ABSTRACT
Abscisic acid (ABA)-induced stomatal closure can improve drought tolerance in higher plants. However, the relationship between ABA-related ion flux and improved drought resistance in the roots of woody plants is unclear. To investigate this relationship, we employed a noninvasive micro-test technique (NMT) to detect potassium (K+) flux in Catalpa fargesii and C. fargesii f. duclouxii after treatment with polyethylene glycol (PEG) and ABA. PEG treatment slightly increased the free proline content in both Catalpa species. However, simultaneous treatment with ABA and PEG resulted in a large increase in free proline content. Treatment with PEG led to a significant increase in K+ efflux, and both ABA and tetraethylammonium (TEA, a K+ channel inhibitor) blocked this efflux under short-term (1 d) and long-term (7 d) drought conditions. Furthermore, we detected SKOR (stelar K+ outward-rectifying channel) gene expression in roots, and the results showed that PEG significantly increased SKOR expression in C. fargesii f. duclouxii, but SKOR expression was inhibited by ABA in Catalpa fargesii. These findings indicate that ABA improves drought tolerance by inhibiting K+ efflux in Catalpa, but distinct ABA response patterns exist. Drought-tolerant species have better potassium retention are dependent on ABA, and can accumulate more proline than other species. SKOR is also ABA-dependent and sensitive to ABA, and K+ flux is a target of the ABA-mediated drought response.
Introduction
Drought is defined as severe water deficit or osmotic stress that leads to a large decline in the yield of higher plants. Since trees have a long-life cycle, they may be exposed to multiple short- and long-term droughts throughout their lifetime.Citation1 During drought, rapid accumulation of abscisic acid (ABA) regulates turgor pressure through osmotic adjustment,Citation2 and ABA-dependent and -independent signal transduction pathways are activated and deliver the signals above ground.Citation3-Citation6
Catalpa is a beautiful native tree species in China. It has many advantages, including fast growth, high quality wood, and strong adaptability. However, different varieties of Catalpa (for example Catalpa fargesii and C. fargesii f. duclouxii) show different levels of drought-resistance in different environments.Citation7,Citation8 Drought affects the osmotic potential of plant cells by reducing their water content. Therefore, for plants to survive drought, their osmotic potential must be adjusted by regulating cellular levels of organic solutes (e.g., amino acids) and inorganic ions such as potassium (K+).Citation9 ABA, which is synthesized in roots and transported above ground, acts as a stress signal and can improve drought resistance during low-water conditions.Citation10-Citation13 Because of the importance of K+ to proper cell functioning and the role of ABA in drought resistance, the signaling pathways involved in the regulation of cellular K+ content by ABA are of interest to many researchers.Citation14-Citation16 Previous studies found that K+ channel activity facilitated the adaptation of maize roots to dry soils, but the role of ABA in regulating K+ flux was not clarified.Citation17,Citation18 ABA inhibits K+ uptake during the germination of barley seeds, indicating that ABA regulates K+ channels in the roots of this species.Citation19 Moreover, K+ channels may target ABA-perception sites in Arabidopsis thaliana suspension cells.Citation20 However, the mechanisms by which drought resistance is improved by ABA-mediated changes in K+ channels are unclear in many plants, especially trees.Citation18
The aim of the present study was to identify the physiological mechanisms of ABA-improved drought tolerance in woody plants. We employed a noninvasive micro-test technique (NMT) to detect the K+ flux in Catalpa fargesii and C. fargesii f. duclouxii after treatment with PEG and ABA. ABA was found to enhance drought tolerance by inhibiting the K+ efflux that was activated by SKOR gene expression. Catalpa fargesii was more drought-resistant than C. fargesii f. duclouxii and showed both ABA-dependent and ABA-independent patterns of K+ flux.
