ABSTRACT
Glutathione peroxidase 8 (GPX8) is a key regulator of redox homoeostasis. Whether its antioxidant activity participates in the regulation of m6A modification is a crucial issue, which has important application value in cancer treatment. In this study, MeRIP-seq was used to explore the characteristics of transcriptome-wide m6A modification in GPX8-deficient oral cancer cells. Oxidative stress caused by the lack of GPX8 resulted in 1,279 hyper- and 2,287 hypo-methylated m6A peaks and 2,036 differentially expressed genes in GPX8-KO cells. Twenty-eight differentially expressed genes were related to the cell response to oxidative stress, and half of them changed their m6A modification. In GPX8-KO cells, m6A regulators IGF2BP2 and IGF2BP3 were upregulated, while FTO, RBM15, VIRMA, ZC3H13, and YTHDC2 were downregulated. After H2O2 treatment, the expression changes of RBM15, IGF2BP2, and IGF2BP3 were further enhanced. These data indicated that GPX8-mediated redox homoeostasis regulated m6A modification, thereby affecting the expression and function of downstream genes. This study highlights the possible significance of GPX8 and the corresponding m6A regulatory or regulated genes as novel targets for antioxidant intervention in cancer therapy.
Key policy highlights
Lack of GPX8 caused oxidative stress of oral cancer cells.
Oxidative stress induced by GPX8 deficiency reprogrammed m6A epitranscriptome.
GPX8 deletion–caused oxidative stress regulated expression of m6A regulatory genes.
m6A modification of antioxidant genes is the adaptive response of cells to oxidative stress.
Introduction
Glutathione peroxidase (GPX) family proteins play an important role in maintaining cell redox balance and normal cell function [Citation1]. GPX8 is the most recently identified member of this family. Its peroxidase activity and endoplasmic reticulum (ER) localization can prevent the leakage of H2O2 from ER and maintain the ER redox control [Citation2]. GPX8 has a broad range of biological functions. It was found to be a cellular substrate of the hepatitis C virus NS3-4A protease [Citation3]. GPX8 can regulate Ca2+ storage and flux in ER and its expression was related to the concentration of Ca2+ in the ER and the flux of Ca2+ in cytoplasm and mitochondria [Citation4]. GPX8 protected insulin-secreting INS-1E beta-cells against lipotoxicity by improving the ER antioxidative capacity [Citation5]. It was reported that GPX8 is a negative regulator of caspase 4/11, which can prevent against colitis [Citation6]. GPX8 deficiency impacted on the lipid composition of cancer cell microsomal membranes [Citation7]. GPX8 was also identified as a key gene involved in the spermatogenesis in patients with cryptorchidism [Citation8].
There is increasing evidence that GPX8 is dysregulated in various tumours. Existing studies have shown that GPX8 is related to tumour progression and prognosis. Bioinformatics analysis revealed that the expression of GPX8 was positively correlated with progression and poor prognosis in patients with gastric cancer [Citation9,Citation10], pancreatic cancer [Citation11], colon cancer, and lung cancer [Citation12,Citation13]. GPX8 can also inhibit the apoptosis of tumour cells and promote their migration and invasion by regulating epithelial properties [Citation13]. Elevated GPX8 activated Wnt signalling pathway to promote the proliferation, migration, and invasion of gastric cancer cells [Citation14]. Histone deacetylase inhibitors inhibited the expression of GPX8, which makes hepatocellular carcinoma sensitive to ER stress and apoptosis through oxidative stress [Citation15].
Oxidative stress is characterized by the excessive production of reactive oxygen species (ROS), which is widely recognized as a key factor in many pathophysiological processes and cancer development [Citation16]. ROS has dual biological properties, playing an anti-tumour or tumour promoting role in different tumours [Citation16,Citation17]. Elevated levels of ROS are commonly observed in cancer cells and generally play a tumour promoting role, but too high level of ROS is toxic to cancer cells [Citation18]. Normal cells adopt several mechanisms to maintain intracellular ROS levels and overall redox homoeostasis. They can express antioxidant enzymes such as superoxide dismutase, catalase, and glutathione peroxidase and produce nonenzymatic antioxidants such as glutathione and thioredoxin, to protect them from ROS damage [Citation19]. In order to adapt to relatively high levels of ROS, cancer cells must enhance their antioxidant capacity to neutralize the cytotoxicity of excessive ROS [Citation17]. Antioxidant protection treatment converts ROS into less reactive species, which can neutralize the harmful effect of ROS [Citation16].
Conventional chemotherapy and radiotherapy are closely related to oxidative stress and can cause ROS-mediated DNA damage and apoptosis. For example, the generation of ROS contributes to the cytotoxicity of 5-fluorouracil, and antioxidant treatment facilitates the drug resistance of tumour cells to 5-fluorouracil [Citation20,Citation21]. The arsenic-mediated elevation of ROS induces apoptosis and pyroptosis in a variety of cancer cells [Citation22,Citation23]. Ionizing radiation-mediated elevation of ROS induces oxidative damage and ferroptosis [Citation24–26]. Excessive levels of ROS induced by ionizing radiation in radiotherapy will destroy the redox homoeostasis, leading to oxidative stress that may result in cell death. On the contrary, tumour cells remove excess ROS by activating endogenous antioxidant enzymes, thereby generating radioresistance [Citation27]. Although antioxidant therapy is a potential strategy for ROS-induced cancer, antioxidant treatment may increase the risk of some cancers and promote their progression [Citation16,Citation28]. Therefore, the regulation of oxidative stress is related to the efficacy of tumour therapy.
