12 is a novel target of miR-29c
The main manner of miRNA functions is to sponge mRNAs [Citation32]. Based on this, KCNJ12 was predicted to be a potential miR-29c target according to TargetScan (Supplementary Fig. 2B). In addition, the comparison of miR-29c sequences among cattle, humans, mice, and rats showed a high degree of conservation of miR-29c (Supplementary Fig. 2C). Dual-luciferase assays were performed to detect the interaction between miR-29c and the 3'-UTR of KCNJ12, and the results indicated that miR-29c markedly repressed the activity of the Rluc reporter which contained the wild-type KCNJ12 (WT-KCNJ12) 3'-UTR, but did not affect the Rluc activity of the mutant KCNJ12 (MUT-KCNJ12) 3'-UTR (). Next, qRT-PCR and western blot analyses revealed that miR-29c significantly suppressed the expression of KCNJ12 at both the mRNA and protein levels (), indicating that KCNJ12 was a target of miR-29c.
Figure 4. KCNJ12 is a novel target of miR-29c. (a) miR-29c and psi-CHECK2 plasmid inserted with 3'UTR of WT-KCNJ12 or MUT-KCNJ12 were transfected into HEK293T cells, and Renilla luciferase activity was normalized to the firefly luciferase (hLuc) activity. (b) The sequence of predicted sites of KCNJ12 binding with miR-29c their locations in the psi-CHECK2 plasmid. (c) KCNJ12 mRNA expression in bovine primary cells was detected by qRT-PCR at 24 h post transfection with miR‐29c. (d) Protein expression of KCNJ12 was detected by western blot. The relative expression levels were normalized to GAPDH. *p < 0.05; **p < 0.01.
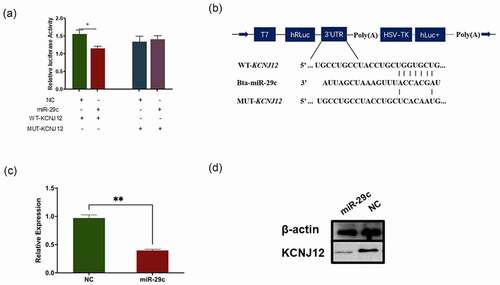
KCNJ12 promotes the proliferation and inhibits the differentiation of bovine primary myoblasts
To determine the effects of KCNJ12 on muscle development, KCNJ12 was overexpressed or inhibited in bovine primary myoblasts, and the expression efficiency as well as inhibition efficiency were detected and the results showed KCNJ12 was significantly overexpressed or inhibited ( a,b). The results of the CCK-8 assay showed that the overexpression of KCNJ12 enhanced the cell viability of myoblasts, whereas the inhibition of KCNJ12 decreased cell viability ( c, d). The EdU assay indicated that the overexpression of KCNJ12 increased the percentage of EdU-positive cells, whereas inhibiting the expression of KCNJ12 decreased the percentage of EdU-positive cells ( e and f). The cell cycle assay showed that KCNJ12 overexpression increased the percentage of S-phase cells and decreased the percentage of G1-phase cells and inhibition of KCNJ12 revealed the opposite results ( g and h). In addition, the overexpression of KCNJ12 also promoted protein expression levels of CDK2 and CCND1, and inhibited the expression of p27, whereas KCNJ12 inhibition showed the opposite results (). These results indicated that KCNJ12 could affect the proliferation of bovine primary myoblasts.
Figure 5. KCNJ12 promotes the proliferation and inhibits the differentiation of bovine primary myoblasts. (a) The expression efficiency of pcDNA3.1-KCNJ12 was detected by qRT-PCR. (b) The inhibition efficiency of Si-KCNJ12 was detected by qRT-PCR. (c)(d) The cells were treated with pcDNA3.1(NC), pcDNA3.1‐KCNJ12(KCNJ12), Si-RNA negative control (Si-NC), and Si-RNA for KCNJ12(Si-KCNJ12) and cell verbality was detected via CCK-8 assay 24 h after transection. (e)(f) EdU assay detected the cell proliferation index and analysis results of EdU‐positive cells. (g)(h) Cell cycle phase indexes were analysed by flow cytometry and the distribution of cell phase was analysed. (i) The protein levels of CDK2, CCND1 and p27 were detected by Western Blot. (j)(k) The mRNA levels of MyoD and MyoG were detected by qRT-PCR. (l) The protein levels of MyoD and MyoG were detected by Western Blot. The relative expression levels were normalized to GAPDH. *p < 0.05; **p < 0.01.