Materials and methods
Plant materials and growth conditions
Catalpa fargesii and Catalpa fargesii duclouxii were collected in northwestern China (Gansu, Shaanxi Province) and in southwestern China (Guizhou, Yunnan, Province), respectively. Seeds (n = 400) of each species were sown in culture dishes and placed in an illumination box (10/14-h day/night cycle at 28/22°C with irradiance of 180 μmol m−2s−1. The seeds were watered daily to ensure that the bottom of the culture dish remained wet. Seeds germinated after 4 d and were transplanted into plastic pots (12-cm diameter) with soil and cultured in the greenhouse at the Chinese Academy of Forestry. Seedlings (n = 36) of each species were washed with water to remove soil and cultured in four plastic pots (n = 9 per pot) each containing 6 L of growth solution (GS) including CaCl2 34 mg/L, KCl 1.68 mg/L, MgSO4 10.5 mg/L, NaCl 32.2 mg/L, and CuSO4 5 μg/L. The GS in the pots was continuously aerated and was replenished every 2 d. Greenhouse conditions included a temperature of 28–32°C, a 13-h photoperiod (5:30 AM-6:30 PM), and approximately 200 μmol m−2s−1 photosynthetically active radiation.
PEG treatments
After 7 d of hydroponic growth, seedlings of each species were transferred to four plastic pots for treatment with different concentrations of PEG-6000. Four PEG-6000 concentrations (0, 100, 150, and 225 g/L) were administered (n = 3 plants for each concentration).
PEG and ABA treatment
Twenty-four plants of each species were transplanted into four plastic pots (n = 6 plants per pot). Each pot was treated with one of the following conditions (treatment names in parentheses): GS (CK, control); GS + 225 g/L PEG-6000 (PEG); GS + 100 μg/L ABA (ABA); or GS + 225 g/L PEG-6000 and 100 μg/L ABA (PEG + ABA). The osmotic pressure, Pn, and K+ flux of plant roots was determined after 1 (short-term) and 7 d (long-term).
Measurement of vapor pressure
The vapor pressure of the culture solution was measured using a vapor pressure osmometer (Vapro 5600, Wescor Inc., USA) at 25°C.
Measurement of proline
The free proline content of plants under long-term treatment (7 d) was determined using the approach described by Bates et al.Citation21 with modifications. We added 5.0 ml 3% (w/v) sulfosalicylic acid to 20-mL glass tubes containing 0.5 g fresh leaves or roots, plugged the tubes, and boiled the mixtures in a water bath at 100°C for 10 min. After cooling, the mixtures were filtered into plastic tubes. Extracts (2 mL) of the filtrate were mixed with 2 mL glacial acetic acid and 2 mL ninhydrin in 20-ml plugged glass tubes and boiled at 100°C for 30 min. After cooling the mixtures to room temperature, 4 mL toluene was added to each tube. The tubes were shaken for 3 min and left to stand for 2 min, after which chromophores were separated and centrifuged at 3000 rpm for 5 min at 25°C. The absorbance of the chromophores was determined using a spectrophotometer (UV-2000, Unico Instrument Co., Shanghai, China) at a wavelength of 520 nm. Toluene was used as a blank. Proline concentrations (mg/g, fresh weight [FW] basis) were calculated using L-proline for the standard curve.
Measurement of K+ flux in roots
Net K+ fluxes were measured noninvasively by the NMT (BIO-001A; Younger USA, Amherst, MA) according to previously described methods.Citation22–Citation28 The K+ concentration gradients were measured by moving the K+-specific microelectrode between two positions close to the root in a preset excursion (30 μm) at a programmable frequency of 0.2 Hz. Fluxes were automatically recorded at the root surface in 0.1 mM KCl, 0.1 mM CaCl2, 0.3 mM MES, pH 6.0 after 5–10 min of reaching the steady-state fluxes.
Measurement of potassium content in roots
One and seven days after treatment seedlings in GS were harvested. Roots from each treatment were collected and dried. Part of the roots were cut into 1 cm fragments. The potassium content (mg/g, dry weight [DW] basis) in roots was measured by atomic absorption spectrometry and calculated using the standard curve of potassium.
Real-time PCR
Total RNA was isolated using TRIzol reagent (Invitrogen, US) according to the manufacturer’s instructions. Real-time PCR (qPCR) was performed using 2× UltraSYBR Mixture (CoWin Biosciences Company, Beijing, China) in triplicate with LineGene9600 Plus (Bioer, Hangzhou, China). Primer sequences (from Sunbiotech Co., Ltd., Beijing, China) for the targeted SKOR genes were forward, GCCAATTCACCTTCCTCGTAGA, reverse, CGATGGAGGCAGAGTTGTAGC. Primer for the reference gene is actin, were forward, CTCTGAGAATCACCGCCAACTACT, reverse, AGGCATATACTCTGGAGGCTTCAC. The relative expression was calculated with the 2−ΔΔCT method (ΔCT = CTSKOR – CTactin).