N6-methyladenosine (m6A) is the most common eukaryotic mRNA modification, which widely regulates RNA transcription, maturation, translation, and metabolism, thus affecting various physiological and pathological processes, including oxidative stress and tumorigenesis. The modification of m6A is a dynamic and reversible process, which is coordinated by methyltransferase/m6A writer and demethylases/m6A eraser. The m6A writers include methyltransferase like-3 (METTL3)/METTL14/METTL16, Wilms tumour 1-associated protein (WTAP), RNA binding motif protein 15 (RBM15), RBM15B, Vir-Like m6A methyltransferase associated (VIRMA), and zinc finger CCCH-type containing 13 (ZC3H13), which transfer methyl to the target sites. The m6A erasers mainly include fat mass and obesity-associated protein (FTO), AlkB homolog 5 (ALKBH5) and ALKBH3, which can remove m6A from the modified sites of RNAs. The m6A reader proteins recognize m6A modification of RNAs, mediating downstream biological functions. Several classes of proteins act as m6A readers, including YT521-B homology (YTH) domain-containing proteins YTHDC1/2 and YTHDF1/2/3, insulin-like growth factor 2 mRNA binding protein 1 (IGF2BP1)/IGF2BP2/IGF2BP3, heterogeneous nuclear ribonucleoproteins HNRNPA2B1 and HNRNPC, and eukaryotic initiation factor 3 (eIF3).
The m6A modification is closely related to oxidative stress. The cross-talk between oxidative stress and m6A modification and its significance in the occurrence and development of tumours are very complicated. First, ROS can dynamically regulate the expression and activity of m6A regulators, thereby changing cellular m6A level [Citation29,Citation30]. On the other hand, m6A modification of the genes related oxidative stress may regulate their own expression, thus affecting the balance of oxidation and antioxidation, as well as consequent occurrence and progression of tumours [Citation31,Citation32]. Therefore, understanding the interaction mechanism between oxidative stress and m6A methylation in tumours is of great significance for tumour therapy. In a previous study, we found that GPX8 was upregulated in oral cancer cells. However, it is not clear whether its antioxidant activity involves the regulation of m6A modification in cells. In this study, we profiled the transcriptome-wide m6A methylome in GPX8-deficient oral cancer cells through MeRIP-seq and measured the expression of major m6A regulatory genes in order to understand the effect of oxidative stress induced by antioxidant gene deletion on m6A modification. This will help to evaluate the cross-talk between oxidative stress and m6A modification and find novel targets for tumour therapy.
Materials and methods
Construct and generation of GPX8 knockout cell line
Oral squamous cell carcinoma cell SCC-9 was provided by Shanghai Guandao Biological Engineering Co., Ltd. (Sgdbio, Shanghai, China) [Citation33,Citation34]. GPX8-KO SCC-9 cells was derived from SCC-9 cells, in which GPX8 gene was edited by clustered regularly interspaced short palindromic repeats (CRISPR)/CRISPR associated protein 9 (Cas9) system. Briefly, according to the sequence GPX8 gene, two sgRNAs targeting 5’ end of GPX8 open reading frame (aactgcaaatacctttgctc and tttagcgggtaagctgcaag) were designed and cloned into lentiCRISPR-v2 vector. The 293T cells were incubated with constructs and lentiviral packaging plasmids psPAX2 and pMD2.G for 66 h. The resulting supernatant containing lentiviral particles was filtered with 0.45 µm filter and concentrated. 2.2 × 105 SCC-9 cells in a well of 6-well plate were infected with 500 µL of concentrated virus. After 48 h of infection, the cells were screened in the medium with 0.3 μg/mL puromycin. The concentration of puromycin-resistant cells was adjusted to 5 cells/mL, and the cells were seeded into a 96-well plate at 100 µl per well to isolate a single clone. The knockout of GPX8 gene in single clone was verified by DNA sequencing and Western blot.
Cells and cell culture
SCC-9 and GPX8-KO SCC-9 cells were cultured in Dulbecco’s Modified Eagle’s Medium (DMEM) supplemented with 10% FBS (GIBCO, Australia), 100 U/mL penicillin G, and 100 μg/mL streptomycin. All cells were cultured under humidified conditions with 5% CO2 at 37°C. About 5 × 107 cells were collected for MeRIP-seq and RNA sequencing (RNA-seq).
MeRIP-seq and bioinformatics analysis
Transcriptome-wide methylated RNA immunoprecipitation sequencing (MeRIP-seq) and RNA sequencing (RNA-seq) were performed as previously described [Citation35–37]. Briefly, the total RNA was extracted using TRIzol reagent (Invitrogen, Carlsbad, CA, USA). PolyA RNA was purified using Dynabeads Oligo (dT)25–61005 (Thermo Fisher, CA, USA) and cleaved into about 100nt fragments using Magnesium RNA Fragmentation Module (NEB, USA). One portion of the RNA fragments was used as input and the other portion was immunoprecipitated with m6A-specific antibody (Synaptic Systems, Germany) to enrich m6A-methylated RNA fragments. The RNA sequence library was prepared and purified, and the paired-end sequencing (PE150) of libraries was performed on Illumina Novaseq™ 6000 platform (LC-Bio Technology CO., Ltd., Hangzhou, China), following the manufacturer’s protocol.