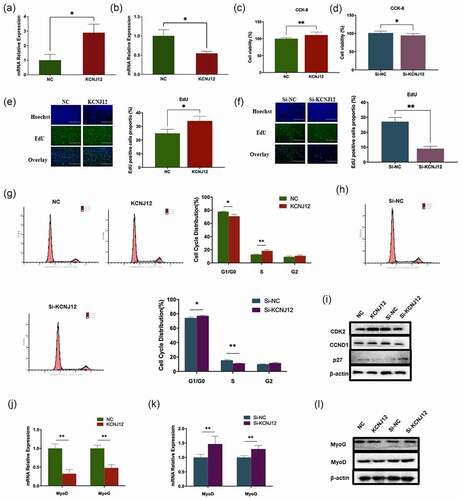
The effects of KCNJ12 on myoblast differentiation were detected by qRT-PCR and western blot, and the results showed that the mRNA levels of MyoD and MyoG were significantly downregulated when KCNJ12 was overexpressed, whereas the inhibition of KCNJ12 showed the opposite results (). These results indicated that KCNJ12 inhibited muscle cell differentiation.
CircRIMKLB and KCNJ12 participate in cell cycle regulation during muscle regeneration after injury in vivo
As circRIMKLB was observed to influence the proliferation of bovine primary myoblasts, it might also affect muscle cell regeneration. Mice were injected with cardiotoxin, and haematoxylin-eosin staining demonstrated the successful construction of a skeletal muscle injury model (). Haematoxylin-eosin staining also showed that muscle injury repair was enhanced following the overexpression of circRIMKLB (). Additionally, qRT-PCR and western blot analyses showed that the expression efficiency of circRIMKLB and the levels of CDK2 and CCND1 were upregulated, and p27 was decreased when circRIMKLB was overexpressed (). Together, these results suggested that circRIMKLB participated in muscle cell regeneration and played a role in cell cycle regulation in vivo.
Figure 6. CircRIMKLB and KCNJ12 participate in cell cycle regulation in muscle regeneration after injury in vivo. (a) Normal and injured muscle at the tibialis anterior of the left leg of mice was checked by haematoxylin–eosin staining, 100 × . (b) The injured muscle injected with NC or circRIMKLB 2 times was checked by haematoxylin–eosin staining 3 days later, 200 × . (c) The expression efficiency of circRIMKLB was checked by qRT-PCR. (d) CDK2, CCND1 and p27 mRNA expressions of mouse tibialis anterior were detected by qRT-PCR at 3 days post-transfection. (e) CDK2, CCND1 and p27 protein levels of mouse tibialis anterior were detected by western blot at 3 days after transfection. (f) The injured muscle injected with NC or KCNJ12 was checked by haematoxylin–eosin staining 3 days later, 200 × . (g) The expression efficiency of KCNJ12 was checked by qRT-PCR. (h) CDK2, CCND1 and p27 mRNA expressions of mouse tibialis anterior were detected by qRT-PCR at 3 days post-transfection. (i) CDK2, CCND1 and p27 protein levels of mouse tibialis anterior were detected by western blot at 3 days after transfection. The relative expression levels were normalized to GAPDH. *p < 0.05; **p < 0.01.
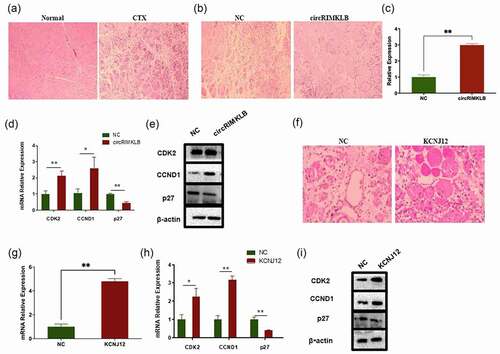
As a target gene of miR-29c, KCNJ12 is also likely to affect muscle injury repair. The conservation of the KCNJ12 was analysed among humans, mice, rats, and cattle and the results showed that its amino acid sequence was highly conserved (Supplementary Fig. 2D). Both ordered and disordered regions of the KCNJ12 protein sequence were highly conserved (Supplementary Fig. 2E). Haematoxylin-eosin staining showed that the repair of injured muscles in mice was promoted after overexpressing KCNJ12 (). After detecting the expression efficiency of KCNJ12, further tests demonstrated that the mRNA and protein levels of CDK2 and CCND1 increased, whereas p27 decreased after KCNJ12 was overexpressed (). Therefore, KCNJ12 might participate in cell cycle regulation, affecting the progress of muscle repair after injury.