Statistical analysis
In all experiments, data were obtained from at least three independent samples and the error bars in the figures represent the standard errors (SE). All statistical analyses were performed by one-tailed Student’s t-test. Among multi-group comparisons, the ANOVA was used. The results are presented as the mean ± SE (p < .05 is significant, p < .01 is extremely significant).
Results
PEG + ABA treatment increased the free proline content
The free proline content of roots after 7 d of treatment with PEG, ABA, and PEG + ABA was measured to determine the degree of drought tolerance levels (). PEG and PEG + ABA treatment led to an increased free proline content in roots in both species. ABA treatment did not affect the free proline content significantly in either C. fargesii or C. fargesii f. duclouxii roots. Notably, when treated with PEG + ABA, the free proline content increased to approximately 2.5-fold of the value in the PEG treatment group in the roots of C. fargesii. However, in the roots of C. fargesii f. duclouxii, the free proline contents were similar in the PEG and PEG+ABA groups. The results showed that the free proline of C. fargesii roots was dependent on ABA induction, but that in C. fargesii f. duclouxii roots was independent of ABA under drought conditions.
PEG increased K+ efflux and ABA reduced K+ loss
To select an optimal location to determine the K+ flux in roots, we measured the K+ flux at 0, 500, 700, 1200, 1800, 5000, and 10 000 μm from the root tip in both species. The largest K+ flux occurred at 500–700 μm in both species (Figure S3); thus, we measured the K+ flux 600 μm from the root tip in our studies.
In C. fargesii roots, the efflux of K+ was significantly (p < .001) elevated after short-term(1 d) PEG treatment (1022 pmol cm−2s−1) compared to that after the CK, ABA, and PEG + ABA treatments (170, 87, and 53 pmol cm−2s−1, respectively) (). After long-term (7 d) treatment, K+ efflux (737 pmol cm−2s−1) was still higher in the PEG treatment group than in the CK group. However, ABA and PEG + ABA treatments showed a lower K+ efflux from roots relative to CK, with rates of 161 and 58 pmol cm−2s−1, respectively ().
Figure 2. K+ efflux in roots of C. fargesii and C. fargesii f. duclouxii after 1 d of treatment with PEG, ABA, or PEG + ABA. Average K+ efflux for the measurement period (400 s) (c). Positive values indicate K+ efflux. n = 3–6, P < .01.
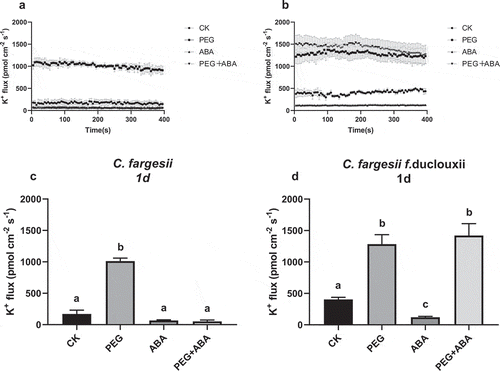
Figure 3. K+ efflux in roots of C. fargesii (a, b) and C. fargesii f. duclouxii (c, d) after 7 d of treatment with PEG, ABA, or PEG + ABA. Positive values indicate K+ efflux. n = 3–6, P < .01.
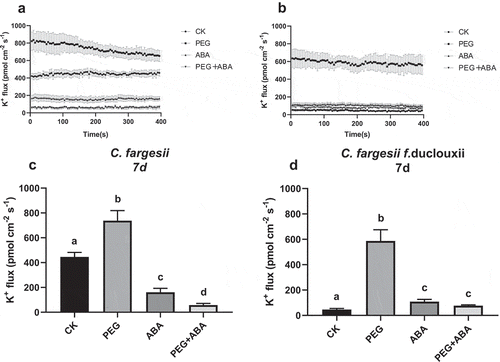
One day of PEG treatment led to a significant increase in K+ efflux in C. fargesii f. duclouxii compared with the control (p < .001; 1 d, ). K+ efflux in the PEG + ABA treatment group (1402 pmol cm−2s−1) was not significantly different from that of the PEG treatment group, but ABA caused a significant decrease in K+ efflux (96 pmol cm−2s−1; p < .001) relative to PEG. K+ efflux decreased significantly (p < .001) after 7 d compared to treatment for 1 d (). After 7 d, K+ efflux in CK (55 pmol cm−2s−1) was not significantly different from that in the ABA or ABA + PEG treatments (106 and 109 pmol cm−2s−1, respectively). Plants treated with PEG had the highest K+-efflux values (297 pmol cm−2s−1).