Low quality reads and adaptors were removed from the raw data using fastp tool (https://github.com/OpenGene/fastp) [Citation38]. The quality of IP and input sequences was verified using FastQC (https://www.bioinformatics.babraham.ac.uk/projects/fastqc/) and RseQC (http://rseqc.sourceforge.net/) [Citation39,Citation40]. HISAT2 (http://daehwankimlab.github.io/hisat2) was used to map clean reads to the reference genome Homo sapiens (Version: v101) [Citation41]. m6A peak calling and differentially methylated peaks were analysed by exomePeak2 of R package (https://bioconductor.org/packages/release/bioc/html/exomePeak2.html) [Citation42], and R package ANNOVAR (http://www.openbioinformatics.org/annovar/) [Citation43] was used to annotate the peaks. MEME (http://meme-suite.org) [Citation44] and HOMER (http://homer.ucsd.edu/homer/motif) were used for motif finding. StringTie (https://ccb.jhu.edu/software/stringtie) [Citation45] was used to analyse the expression level of transcripts and genes through calculating FPKM, and the differentially expressed transcripts and genes were identified using R package edgeR (https://bioconductor.org/packages/edgeR) [Citation46]. The differentially methylated coding genes and differentially expressed genes were analysed by Gene Ontology (GO) and Kyoto Encyclopaedia of Genes and Genomes (KEGG), respectively [Citation47].
Real-time reverse transcription-polymerase chain reaction (RT-PCR)
Total RNA was isolated using TRIzol reagent (Invitrogen, Carlsbad, CA, USA) according to the manufacturer’s instructions. RNA was treated with gDNA Eraser to remove genomic DNA and reverse-transcribed using PrimeScript RT Enzyme Mix I (PrimeScriptTM RT reagent Kit with gDNA Eraser, TaKaRa, Shiga, Japan) and random hexamer primers. The RT-PCR was carried out in 20 μL reaction volumes in triplicate with SYBR® Premix Ex Taq II (Tli RNaseH Plus, TaKaRa, Shiga, Japan) and the ABI PRISM®7900 system (ABI). The threshold cycles and relative expression levels were calculated with 2−ΔΔCt. The primers used to detect 19 major m6A regulatory genes have been described in a previous study [Citation37]. To investigate the effects of oxidative stress, cells were treated with 100 μM hydrogen peroxide for 12 h or 24 h before RNA isolation.
ROS assay
2‘,7’-dichlorofluorescein diacetate (DCFDA) cellular ROS detection assay kit (ab113851, Abcam, USA) was used to detect the ROS level in wild-type and GPX8-KO SCC-9 cells. The cells were seeded in 35 mm cell culture dishes and maintained in the DMEM medium for 24 h. Cells were washed twice with 1× buffer and incubated with 200 μl 25 μM DCFDA working solution at 37 ºC in the dark for 45 min. After two washings, the cells were examined under a fluorescence microscope. For flow cytometry analysis, cells were trypsinized and incubated with 25 μM DCFDA at 37°C for 30 min. After washing twice, the cells were suspended in PBS and analysed by CytoFlex (Beckman Courtier) at 488 nm.
Statistical analysis
According to the criteria |log2FC| ≥ 1 and P < 0.05, the differentially methylated peaks of MeRIP-seq and differentially expressed genes of RNA-seq were determined using exomePeak2 [Citation42] and edgeR [Citation46] in R package, respectively. The GraphPad Prism 8 was used to analyse RT-PCR data, and two tailed unpaired Student's t-test was used to calculate P values.
Results
GPX8 deficiency increased the production of cellular ROS
In order to explore the role of GPX8 in maintaining cell redox homoeostasis, we designed two gdRNAs and edited GPX8 gene in SCC-9 oral cancer cells using CRISPR-Cas9 technology. Single colonies of GPX8-edited cells were screened and sequenced. In a monoclonal cell line, a guanine nucleotide was inserted at codon 5 of the coding sequence of GPX8, causing a frameshift mutation in the open reading frame (ORF) of GPX8 and premature termination of translation at codon 43 (). Western blot conformed that there was no GPX8 proteins in the GPX8-edited cell line, which was named GPX8-KO SCC-9 cells (). When the cells were stained with DCFDA, it was found that the ROS level in GPX8-deficient SCC-9 cells was significantly higher than that in wild-type SCC-9 cells (), indicating that the lack of GPX8 led to oxidative stress in cells.
Figure 1. The deletion of GPX8 induced oxidative stress in cells. (a) Editing of the GPX8 gene resulted in the insertion of a guanine nucleotide at codon 5 of the GPX8 coding sequence. (b) The edited GPX8 gene caused a frameshift mutation and premature termination of translation at codon 43. (c) GPX8 expression was not detected in GPX8-KO SCC-9 cells. (d, e) Compared with wild-type SCC-9 cells, GPX8-deficient SCC-9 cells increased ROS production. **P < 0.01.

Loss of GPX8 reprogrammed m6A epitranscriptome in oral cancer cells
To explore the effect of oxidative stress induced by GPX8 deletion on the m6A modification, we performed MeRIP-seq and RNA-seq of GPX8-deficient SCC-9 and SCC-9 cells (Table S1). After removing the reads with adapters, low-quality bases, and undetermined bases in the raw data, more than 90% of the clean reads from the IP and input samples can be mapped to gene exons in the reference genome. A total of 45,108 and 43,608 m6A peaks were identified in SCC-9 and GPX8-deficient SCC-9 cells, respectively. The two cell lines shared similar characteristics in the distribution and density of m6A peaks that were highly enriched in 3`UTR and stop codon regions (). Typical conserved m6A motifs were enriched in some m6A peak sequences ().
Figure 2. Distribution of m6A peaks across the mRNA transcripts and the representative m6A motifs enriched. (a) The m6A peaks were highly enriched in 3`UTR and stop codon regions. (b) The m6A motifs with typical conserved sequence in SCC-9 cells. (c) The m6A motifs with typical conserved sequence in GPX8-KO SCC-9 cells. NC, SCC-9 cells; KO, GPX8-KO SCC-9 cells.