Discussion
Skeletal muscle development is orchestrated by a complex network involving transcription factors and epigenetic regulators [Citation33]. To date, the specific functions of ncRNAs largely remain unknown, especially for circRNAs. In this study, circRIMKLB was identified as a circRNA and functions as a promoter of myoblast proliferation, an inhibitor of myoblasts differentiation, and a regulator of the cell cycle and muscle regeneration via sponging miR-29c to release KCNJ12.
To identify a circRNA, it is important to recognize the closed-loop structure. In this study, three strategies were performed. Firstly, Sanger sequencing was used to identify the back-splicing junction of circRIMKLB as it is a characteristic of circRNAs. Secondly, we performed multiple tests, PCR and qRT-PCR, to detect the tolerance of circRIMKLB to RNase R as the closed-loop structure had a strong resistance to circRIMKLB. Additionally, the stability of circRIMKLB was detected by actinomycin D assay. The multiple tests improve the confidence of circRIMKLB as a real circRNA.
Our study revealed circRIMKLB promoted the proliferation and inhibited the differentiation of myoblasts using CCK8, EdU, flow cytometry, qRT-PCR, and western blot assays, providing evidence of circRNA acted as a functional molecular regulator of muscle development. CircRNAs were initially regarded as endogenous RNA splicing by-products or intermediates that escaped from intron lariat debranching, with no function [Citation18,Citation34–36]. However, emerging studies have indicated that circRNAs exert enormous effects that regulate various activities in cells as well as the components of extracellular fluids, such as exosomes[Citation32,Citation37]. To explore the underlying mechanism of circRIMKLB action, RNAhybrid was used to predict the target miRNA. Dual-luciferase assay and qRT-PCR analysis revealed that miR-29c is a target of circRIMKLB. MiR-29c is a highly expressed miRNA found in muscle tissue and important for muscle development. And KCNJ12 was identified as a novel target gene of miR-29c. Based on the high level of conservation in the miR-29c and KCNJ12 sequences between mice and cattle, the influence of circRIMKLB was examined in mouse muscle injury. CircRIMKLB was involved in cell cycle regulation in vivo, which affected muscle regeneration after injury.
All three family members played roles in epigenetic regulation. For instance, participates in the regulation of the t-cell leukaemia-lymphoma (TCL1) oncogene in B-cell chronic lymphocytic leukaemia. MiR-29c directly targets DNA methyltransferase 3 alpha (DNMT3a) and DNA methyltransferase 3 beta (DNMT3b) in lung cancer and acute myeloid leukaemia [Citation38–40]. In addition to DNA methylation, also participated in post-translational modification of histones. Histone deacetylases (HDACs) are responsible for removing acetyl groups that could affect the binding between DNA and histone. mimics were found to be an inhibitor of class II , and overexpression lead to increased acetylation of histone H4 [Citation12]. Due to the global expression of miR-29 family members, many physiological and pathological processes were affected by miR-29a, b and c [Citation2]. It was demonstrated that miR-29s had strong antifibrotic effects in many organs, including heart, kidney, liver and muscle [Citation41–43]. For example, all three members of the miR-29 family were found to be significantly downregulated in the livers of hepatic fibrogenesis mice [Citation42].
Emerging evidence has indicated that miR-29 is important for muscle function. In human muscle, miR-29c is downregulated in quiescent satellite cells compared with proliferating satellite cells [Citation44]. The miR-29 family affects the expression of many muscle-important factors, such as HDAC4, YY1 transcription factor (YY1), ring finger protein 1 (Ring1), Ring1- and YY1-binding protein (Rybp), AKT serine/threonine kinase 3 (Akt3), collagen type XVII alpha 1 chain (Col), and LIM zinc finger domain containing 1 (Lims1), suggesting that the miR-29 family is critical to muscle development [Citation33,Citation45–48]. In some muscle-related diseases, the miR-29 family also plays important regulatory roles. For example, in Duchenne muscle and myoblasts, miR-29 participated in regulating fibrosis in skeletal muscle [Citation43]. In primary muscular dystrophy, 185 miRNAs, including miR-29c, were found to be either upregulated or downregulated in 10 major muscle disorders in humans [Citation3]. All of these studies indicate that the miR-29 family is closely related to muscle development. Also, the manners of how miR-29 regulated muscular functions varied. The factors involved in regulating of miR-29 expression and the genes regulated by the miR-29 family are all important to muscle function. This study found that circRIMKLB regulated the expression of miR-29c and also identified KCNJ12 as a new target gene for miR-29c in muscle tissue.