We used tetraethylammonium (TEA), a K+ channel blocker, to determine whether K+ efflux was regulated through K+ channels. Seedlings were treated for 1 d with CK, PEG, ABA, or PEG + ABA, followed by 1 h the TEA treatment. In C. fargesii seedlings, K+ efflux in the CK and CK + TEA treatments was 260 and 212 pmol cm−2s−1, respectively (). In C. fargesii f. duclouxii seedlings, K+ efflux was 406 and 11 pmol cm−2s−1 in CK and CK + TEA, respectively. K+ efflux was significantly (p < .001) decreased in seedlings cultured in PEG, ABA, and ABA + PEG and treated with TEA compared to CK without TEA. Catalpa fargesii exhibited higher K+ efflux than C. fargesii f. duclouxii after short-term treatment (CK, PEG, ABA, or PEG+ABA) followed by TEA treatment (p < .001).
K+ content, PEG increased K+ efflux and ABA reduced K+ loss
The K+ content was increased in the PEG 1d treatment group in C. fargesii roots, but was decreased in C. fargesii f. duclouxii roots. The K+ content became balanced after 7 d (). A faster response occurred in C. fargesii than in fargesii f. duclouxii. ABA can aid K+ retention in C. fargesii more than in C. fargesii f. duclouxii roots.
SKOR genes were activated by PEG
SKOR is a type of K+ channel for K+ outward movement of K+ from cell. After 1 d of PEG treatment, the expression of SKOR was sharply increased in C. fargesii f. duclouxii roots, but did not change significantly in C. fargesii roots (). With the extension of the treatment period, SKOR expression decreased in both C. fargesii and C. fargesii f. duclouxii roots (). The results showed that SKOR has a better sensitivity to drought stress in C. fargesii f. duclouxii than in C. fargesii.
Figure 6. SKOR gene expression levels in roots of Catalpa fargesii and C. fargesii f. duclouxii seedlings treated for 1 d (a) and 7 d (b) with PEG, ABA, or PEG + ABA. n = 3–6, P < .01.
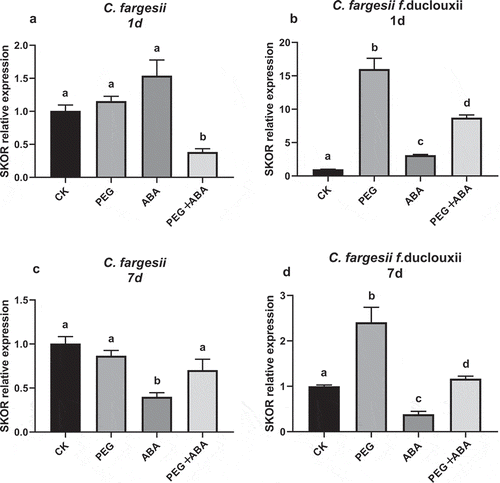
SKOR is an outward- rectifying K+ channel located on the cell membrane. After PEG treatment, the SKOR expression in roots of C. fargesii and C. fargesii f. duclouxii roots showed different trends with the prolongation of drought stress time. At 1 day of treatment, the SKOR gene was significantly upregulated in C. fargesii f. duclouxii root, but a change was not obvious in C. fargesii. After 7 d of treatment, the SKOR gene was downregulated in C. fargesii root, while that in C. fargesii f. duclouxii roots was still upregulated. This indicates that SKOR gene expression in C. fargesii roots and C. fargesii f. duclouxii roots is sensitive to PEG stress, but the downregulated expression of SKOR in C. fargesii roots is beneficial to K+ retention in cells.