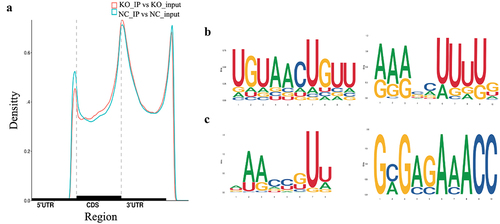
Then, we compared the difference of m6A peaks between two cell lines and found 1,279 hyper-methylated and 2,287 hypo-methylated m6A peaks in GPX8-KO SCC-9 cells compared with SCC-9 cells (|log2FC|≥1.0 and P < 0.05). The 20 genes with the most significant m6A modification change are listed in . The GO function and KEGG pathway enrichment of the differentially methylated mRNA was performed to explore the biological significance of m6A modification. These genes were enriched into GO terms such as protein binding and KEGG pathways such as ubiquitin mediated proteolysis (). Further analysis indicated that many genes listed under GO term of cellular response to oxidative stress had m6A modification change ().
Figure 3. GO function and KEGG pathway enrichment of differentially methylated mRNA. (a) Top 20 significantly enriched GO terms. (b) Top 20 significantly enriched KEGG pathways.
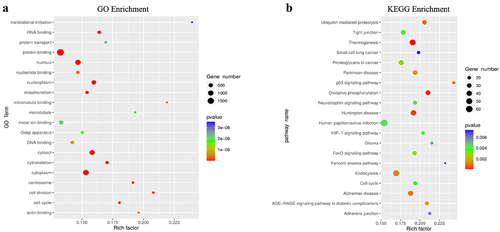
Table 1. The 20 coding genes with the most significantly altered m6A peaks in GPX8-KO SCC-9 cells compared with SCC-9 cells.
Table 2. Differential expression of oxidative stress-related genes in GPX8-KO SCC-9 cells.
Change of m6A modification in GPX8-KO SCC-9 cells may regulate gene expression
It seems that the loss of GPX8 has no significant effect on the transcriptional activity of the whole cell (). When RNA-seq data were analysed for differentially expressed genes, 1,123 genes were significantly upregulated and 913 genes were significantly downregulated genes in GPX8-KO SCC-9 cells (|log2FC|≥1.0 and P < 0.05; ). Among them, 28 genes are involved in the cellular response to oxidative stress (). The top 20 and top 100 genes differentially expressed between GPX8-KO SCC-9 cells and SCC-9 cells are shown in and Fig S1.
Figure 4. Transcriptional activity of the whole cell and differentially expressed genes. (a) GPX8-KO SCC-9 and SCC-9 cells have similar overall transcriptional activity. (b) Volcano plots of differentially expressed genes between GPX8-KO SCC-9 and SCC-9 cells. |log2FC|≥1.0 and P < 0.05. KO, GPX8-KO SCC-9 cells; NC, SCC-9 cells.
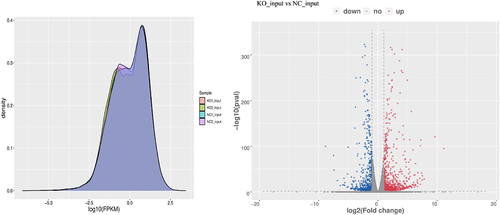
Table 3. The top 20 differentially expressed coding genes in GPX8-KO SCC-9 cells compared with SCC-9 cells.
We further jointly analysed the MeRIP-seq and RNA-seq data and identified 509 upregulated and 453 downregulated mRNAs in GPX8-KO SCC-9 cells. These genes were either m6A hyper-methylated or m6A hypo-methylated (, ). Even a single mRNA, such as serpin family E member 1 (SERPINE1) or DAB adaptor protein 2 (DAB2), can be modified by hypermethylation and hypomethylation at different sites at the same time (). The data indicated that the expression changes of many genes in GPX8-KO SCC-9 cells appeared to be related to the m6A modification, although the regulation of mRNA m6A methylation on its own expression needed further evaluation. The GO function and KEGG pathway enrichment of these genes were further analysed ().
Figure 5. Distribution of differentially expressed genes with differential m6A peaks. Hyper-up, m6A peak upregulated and mRNA expression upregulated; Hyper-down, m6A peak upregulated and mRNA expression downregulated; Hypo-up, m6A peak downregulated and mRNA expression upregulated; Hypo-down, m6A peak downregulated and mRNA expression downregulated.
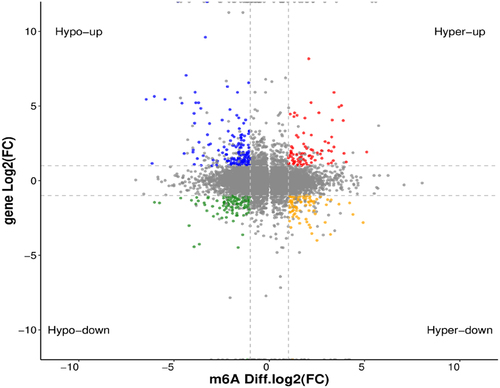
Figure 6. GO function and KEGG pathway enrichment of differentially expressed genes with differential m6A peaks. (A) Top 20 significantly enriched GO terms. (B) Top 20 significantly enriched KEGG pathways.
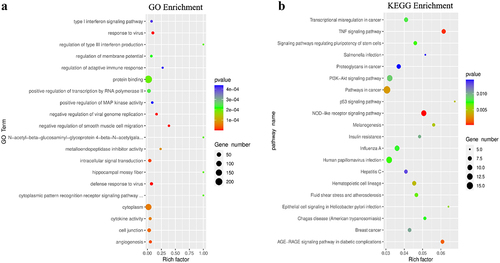
Table 4. The first 10 differentially expressed genes with differential m6A peaks in GPX8-KO SCC-9 cells compared with SCC-9 cells.