KCNJ12 (also called Kir2.2), a member of the Kir2 subfamily, encodes an inwardly rectifying potassium channel, which plays an important physiological role in the maintenance of normal cellular excitability and a normal potassium balance in blood [Citation49,Citation50]. Blocking the cellular trafficking of KCNJ12 and KCNJ2 to the cell surface has been associated with hyperthyroidism in thyrotoxic periodic paralysis [Citation51]. In addition, recent studies have shown that KCNJ12 is involved in the muscle contraction process [Citation52]. Our study demonstrated that KCNJ12 expression promoted the proliferation and inhibited the differentiation of bovine primary myoblasts. In addition, the study explored the influence of KCNJ12 on muscle regeneration, and our experiments in mice suggested that KCNJ12 was able to influence the cell cycle in vivo during the process of muscle regeneration after injury. Previous studies showed that KCNJ12 promoted cancer cell proliferation and controlled the tumour growth [Citation53]. Further experiments proved that KCNJ12 increased RelA phosphorylation at S536, activated the transcription factor and increased the expression level of nuclear factor kappa-light-chain-enhancer of activated B cells (NF-κB) targets, including CCND1, matrix metalloproteinase 9 (MMP9), and vascular endothelial growth factor (VEGF) [Citation53]. We speculated that KCNJ12 might promote bovine primary cell proliferation through a similar approach. However, the specific approaches of how KCNJ12 functions in cattle still require more researches.
Due to the important role of miR-29c in muscle diseases, the effects of circRIMKLB on the muscle damage repair process were studied, which indicated that circRIMKLB was able to participate in the cell cycle regulation of myoblasts associated with muscle regeneration caused by damage, resulting in down-regulation of p27 and the upregulation of CDK2 and CCND1 expression. CircRIMKLB is predicted to affect the expression of miR-29c in some muscle diseases and might be directly involved in the development of some muscle diseases. Owning to the high level of conservation observed for both circRIMKLB and KCNJ12 sequences in mice and cattle, these effects could be studied in mice. However, due to differences between these species, whether other factors affected the results of the trial requires further research.
The study identified a novel circRNA, named circRIMKLB, examined its function, and explored its mechanism. CircRIMKLB acts as an endogenous miR-29c sponge, releasing KCNJ12, promoting bovine myoblast proliferation and inhibiting differentiation by affecting the cell phase during muscle regeneration. CircRIMKLB may serve as a potential candidate for the regulation of muscle function
Ethics approval
All experiments were performed according to the protocols approved by the Animal Care Committee of Northwest A&F University.
Authors’ contributions
J. Wang performed most experiments, summarized most part of data, and drafted the manuscript. Y.F. Wen and J.W. Xu performed qRT-PCR. B.L. Yue performed animal experiments. L. Zheng and J.L. Zhong summarized part of data and did the informatic analysis. C.Z Lei, H. Chen and Y.Z. Huang contributed to the experimental design and approved the final version.
Supplemental Material
Download Zip (245.9 KB)Acknowledgments
The authors thank Xiaoting Ding for her help in PCR assay and FISH assay.
Disclosure statement
No potential conflict of interest was reported by the author(s).
Data availability statement
All datasets generated for this study are available within the article and its supplementary materials.
Supplementary material
Supplemental data for this article can be accessed here
Additional information
Funding
References
- Endo T. Molecular mechanisms of skeletal muscle development, regeneration, and osteogenic conversion. Bone. 2015;80:2–13.
- Frontera WR, Ochala J. Skeletal muscle: a brief review of structure and function. Calcif Tissue Int. 2015;96(3):183–195.
- Horak M, Novak J, Bienertova-Vasku J. Muscle-specific microRNAs in skeletal muscle development. Dev Biol. 2016;410(1):1–13.
- Christ B, Ordahl CP. Early stages of chick somite development. Anat Embryol (Berl). 1995;191(5):381–396.
- Yin H, Price F, Rudnicki MA. Satellite cells and the muscle stem cell niche. Physiol Rev. 2013;93(1):23–67.
- Karalaki M, Fili S, Philippou A, et al. Muscle regeneration: cellular and molecular events. Vivo. 2009;23(5):779–796.
- Guller I, Russell AP. MicroRNAs in skeletal muscle: their role and regulation in development, disease and function. J Physiol. 2010a;588(21):4075–4087.