Discussion
Drought-induced osmotic stress critically affects the growth of forest trees.Citation1 Roots are the first plant organs to sense soil drought signals, and thus, the root cell membrane is the first line of defense against drought stress.Citation29 It has generally been thought that cell membrane integrity is a major determinant of the effects of drought on plants.Citation30 However, ion channels may also play an important role in mediating drought stress. Here, we used ABA to investigate the roles of K+ channels under drought stress in plant roots. ABA and K+ affect water balance under drought and salt stress conditions primarily through guard cell regulation, and increase cellular resistance to dehydration by inducing the expression of dehydration-tolerance proteins.Citation3,Citation31-Citation33
In the present study, drought stress enhanced root K+ efflux in both Catalpa species ( and ). After 1 d of PEG treatment, K+ efflux in roots of C. fargesii and C. fargesii f. duclouxii seedlings was more than 19-fold and 3-fold higher than that in the respective controls. After 7 d of treatment, root K+ efflux was 1.4-fold (C. fargesii) and 5.4-fold (C. fargesii f. duclouxii) higher than that in the respective controls. These results are consistent with other studies that showed that abiotic stresses, including drought, salinity, and reactive oxygen species induced K+ efflux in plant roots.Citation34-Citation38 K+ efflux was partially inhibited by ABA () compared with PEG-6000 treatment in both species. The role of ABA as a K+ channel inhibitor was reported in previous studies that showed that ABA decreased the outward K+ current in maize roots and cells.Citation17,Citation38 After short-term treatment with PEG, ABA, or PEG + ABA, we treated Catalpa seedlings with TEA for 60 min and found that ABA significantly reduced (but did not eliminate) K+ efflux (). This observation led us to conclude that most of the K+ supply moves out of plant cells subjected to drought stress (PEG-6000 treatment). C. fargesii exhibited greater K+ efflux than C. fargesii f. duclouxii after 1 d of PEG-6000 treatment, but the trend was reversed after 7 d of treatment. Plant drought resistance is complex and involves various factors, including protective enzymes, solutes, and signal transduction. Thus, K+ accumulation in plant roots may be an important adaptive mechanism for survival under drought stress.Citation39
Gene regulation is an elemental factor in drought stress defense mechanisms. To reveal the mechanism and underlying reason why ABA improves drought tolerance by inhibiting K+ channels and efflux, we detected K+ outward channel gene SKOR. SKOR was identified in 1998,Citation40 and regulates K+ efflux by the ABA-dependent pathway to improve drought tolerance.Citation41-Citation43 The SKOR and KAT driving forces for K+ are inwardly directed, and could be of use to improve plant growth on K+‐starved soil.Citation44 In these results, the expression of SKOR decreased in C. fargesii and increased in C. fargesii f. duclouxii with 1 day of drought stress, but it increased in roots of both Catalpa species at 7 d of drought stress. ABA can reduce SKOR expression in both C. fargesii and C. fargesii f. duclouxii, but C. fargesii was more sensitive to ABA. The results showed that SKOR expression is consistent with K+ efflux and has a positive relationship, with the K+ content is negative. ABA can block drought induced-SKOR expression and K+ efflux is significantly reduced in C. fargesii.
In summary, we found that root cells of two Catalpa species were damaged under simulated drought conditions, resulting in increased K+ efflux under drought treatment. We propose that ABA can improve drought resistance by reducing K+ efflux and inhibiting the expression of SKOR and the activity of K+ channels. However, we found two patterns, including ABA-dependent and ABA-independent patterns, expressed as different reactions that are resistant and sensitive to drought in two Catalpa species, respectively. In this study, the NMT provided a powerful tool for real-time detection of K+ efflux in intact woody roots without damaging cells. In the future, we will apply the NMT with molecular biology methods to reveal the mechanisms by which ABA regulates K+ channel genes. For example, scientists have analyzed ABA regulated miRNAs or lncRNAs at the level of transcription during drought.Citation45 We expect to improve our understanding of the mechanisms of drought tolerance in tree species of economic importance by combining molecular biology methods with electrophysiological methods.
Disclosure of Potential Conflicts of Interest
No potential conflicts of interest were disclosed.
Abbreviations
ABA | = | Abscisic acid |
NMT | = | Non-invasive micro-test technique |
TEA | = | Tetraethylammonium |
PEG | = | Polyethylene glycol |
SKOR | = | Stelar K+ outward-rectifying channel |
Supplemental Material
Download Zip (140.3 KB)Supplementary Material
Supplemental data for this article can be accessed on the publisher’s website.