GPX8 deficiency–induced oxidative stress dysregulated expression of m6A regulatory genes
Since RNA m6A modification is dynamically regulated by m6A regulatory genes, such as m6A writer and eraser, the expression of these genes was further investigated. By analysing our sequencing data, we found that insulin-like growth factor 2 mRNA binding protein 2 (IGF2BP2) (log2FC, 1.32) and IGF2BP3 (log2FC, 3.49) were upregulated and fat mass and obesity-associated protein (FTO) (log2FC, −1.26) was downregulated in GPX8-KO SCC-9 cells compared with SCC-9 cells (p < 0.05). These were confirmed when the expression of 19 m6A regulatory genes was further measured by RT-PCR (P < 0.01, ). In addition, the expression of RNA binding motif protein 15 (RBM15), Vir-Like m6A methyltransferase associated (VIRMA), zinc finger CCCH-type containing 13 (ZC3H13), and YTH domain-containing 2 (YTHDC2) decreased significantly in GPX8-KO SCC-9 cells (P < 0.01). To some extent, methyltransferase like-3 (METTL3), RNA binding motif protein 15B (RBM15B), heterogeneous nuclear ribonucleoprotein A2B1 (HNRNPA2B1) and heterogeneous nuclear ribonucleoprotein C (HNRNPC) were downregulated in GPX8 deficient cells (0.01< P < 0.05).
Figure 7. Expression of m6A regulatory genes detected by real-time RT-PCR. (A) Expression of m6A regulators in GPX8-KO SCC-9 and SCC-9 cells. (B) Expression of m6A regulators after hydrogen peroxide treatment within the indicated time. All bars indicate the mean ± SD of three independent experiments. *P < 0.05, **P < 0.01.
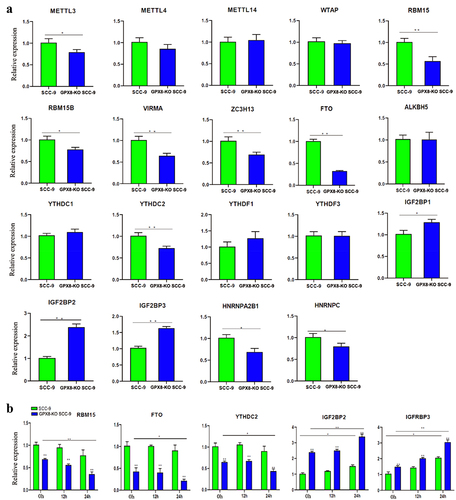
To study the effects of oxidative stress on the expression of m6A regulatory genes, the cells were treated with hydrogen peroxide for 12 h or 24 h, and several m6A regulatory genes were detected by RT-PCR. We found that the expression of RBM15, IGF2BP2, and IGF2BP3 further decreased or increased in GPX8-KO SCC-9 cells after 24 h of treatment (P < 0.01, ). The expression of FTO and YTHDC2 was also downregulated to some extent (0.01< P < 0.05). Hydrogen peroxide treatment induced relatively smaller changes in the expression of these genes in SCC-9 cells.
Discussion
GPX8 is a recently discovered member of the GPX family, which is upregulated in many tumours. The significance of its antioxidant capacity in tumours and its cross-talk with epigenetic modification of m6A has not been well understood. In this study, we analysed the transcriptome-wide m6A modification through MeRIP-seq in GPX8-deficient oral cancer cells and investigated the expression of major m6A regulatory genes by RT-PCR. We found that the loss of GPX8 resulted in over production of ROS in cells. Thousands of genes in GPX8-KO SCC-9 cells, including nearly half of the differentially expressed genes, have changed their m6A modification status, and many of them are involved in the cell’s response to oxidative stress. GPX8 deficiency also regulated the expression of several m6A regulatory genes, and hydrogen peroxide stimulation led to further changes in the expression of these genes.
We can see the m6A peaks of both hyper-methylation and hypo-methylation in GPX8-deficient cells, and the m6A peak distribution of GPX8-deficient cells and wild-type cells is similar, which indicates that the lack of GPX8 will not affect the overall m6A methylation level and m6A modification characteristics. Changes in m6A modification may vary by gene. Hyper- or hypo-methylation can occur at multiple sites of a single transcript.
We found that in GPX8-KO SCC-9 cells, many genes changed their m6A modification, and some of them showed differential expression, which needs to be evaluated in the future. First, we need to confirm the change of m6A modification through various experimental methods. It is reported that some genes identified here are regulated by m6A methylation. For example, m6A methylation of eukaryotic translation initiation factor 3 subunit C (EIF3C) mRNA dependent on WTAP facilitates prostate cancer metastasis [Citation48]. Secondly, we are not sure whether the difference of m6A modification will lead to the change of gene expression level. Among 2036 (1123 + 913) differentially expressed genes in this study, 962 (509 + 453) genes changed the state of m6A modification, which supports the regulatory effect of m6A modification on gene expression. The mechanism by which some key genes regulate their expression through m6A modification is worthy studying.
The deletion of GPX8 may regulate gene expression by affecting mRNA m6A modification. In turn, these genes may function to adapt to cellular oxidative stress, which is caused by the loss of GPX8. We must point out that many genes with differential m6A modification or differential expression in GPX8-KO SCC-9 cells do not seem to be directly related to oxidative stress. Their potential association with oxidative stress and the significance of these changes in GPX8 deficient cells remain to be clarified. However, we found that 28 genes () related to the cellular response to oxidative stress were differentially expressed in GPX8-KO SCC-9 cells. Half of them changed their m6A levels. This indicates that GPX8 loss mediated oxidative stress can modulate the expression of genes, including those regulating redox homoeostasis.