- Lau NC, Lim LP, Weinstein EG, et al. An abundant class of tiny RNAs with probable regulatory roles in Caenorhabditis elegans. Science. 2001;294(5543):858–862.
- Lee RC, Ambros V. An extensive class of small RNAs in Caenorhabditis elegans. Science. 2001;294(5543):862–864.
- Brzeszczynska J, Johns N, Schilb A, et al. Loss of oxidative defense and potential blockade of satellite cell maturation in the skeletal muscle of patients with cancer but not in the healthy elderly. Aging (Albany NY). 2016;8(8):1690–1702.
- Brzeszczynska J, Meyer A, McGregor R, et al. Alterations in the in vitro and in vivo regulation of muscle regeneration in healthy ageing and the influence of sarcopenia. J Cachexia Sarcopenia Muscle. 2018;9(1):93–105.
- Amodio N, Stamato MA, Gulla AM, et al. Therapeutic targeting of miR-29b/HDAC4 epigenetic loop in multiple myeloma. Mol Cancer Ther. 2016;15(6):1364–1375.
- Jung HJ, Lee K-P, Ki-Sun, et al. MicroRNAs in skeletal muscle aging: current issues and perspectives. J Gerontol. 2018;74(7): 1008–1014.
- Li J, Chan MC, Yu Y, et al. MiR-29b contributes to multiple types of muscle atrophy. Nat Commun. 2017;8(1):15201.
- Ashwal-Fluss R, Meyer M, Pamudurti NR, et al. CircRNA biogenesis competes with pre-mRNA splicing. Mol Cell. 2014;56(1):55–66.
- Capel B, Swain A, Nicolis S, et al. Circular transcripts of the testis-determining gene Sry in adult mouse testis. Cell. 1993;73(5):1019–1030.
- Jeck WR, Sharpless NE. Detecting and characterizing circular RNAs. Nat Biotechnol. 2014a;32(5):453–461.
- Cocquerelle C, Mascrez B, Hetuin D, et al. Mis-splicing yields circular RNA molecules. FASEB J. 1993a;7(1):155–160.
- Haque S, Harries LW. Circular RNAs (circRNAs) in health and disease. Genes (Basel). 2017;8(12):353.
- Pamudurti NR, Bartok O, Jens M, et al. Translation of CircRNAs. Mol Cell. 2017;66(1):9–21.
- Li Z, Huang C, Bao C, et al. Exon-intron circular RNAs regulate transcription in the nucleus. Nat Struct Mol Biol. 2015a;22(3):256–264.
- Zhang Y, Zhang XO, Chen T, et al. Circular intronic long noncoding RNAs. Mol Cell. 2013a;51(6):792–806.
- Greco S, Cardinali B, Falcone G, et al. Circular RNAs in muscle function and disease. Int J Mol Sci. 2018;19(11):3454.
- Peng L, Yuan XQ, Li GC. The emerging landscape of circular RNA ciRS-7 in cancer (Review). Oncol Rep. 2015;33(6):2669–2674.
- Bonizzato A, Gaffo E, Te KG, et al. CircRNAs in hematopoiesis and hematological malignancies. Blood Cancer J. 2016;6(10):e483.
- Militello G, Weirick T, John D, et al. Screening and validation of lncRNAs and circRNAs as miRNA sponges. Brief Bioinform. 2017;18(5):780–788.
- Zhou R, Wu Y, Wang W, et al. Circular RNAs (circRNAs) in cancer. Cancer Lett. 2018;425:134–142.
- Wei X, Li H, Yang J, et al. Circular RNA profiling reveals an abundant circLMO7 that regulates myoblasts differentiation and survival by sponging miR-378a-3p. Cell Death Dis. 2017;8(10):e3153.
- Song C, Wang J, Ma Y, et al. Linc-smad7 promotes myoblast differentiation and muscle regeneration via sponging miR-125b. Epigenetics-US. 2018;13(6):591–604.
- Miyake M, Takahashi H, Kitagawa E, et al. AMPK activation by AICAR inhibits myogenic differentiation and myostatin expression in cattle. Cell Tissue Res. 2012;349(2):615–623.
- Rehmsmeier M, Steffen P, Hochsmann M, et al. Fast and effective prediction of microRNA/target duplexes. RNA. 2004;10(10):1507–1517.
- Memczak S, Jens M, Elefsinioti A, et al. Circular RNAs are a large class of animal RNAs with regulatory potency. Nature. 2013a;495(7441):333–338.