Additional information
Funding
References
- Aranda I, Gil-Pelegrín E, Gascó A, Guevara MA, Cano JF, De Miguel M, Ramírez-Valiente JA, Peguero-Pina JJ, Perdiguero P, Soto Aet al. Drought response in forest trees: from the species to the gene. Plant Responses Drought Stress. 2012;1–9. doi:10.1007/978-3-642-32653-0_12.
- Mahdid M, Kameli A, Ehlert C, Simonneau T. Rapid changes in leaf elongation, ABA and water status during the recovery phase following application of water stress in two durum wheat varieties differing in drought tolerance. Plant Physiol Biochem. 2011;49:1077–1083. doi:10.1016/j.plaphy.2011.08.002.
- Zhu JK. Salt and drought stress signal transduction in plants. Annu Rev Plant Biol. 2002;53:247–273. doi:10.1146/annurev.arplant.53.091401.143329.
- De Leonardis A, Petrarulo M, De Vita P, Mastrangelo A. Genetic and molecular aspects of plant response to drought in annual crop species. Adv Sel Plant Physiol Aspects: InTech. 2012. doi:10.5772/31352.
- Yoshida T, Mogami J, Yamaguchi-Shinozaki K. ABA-dependent and ABA-independent signaling in response to osmotic stress in plants. Curr Opin Plant Biol. 2014;21:133–139. doi:10.1016/j.pbi.2014.07.009.
- Liu S, Lv Z, Liu Y, Li L, Zhang L. Network analysis of ABA-dependent and ABA-independent drought responsive genes in Arabidopsis thaliana. Genet Mol Biol. 2018;41:624–637. doi:10.1590/1678-4685-GMB-2017-0229.
- Shi H, Ma W, Song J, Lu M, Rahman SU, Bui TTX, Vu DD, Zheng H, Wang J, Zhang Y, et al. Physiological and transcriptional responses of Catalpa bungei to drought stress under sufficient- and deficient-nitrogen conditions. Tree Physiol. 2017;37:1457–1468. doi:10.1093/treephys/tpx090.
- Wang P, Ma L, Li Y, Wang S, Li L, Yang R, Ma Y, Wang Q. Transcriptome profiling of indole-3-butyric acid-induced adventitious root formation in softwood cuttings of the Catalpa bungei variety ‘YU-1ʹat different developmental stages. Genes Genomics. 2016;38:145–162. doi:10.1007/s13258-015-0352-8.
- Morgan J. Osmoregulation as a selection criterion for drought tolerance in wheat. Aust J Agric Res. 1983;34:607–614. doi:10.1071/AR9830607.
- Umezawa T, Nakashima K, Miyakawa T, Kuromori T, Tanokura M, Shinozaki K, Yamaguchi-Shinozaki K. Molecular basis of the core regulatory network in ABA responses: sensing, signaling and transport. Plant Cell Physiol. 2010;51:1821–1839. doi:10.1093/pcp/pcq156.
- Rajasheker G, Jawahar G, Jalaja N, Kumar SA, Kumari PH, Punita DL, Karumanchi AR, Reddy PS, Rathnagiri P, Sreenivasulu N, et al. Role and regulation of osmolytes and ABA interaction in salt and drought stress tolerance. Plant Signaling Mol. 2019;417–436. doi:10.1016/b978-0-12-816451-8.00026-5.
- Ullah A, Manghwar H, Shaban M, Khan AH, Akbar A, Ali U, Ali E, Fahad S. Phytohormones enhanced drought tolerance in plants: a coping strategy. Environ Sci Pollut R. 2018;25:33103–33118. doi:10.1007/s11356-018-3364-5.
- Tuteja N. Abscisic acid and abiotic stress signaling. Plant Signal Behav. 2007;2:135–138. doi:10.4161/psb.2.3.4156.
- Becker D, Hoth S, Ache P, Wenkel S, Roelfsema MRG, Meyerhoff O, Hartung W, Hedrich R. Regulation of the ABA-sensitive Arabidopsis potassium channel gene GORK in response to water stress. FEBS Lett. 2003;554:119–126. doi:10.1016/S0014-5793(03)01118-9.
- Maathuis FJM, Sanders D. Mechanisms of potassium absorption by higher plant roots. Physiol Plant. 1996;96:158–168. doi:10.1111/j.1399-3054.1996.tb00197.x.
- Xiong L, Zhu J-K. Molecular and genetic aspects of plant responses to osmotic stress. Plant Cell Environ. 2002;25:131–139. doi:10.1046/j.1365-3040.2002.00782.x.