The significance of cross-talk between m6A modification and oxidative stress is an important issue in many biological processes [Citation19]. m6A modifications can modulate cellular ROS levels, and in turn, ROS signalling also plays a regulatory role in m6A modifications. ROS significantly induced global mRNA m6A levels by regulating ALKBH5 post-translational modification [Citation49]. Hypoxia can induce ROS production [Citation50]. ALKBH5 reduced the overall m6A level in response of hypoxia [Citation51]. Hypoxia-induced breast cancer stem cell phenotype through ALKBH5-mediated m6A demethylation of NANOG mRNA [Citation52]. YTHDF1 played an important role in hypoxic adaptation and pathogenesis of non-small cell lung cancer [Citation53]. Hypoxia can induce SUMOylation of YTHDF2, which promotes mRNA degradation and cancer progression by increasing its binding affinity with m6A modified mRNAs [Citation29]. Hypoxia blocked ferroptosis of hepatocellular carcinoma via suppression of METTL14 triggered YTHDF2-dependent silencing of SLC7A11 [Citation54]. Some studies also described the role of m6A modification in regulating the redox balance of cells. For examples, several m6A-related lncRNAs are associated with oxidative stress in oral cancer and can predict the prognosis of oral squamous cell carcinoma [Citation55]. The demethylase FTO promoted oxidative stress and apoptosis of ovarian cancer cells to inhibit tumour growth in nude mice [Citation56]. It can also inhibit oxidative stress by mediating m6A demethylation of Nrf2 to reduce cerebral ischaemia/reperfusion injury [Citation57]. YTHDC2 inhibited lung adenocarcinoma tumorigenesis by suppressing SLC7A11-dependent antioxidant function [Citation58].
In fact, it has not been reported that the antioxidant activity of GPX8 is related to m6A modification. In this study, based on the following findings, we concluded that the oxidative stress caused by GPX8 deficiency changed m6A modification. First, GPX8 deficiency increased ROS level in cells. Next, the hydrogen peroxide stimulation in GPX8-KO SCC-9 cells did change the expression of several m6A regulatory genes (). This indirectly supports the change of m6A modification mediated by oxidative stress, but the regulatory effect of these genes on m6A modification is still unclear. Finally, the global change of m6A modification was observed in GPX8-KO cells. In the future, it will be interesting to explore the direct correlation between oxidative stress induced by GPX8 deficiency and m6A modification. It should be noted that the biological function and pathological significance of GPX8 are largely attributed to its antioxidant capacity. So far, there is no evidence that GPX8 acts as an m6A regulatory gene.
In conclusion, our results suggest that GPX8 lack-induced oxidative stress reprograms m6A epitranscriptome of oral cancer cells. It is supposed that oxidative stress modulates the expression of m6A regulatory genes, which in turn leads to transcriptome-wide changes of m6A modification, and consequentially affects the expression and function of downstream genes to adapt to oxidative stress. The study highlights the potential value of GPX8 and the related m6A regulatory or regulated genes as novel targets for antioxidant intervention in cancer treatment.
Author contributions
DY, WZ, and SC contributed to the conception and design of the study. XC, LY, LZ, LC, YH, CW, and JW collected samples and performed experiments. All authors participated in the writing of the manuscript. All authors have read and agreed to the published version of the manuscript.
Supplemental Material
Download MS Word (276.1 KB)Disclosure statement
No potential conflict of interest was reported by the authors.
Data availability statement
All data generated or analysed during this study are available from the corresponding author upon reasonable request (https://www.ncbi.nlm.nih.gov/geo/query/acc.cgi?) with GEO accession number GSE224718.
Supplementary material
Supplemental data for this article can be accessed online at https://doi.org/10.1080/15592294.2023.2208707.
Additional information
Funding
References
- Buday K, Conrad M. Emerging roles for non-selenium containing ER-resident glutathione peroxidases in cell signaling and disease. Biol Chem. 2021;402(3):271–15.
- Ramming T, Hansen HG, Nagata K, et al. Gpx8 peroxidase prevents leakage of H2O2 from the endoplasmic reticulum. Free Radical Bio Med. 2014;70:106–116.
- Morikawa K, Gouttenoire J, Hernandez C, et al. Quantitative proteomics identifies the membrane-associated peroxidase GPx8 as a cellular substrate of the hepatitis C virus NS3-4A protease. Hepatology. 2014;59(2):423–433. DOI:10.1002/hep.26671
- Yoboue ED, Rimessi A, Anelli T, et al. Regulation of calcium fluxes by GPX8, a Type-II transmembrane peroxidase enriched at the mitochondria-associated endoplasmic reticulum membrane. Antioxid Redox Signal. 2017;27(9):583–595. DOI:10.1089/ars.2016.6866
- Mehmeti I, Lortz S, Avezov E, et al. ER-resident antioxidative GPx7 and GPx8 enzyme isoforms protect insulin-secreting INS-1E beta-cells against lipotoxicity by improving the ER antioxidative capacity. Free Radic Biol Med. 2017;112:121–130.
- Hsu JL, Chou JW, Chen TF, et al. Glutathione peroxidase 8 negatively regulates caspase-4/11 to protect against colitis. EMBO Mol Med. 2020;12(1):e9386. DOI:10.15252/emmm.201809386
- Bosello Travain V, Miotto G, Vuckovic AM, et al. Lack of glutathione peroxidase-8 in the ER impacts on lipid composition of HeLa cells microsomal membranes. Free Radic Biol Med. 2020;147:80–89.