- Zhou L, Wang L, Lu L, et al. A novel target of microRNA-29, Ring1 and YY1-binding protein (Rybp), negatively regulates skeletal myogenesis. J Biol Chem. 2012a;287(30):25255–25265.
- Kopczynski CC, Muskavitch MA. Introns excised from the Delta primary transcript are localized near sites of Delta transcription. J Cell Biol. 1992;119(3):503–512.
- Pasman Z, Been MD, Garcia-Blanco MA. Exon circularization in mammalian nuclear extracts. RNA. 1996;2(6):603–610.
- Qian L, Vu MN, Carter M, et al. A spliced intron accumulates as a lariat in the nucleus of T cells. Nucleic Acids Res. 1992;20(20):5345–5350.
- Li Y, Zheng Q, Bao C, et al. Circular RNA is enriched and stable in exosomes: a promising biomarker for cancer diagnosis. Cell Res. 2015;25(8):981–984.
- Fabbri M, Garzon R, Cimmino A, et al. MicroRNA-29 family reverts aberrant methylation in lung cancer by targeting DNA methyltransferases 3A and 3B. Proc Natl Acad Sci U S A. 2007;104(40):15805–15810.
- Garzon R, Liu S, Fabbri M, et al. MicroRNA-29b induces global DNA hypomethylation and tumor suppressor gene reexpression in acute myeloid leukemia by targeting directly DNMT3A and 3B and indirectly DNMT1. Blood. 2009;113(25):6411–6418.
- Pekarsky Y, Santanam U, Cimmino A, et al. Tcl1 expression in chronic lymphocytic leukemia is regulated by miR-29 and miR-181. Cancer Res. 2006;66(24):11590–11593.
- Kriegel AJ, Liu Y, Fang Y, et al. The miR-29 family: genomics, cell biology, and relevance to renal and cardiovascular injury. Physiol Genomics. 2012;44(4):237–244.
- Roderburg C, Urban GW, Bettermann K, et al. Micro-RNA profiling reveals a role for miR-29 in human and murine liver fibrosis. Hepatology. 2011;53(1):209–218.
- Zanotti S, Gibertini S, Curcio M, et al. Opposing roles of miR-21 and miR-29 in the progression of fibrosis in Duchenne muscular dystrophy. Biochim Biophys Acta. 2015;1852(7):1451–1464.
- Koning M, Werker PM, van Luyn MJ, et al. A global downregulation of microRNAs occurs in human quiescent satellite cells during myogenesis. Differentiation. 2012;84(4):314–321.
- McFarlane C, Vajjala A, Arigela H, et al. Negative auto-regulation of myostatin expression is mediated by Smad3 and microRNA-27. PLoS One. 2014;9(1):e87687.
- Wei W, He HB, Zhang WY, et al. MiR-29 targets Akt3 to reduce proliferation and facilitate differentiation of myoblasts in skeletal muscle development. Cell Death Dis. 2013;4(6):e668.
- Winbanks CE, Wang B, Beyer C, et al. TGF-beta regulates miR-206 and miR-29 to control myogenic differentiation through regulation of HDAC4. J Biol Chem. 2011;286(16):13805–13814.
- Zhou L, Wang L, Lu L, et al. Inhibition of miR-29 by TGF-beta-Smad3 signaling through dual mechanisms promotes transdifferentiation of mouse myoblasts into myofibroblasts. PLoS One. 2012c;7(3):e33766.
- Kaibara M, Ishihara K, Doi Y, et al. Identification of human Kir2.2 (KCNJ12) gene encoding functional inward rectifier potassium channel in both mammalian cells and Xenopus oocytes. FEBS Lett. 2002;531(2):250–254.
- Wible BA, De Biasi M, Majumder K, et al. Cloning and functional expression of an inwardly rectifying K+ channel from human atrium. Circ Res. 1995;76(3):343–350.
- Rennels MB, Wasserman SS, Glass RI, et al. Index - rosenberg’s molecular and genetic basis of neurological and psychiatric disease (Fifth edition). Pediatrics. 1995;96(6):1132–1136.
- Zhou Y, Utsunomiya YT, Xu L, et al. Genome-wide CNV analysis reveals variants associated with growth traits in Bos indicus. BMC Genomics. 2016;17(1):419.
- Lee I, Lee SJ, Kang TM, et al. Unconventional role of the inwardly rectifying potassium channel Kir2.2 as a constitutive activator of RelA in cancer. Cancer Res. 2013;73(3):1056–1062.