- Roberts SK. Regulation of K+ channels in maize roots by water stress and abscisic acid. Plant Physiol. 1998;116:145–153. doi:10.1104/pp.116.1.145.
- Roberts SK, Snowman BN. The effects of ABA on channel‐mediated K+ transport across higher plant roots. J Exp Bot. 2000;51:1585. doi:10.1093/jexbot/51.350.1585.
- van den Wijngaard PW, Sinnige MP, Roobeek I, Reumer A, Schoonheim PJ, Mol JN, Wang M, De Boer AH. Abscisic acid and 14-3-3 proteins control K channel activity in barley embryonic root. Plant J. 2005;41:43–55. doi:10.1111/j.1365-313X.2004.02273.x.
- Jeannette E, Rona JP, Bardat F, Cornel D, Sotta B, Miginiac E. Induction of RAB18 gene expression and activation of K+ outward rectifying channels depend on an extracellular perception of ABA in Arabidopsis thaliana suspension cells. Plant J. 1999;18:13–22. doi:10.1046/j.1365-313X.1999.00423.x.
- Bates LS, Waldren RP, Teare ID. Rapid determination of free proline for water-stress studies. Plant Soil. 1973;39:205–207. doi:10.1007/BF00018060.
- Kühtreiber WM, Jaffe LF. Detection of extracellular calcium gradients with a calcium-specific vibrating electrode. J Cell Biol. 1990;110:1565–1573. doi:10.1083/jcb.110.5.1565.
- Jian S, Shaoliang C, Songxiang D, Ruigang W, Niya L, Xin S, Zhou X, Lu C, Zheng X, Hu Z, et al. NaCl-induced alternations of cellular and tissue ion fluxes in roots of salt-resistant and salt-sensitive poplar species. Plant Physiol. 2009;149:1141–1153. doi:10.1104/pp.108.129494.
- Sun J, Wang MJ, Ding MQ, Deng SR, Liu MQ, Lu CF, Zhou XY, Shen X, Zheng XJ, Zhang ZK, et al. H2O2 and cytosolic Ca2+ signals triggered by the PM H-coupled transport system mediate K+/Na+ homeostasis in NaCl-stressed Populus euphratica cells. Plant Cell Environ. 2010;33:943–958. doi:10.1111/j.1365-3040.2010.02118.x.
- Rui-Rui X, Sheng-Dong Q, Long-Tao L, Chang-Tian C, Chang-Ai W, Cheng-Chao Z. A DExD/H box RNA helicase is important for K+ deprivation responses and tolerance in Arabidopsis thaliana. Febs J. 2011;278:2296–2306. doi:10.1111/j.1742-4658.2011.08147.x.
- Jian S, Wang R, Xuan Z, Yu Y, Rui Z, Li Z, Chen S. Hydrogen sulfide alleviates cadmium toxicity through regulations of cadmium transport across the plasma and vacuolar membranes in Populus euphratica cells. Plant Physiol Biochem. 2013;65:67–74. doi:10.1016/j.plaphy.2013.01.003.
- Jie L, Jingjing Q, Fangfang H, Hong L, Tongxian L, Andrea P, Peng C, Luo Z-B. Net fluxes of ammonium and nitrate in association with H+ fluxes in fine roots of Populus popularis. Planta. 2013;237:919–931. doi:10.1007/s00425-012-1807-7.
- Živanović BD, Pang J, Shabala S. Light-induced transient ion flux responses from maize leaves and their association with leaf growth and photosynthesis. Plant Cell Environ. 2005;28:340–352. doi:10.1111/j.1365-3040.2005.01270.x.
- And WJD, Zhang J. Root signals and the regulation of growth and development of plants in drying soil. Annu Rev Plant Physiol Plant Mol Biol. 1991;42:55–76. doi:10.1146/annurev.pp.42.060191.000415.
- Bajji M, Kinet JM, Lutts S. The use of the electrolyte leakage method for assessing cell membrane stability as a water stress tolerance test in durum wheat. Plant Growth Regul. 2002;36:61–70. doi:10.1023/a:1014732714549.
- Chérel I, Gaillard I. The complex fine-tuning of K+ fluxes in plants in relation to osmotic and ionic abiotic stresses. Int J Mol Sci. 2019;20:715. doi:10.3390/ijms20030715.