- Zhou Y, Zhang D, Liu B, et al. Bioinformatic identification of key genes and molecular pathways in the spermatogenic process of cryptorchidism. Genes Dis. 2019;6(4):431–440. DOI:10.1016/j.gendis.2018.11.002
- Wu JH, Wang X, Wang N, et al. Identification of novel antioxidant gene signature to predict the prognosis of patients with gastric cancer. World J Surg Oncol. 2021;19(1):219. DOI:10.1186/s12957-021-02328-w
- Zhang XX, Zhan DK, YY L, et al. Glutathione peroxidase 8 as a prognostic biomarker of gastric cancer: an analysis of the cancer genome atlas (TCGA) data. Med Sci Monit. 2020;26:e921775.
- Jagust P, Alcala S, Sainz B, et al. Glutathione metabolism is essential for self-renewal and chemoresistance of pancreatic cancer stem cells. World J Stem Cells. 2020;12(11):1410–1428. DOI:10.4252/wjsc.v12.i11.1410
- Jia Y, Dai J, Zeng Z. Potential relationship between the selenoproteome and cancer. Mol Clin Oncol. 2020;13(6):83.
- Zhang J, Liu Y, Guo Y, et al. GPX8 promotes migration and invasion by regulating epithelial characteristics in non-small cell lung cancer. Thorac Cancer. 2020;11(11):3299–3308. DOI:10.1111/1759-7714.13671
- Chen H, Xu L, Shan ZL, et al. GPX8 is transcriptionally regulated by FOXC1 and promotes the growth of gastric cancer cells through activating the Wnt signaling pathway. Cancer Cell Int. 2020;20(1):596. DOI:10.1186/s12935-020-01692-z
- Lee HA, Chu KB, Moon EK, et al. Glutathione peroxidase 8 suppression by histone deacetylase inhibitors enhances endoplasmic reticulum stress and cell death by oxidative stress in hepatocellular carcinoma cells. Antioxidants (Basel). 2021;10(10):1503. DOI:10.3390/antiox10101503
- Zahra KF, Lefter R, Ali A, et al. The Involvement of the oxidative stress status in cancer pathology: a double view on the role of the antioxidants. Oxid Med Cell Longev. 2021;2021:9965916.
- Azmanova M, Pitto-Barry A. Oxidative stress in cancer therapy: friend or enemy? Chembiochem. 2022;23(10):e202100641.
- Hayes JD, Dinkova-Kostova AT, Tew KD. Oxidative stress in cancer. Cancer Cell. 2020;38(2):167–197.
- Yang B, Chen Q. Cross-talk between oxidative stress and m(6)A RNA methylation in cancer. Oxid Med Cell Longev. 2021;2021:6545728.
- Xue D, Zhou X, Qiu J. Emerging role of NRF2 in ROS-mediated tumor chemoresistance. Biomed Pharmacother. 2020;131:110676.
- Li D, Song C, Zhang J, et al. ROS and iron homeostasis dependent ferroptosis play a vital role in 5-Fluorouracil induced cardiotoxicity in vitro and in vivo. Toxicology. 2022;468:153113.
- Xin X, Wen T, Gong LB, et al. Inhibition of FEN1 increases arsenic trioxide-induced ros accumulation and cell death: novel therapeutic potential for triple negative breast cancer. Front Oncol. 2020;10:425.
- Zhong G, Wan F, Ning Z, et al. The protective role of autophagy against arsenic trioxide-induced cytotoxicity and ROS-dependent pyroptosis in NCTC-1469 cells. J Inorg Biochem. 2021;217:111396.
- Lei G, Zhang Y, Koppula P, et al. The role of ferroptosis in ionizing radiation-induced cell death and tumor suppression. Cell Res. 2020;30(2):146–162. DOI:10.1038/s41422-019-0263-3
- Yalcin Y, Tekin IO, Tigli Aydin RS. Ionizing radiation induced DNA damage via ROS production in nano ozonized oil treated B-16 melanoma and OV-90 ovarian cells. Biochem Biophys Res Commun. 2022;615:143–149.
- Havaki S, Kotsinas A, Chronopoulos E, et al. The role of oxidative DNA damage in radiation induced bystander effect. Cancer Lett. 2015;356(1):43–51. DOI:10.1016/j.canlet.2014.01.023
- Liu R, Bian Y, Liu L, et al. Molecular pathways associated with oxidative stress and their potential applications in radiotherapy. Int J Mol Med. 2022;49(5):65. DOI:10.3892/ijmm.2022.5121
- Breau M, Houssaini A, Lipskaia L, et al. The antioxidant N-acetylcysteine protects from lung emphysema but induces lung adenocarcinoma in mice. JCI Insight. 2019;4(19):e127647. DOI:10.1172/jci.insight.127647
- Hou G, Zhao X, Li L, et al. Sumoylation of YTHDF2 promotes mRNA degradation and cancer progression by increasing its binding affinity with m6A-modified mRnas. Nucleic Acids Res. 2021;49(5):2859–2877. DOI:10.1093/nar/gkab065
- Zhao T, Li X, Sun D, et al. Oxidative stress: one potential factor for arsenite-induced increase of N(6)-methyladenosine in human keratinocytes. Environ Toxicol Pharmacol. 2019;69:95–103.
- Wang J, Ishfaq M, Xu L, et al. Mettl3/m(6)a/miRNA-873-5p attenuated oxidative stress and apoptosis in colistin-induced kidney injury by modulating Keap1/Nrf2 pathway. Front Pharmacol. 2019;10:517.
- Fu Y, Zhuang X. M(6)a-binding YTHDF proteins promote stress granule formation. Nat Chem Biol. 2020;16(9):955–963.