- Daszkowska-Golec A, Szarejko I. The molecular basis of ABA-mediated plant response to drought. Kourosh Vahdati Charles Leslie. 2013. doi:10.5772/53128.
- Tavakol E, Jákli B, Cakmak I, Dittert K, Karlovsky P, Pfohl K, Senbayram M. Optimized potassium nutrition improves plant-water-relations of barley under PEG-induced osmotic stress. Plant Soil. 2018;430:23–35. doi:10.1007/s11104-018-3704-8.
- Shabala SN, Lew RR. Turgor regulation in osmotically stressed Arabidopsis epidermal root cells. Direct support for the role of inorganic ion uptake as revealed by concurrent flux and cell turgor measurements. Plant Physiol. 2002;129:290–299. doi:10.1104/pp.020005.
- Chen Z, Shabala S, Mendham N, Newman I, Zhang G, Zhou M. Combining ability of salinity tolerance on the basis of NaCl-induced K+ flux from roots of barley. Crop Sci. 2008;48:1382–1388. doi:10.2135/cropsci2007.10.0557.
- Vadim D, Cuin TA, Dimitri S, Smith SJ, Miller AJ, Sergey S, Sokolik A, Yurin V. Arabidopsis root K+-efflux conductance activated by hydroxyl radicals: single-channel properties, genetic basis and involvement in stress-induced cell death. J Cell Sci. 2010;123:1468–1479. doi:10.1242/jcs.064352.
- Cuin TA, Betts SA, Chalmandrier R, Shabala S. A root’s ability to retain K+ correlates with salt tolerance in wheat. J Exp Bot. 2008;59:2697–2706. doi:10.1093/jxb/ern128.
- Wolf T, Heidelmann T, Marten I. ABA regulation of K(+)-permeable channels in maize subsidiary cells. Plant Cell Physiol. 2006;47:1372–1380. doi:10.1093/pcp/pcl007.
- Cai K, Gao H, Wu X, Zhang S, Han Z, Chen X, Zhang G, Zeng F. The ability to regulate transmembrane potassium transport in root is critical for drought tolerance in barley. Int J Mol Sci. 2019;20:4111. doi:10.3390/ijms20174111.
- Gaymard F, Pilot G, Lacombe B, Bouchez D, Bruneau D, Boucherez J, Michaux-Ferrière N, Thibaud J-B, Sentenac H. Identification and disruption of a plant shaker-like outward channel involved in K+ release into the xylem sap. Cell. 1998;94:647–655. doi:10.1016/S0092-8674(00)81606-2.
- Hu J, Ma Q, Kumar T, Duan H-R, Zhang J-L, Yuan H-J, Wang Q, Khan SA, Wang P, Wang S-M, et al. ZxSKOR is important for salinity and drought tolerance of zygophyllum xanthoxylum by maintaining K+ homeostasis. Plant Growth Regul. 2016;80:195–205. doi:10.1007/s10725-016-0157-z.
- Johansson I, Wulfetange K, Porée F, Michard E, Gajdanowicz P, Lacombe B, Sentenac H, Thibaud J-B, Mueller-Roeber B, Blatt MR, et al. External K+ modulates the activity of the Arabidopsis potassium channel SKOR via an unusual mechanism. Plant J. 2006;46:269–281. doi:10.1111/j.1365-313X.2006.02690.x.
- Liu K, Li L, Luan S. Intracellular K+ sensing of SKOR, a shaker-type K+ channel from Arabidopsis. Plant J. 2006;46:260–268. doi:10.1111/j.1365-313X.2006.02689.x.
- Gajdanowicz P, Garcia-Mata C, Gonzalez W, Morales-Navarro SE, Sharma T, González-Nilo FD, Gutowicz J, Mueller‐Roeber B, Blatt MR, Dreyer I. Distinct roles of the last transmembrane domain in controlling Arabidopsis K+ channel activity. New Phytol. 2009;182:380–391. doi:10.1111/j.1469-8137.2008.02749.x.
- Wu C, Ding Z, Chen M, Yang G, Tie W, Yan Y, Zeng J, He G, Hu W. Identification and functional prediction of lncRNAs in response to PEG and ABA treatment in cassava. Environ Exp Bot. 2019;166:103809. doi:10.1016/j.envexpbot.2019.103809.