- Chen X, Yi C, Yang MJ, et al. Metabolomics study reveals the potential evidence of metabolic reprogramming towards the Warburg effect in precancerous lesions. J Cancer. 2021;12(5):1563–1574. DOI:10.7150/jca.54252
- Chang SE, Foster S, Betts D, et al. DOK, a cell line established from human dysplastic oral mucosa, shows a partially transformed non-malignant phenotype. Int J Cancer. 1992;52(6):896–902. DOI:10.1002/ijc.2910520612
- Zhang Z, Wang Q, Zhang M, et al. Comprehensive analysis of the transcriptome-wide m6A methylome in colorectal cancer by MeRIP sequencing. Epigenetics. 2021;16(4):425–435. DOI:10.1080/15592294.2020.1805684
- Han Z, Yang B, Wang Q, et al. Comprehensive analysis of the transcriptome-wide m(6)A methylome in invasive malignant pleomorphic adenoma. Cancer Cell Int. 2021;21(1):142. DOI:10.1186/s12935-021-01839-6
- Sasada S, Miyata Y, Tsutani Y, et al. Metabolomic analysis of dynamic response and drug resistance of gastric cancer cells to 5-fluorouracil. Oncol Rep. 2013;29(3):925–931. DOI:10.3892/or.2012.2182
- Chen S, Zhou Y, Chen Y, et al. Fastp: an ultra-fast all-in-one FASTQ preprocessor. Bioinformatics. 2018;34(17):i884–90. DOI:10.1093/bioinformatics/bty560
- de Sena Brandine G, Smith AD. Falco: high-speed FastQC emulation for quality control of sequencing data. F1000Res. 2019;8:1874.
- Wang L, Wang S, Li W. RSeQC: quality control of RNA-seq experiments. Bioinformatics. 2012;28(16):2184–2185.
- Kim D, Langmead B, Salzberg SL. HISAT: a fast spliced aligner with low memory requirements. Nat Methods. 2015;12(4):357–360.
- Meng J, Lu Z, Liu H, et al. A protocol for RNA methylation differential analysis with MeRIP-Seq data and exomePeak R/Bioconductor package. Methods. 2014;69(3):274–281. DOI:10.1016/j.ymeth.2014.06.008
- Wang K, Li M, Hakonarson H. ANNOVAR: functional annotation of genetic variants from high-throughput sequencing data. Nucleic Acids Res. 2010;38(16):e164.
- Bailey TL, Boden M, Buske FA, et al. MEME SUITE: tools for motif discovery and searching. Nucleic Acids Res. 2009;37(Web Server):W202–8. DOI:10.1093/nar/gkp335
- Pertea M, Pertea GM, Antonescu CM, et al. StringTie enables improved reconstruction of a transcriptome from RNA-seq reads. Nat Biotechnol. 2015;33(3):290–295. DOI:10.1038/nbt.3122
- Robinson MD, McCarthy DJ, Smyth GK. edgeR: a Bioconductor package for differential expression analysis of digital gene expression data. Bioinformatics. 2010;26(1):139–140.
- Huang da W, Sherman BT, RA L. Systematic and integrative analysis of large gene lists using DAVID bioinformatics resources. Nat Protoc. 2009;4(1):44–57.
- Ding L, Wang R, Zheng Q, et al. circPDE5A regulates prostate cancer metastasis via controlling WTAP-dependent N6-methyladenisine methylation of EIF3C mRNA. J Exp Clin Cancer Res. 2022;41(1):187. DOI:10.1186/s13046-022-02391-5
- Yu F, Wei J, Cui X, et al. Post-translational modification of RNA m6A demethylase ALKBH5 regulates ROS-induced DNA damage response. Nucleic Acids Res. 2021;49(10):5779–5797. DOI:10.1093/nar/gkab415
- Fuhrmann DC, Brune B. Mitochondrial composition and function under the control of hypoxia. Redox Biol. 2017;12:208–215.
- Wang YJ, Yang B, Lai Q, et al. Reprogramming of m 6 a epitranscriptome is crucial for shaping of transcriptome and proteome in response to hypoxia. RNA Biol. 2021;18(1):131–143. DOI:10.1080/15476286.2020.1804697
- Zhang C, Samanta D, Lu H, et al. Hypoxia induces the breast cancer stem cell phenotype by HIF-dependent and ALKBH5-mediated m 6 A-demethylation of NANOG mRNA. Proc Natl Acad Sci U S A. 2016;113(14):E2047–56. DOI:10.1073/pnas.1602883113
- Shi Y, Fan S, Wu M, et al. YTHDF1 links hypoxia adaptation and non-small cell lung cancer progression. Nat Commun. 2019;10(1):4892. DOI:10.1038/s41467-019-12801-6
- Fan Z, Yang G, Zhang W, et al. Hypoxia blocks ferroptosis of hepatocellular carcinoma via suppression of METTL14 triggered YTHDF2-dependent silencing of SLC7A11. J Cell Mol Med. 2021;25(21):10197–10212. DOI:10.1111/jcmm.16957
- Shan L, Lu Y, Song Y, et al. Identification of nine m6A-related long noncoding RNAs as prognostic signatures associated with oxidative stress in oral cancer based on data from the cancer genome atlas. Oxid Med Cell Longev. 2022;2022:9529814.
- Wu J, Wang X, Li X. N6-methyladenosine methylation regulator FTO promotes oxidative stress and induces cell apoptosis in ovarian cancer. Epigenomics. 2022;14(23):1509–1522.
- Hou L, Li S, Li S, et al. FTO inhibits oxidative stress by mediating m6A demethylation of Nrf2 to alleviate cerebral ischemia/reperfusion injury. J Physiol Biochem. 2023;79(1):133–146. DOI:10.1007/s13105-022-00929-x
- Ma L, Chen T, Zhang X, et al. The m(6)A reader YTHDC2 inhibits lung adenocarcinoma tumorigenesis by suppressing SLC7A11-dependent antioxidant function. Redox Biol. 2021;38:101801.