Abstract
Nanoscience and technology play an essential role in different various scientific fields domains as since it offers unique properties and diverse industrial applications. This study introduces an efficient method to synthesize silver nanoparticles (AgNPs) using Aristolochia bracteolate bud extract. The synthesized AgNPs are embedded within Poly (methyl methacrylate) (PMMA) to prepare a sustainable nanocomposite film (Ag-PMMA NC). Characterization techniques reveal the distinct properties of AgNPs and Ag-PMMA NC. Thermogravimetric analysis (TGA) shows enhanced heat stability of the polymer with Ag nano-filler. UV-vis spectroscopy detects a peak for AgNPs at 440 nm, with an average size of 180.4 nm and a polydispersity index (PDI) of 0.389, indicating uniform size. Fourier transform infrared spectroscopy (FTIR) of Ag-PMMA exhibits characteristic peaks between 300-700 cm-−1, confirming Ag-PMMA interactions. Both green-synthesized AgNPs and the resulting Ag-PMMA NC film demonstrate potent antibacterial activity against Gram-positive Bacillus cereus (∼15 mm) and Gram-negative Escherichia coli (∼26 mm), showing promise for water purification and other applications.
1. Introduction
Nanomaterials refer to materials or chemical substances that are engineered at the nanoscale, and they are an essential component in the current scientific and engineering fields [Citation1,Citation2]. These complex nanostructures have captured the interest of scientists all over the world owing to their unique characteristics, which influence a wide range of fields’ aspects such as physical, chemical, electrical, biological, and optoelectrical performance [Citation3]. In recent times, the manufacturing of nanoparticles has been revolutionized with the advent of green synthesis. As a result, nano-products have been brought into existence which has a countless applications ranging from medicinal, cosmetics, renewable energy, environmental remediation, and medicine-related industries aspects [Citation4,Citation5].
Among the noble metal nanoparticles, silver nanoparticles are one of the most sought after attractive ones. Silver nanoparticles show unique physical–chemical properties enabling their use in colorimetric sensors, bactericidal finishing, and electrochemical work [Citation6,Citation7]. The fabrication of AgNPs from plant sources is environmentally friendly and provides particles with mechanical, thermal, optical, and biological properties that range in size from 1 to 100 nm [Citation8]. Because of these qualities, AgNPs are highly valued in the fields of catalysis, electrochemistry, biomedicines, pharmaceuticals, sensors, and cosmetics. They are used in the production of antibacterial medications, materials for healing wounds, drug delivery systems, and nanocomposite films for food packaging [Citation9].
Green synthesis approaches have many advantages over conventional methods, including ease of use, cost savings, and repeatability. They also produce stable compounds without the need of toxic chemicals, high pressure, energy, or temperature input [Citation10]. Plant-based synthesis yields stable, contamination-resistant nanoparticles that are accessible and necessary for maintaining the biocompatibility required for both environmental safety and human health [Citation1,Citation11]. The inclusion of inorganic nanoparticles has a considerable impact on polymer matrix, optical, magnetic, thermal, flammability, mechanical and electrical properties. Different nanocomposite properties will vary depending on the type of nanoparticle, its size, shape, concentration, and interaction with the polymer matrix [Citation2,Citation12–14].
The goal of this study is to create stable silver nanoparticles (AgNPs) by reducing silver nitrate with an aqueous extract of Aristolochia bracteolate buds. These AgNPs were used to fabricate a unique nanocomposite film, AgNPs-PMMA. In addition, a green synthesis approach was used to produce novel AK-AgNPs-PMMA. AgNPs and Ag-PMMA nanocomposite synthesized were well-characterized and authenticated in this strategic study. TEM, UV-Vis spectrophotometer, fluorescence spectrophotometer, DLS, FTIR, and EDS were used to characterize these nanomaterials.
Furthermore, through the thermal stability test alongside using thermogravimetric analysis and germination testing, as well as antibacterial experiment on different kinds of microbes, the application of silver nanoparticles incorporated into PMMA nanocomposite as a biofilter for tap water purification is presented. The main task was to address critical environmental and health-related issues using cutting-edge advancements, combining nanomaterials with advanced polymer matrices, and investigate potential mechanisms for the antibacterial activity of the Ag-PMMA nanocomposite.
2. Materials and method
2.1. Chemicals, reagents, and sample collection
The precursor silver nitrate AgNO3 was obtained from Sigma Aldrich Chemicals Pvt. Ltd., India, and Nutrient Agar from Hi-Media, India. PMMA polymer poly methyl methacrylate was supplied by SABIC, Saudi Arabia. N’,N-dimethylformamide DMF was purchased from R & M Marketing, Essex, UK, as a dissolving agent to dissolve PMMA.
2.2. Preparation of Aristolochia bracteolate buds extract
Aristolochia bracteolate buds (A. bracteolate) underwent extensive meticulous purification with tap water followed by distilled water. Subsequently, these buds were air-dried in the shade for 4–5 days, and were grounded into a fine powder, and stored in a sealed container. A solution of 100 mL double-distilled water was heated to 70°C for 15 min using a heating mantle and then combined with 10 g of the powdered buds. Through this method, the water solution effectively extracted all phytochemicals present in the A. bracteolate bud. The resulting aqueous A. bracteolate bud extract was filtered using Whatman No. 1 filter paper and refrigerated for subsequent use.
2.3. Preparation of green silver nanoparticles (Ag NPs)
To create environmentally friendly silver nanoparticles (AgNPs), 90 ml of deionized water and 1 mM of precursor AgNO3 were combined in a solution and warmed to 45°C. Then, 10 ml of the filtered extract of A. bracteolate was added to the mixture. A magnetic stirrer with a 600–700 rpm speed was used to vigorously spin the liquid for 10 min. Successful synthesis of nanoparticles was confirmed by an apparent change in the colloidal solution’s colour, which was colourless prior to synthesis and turned light yellow that evolved to dark brown after 15 min. This alteration of the fluid colour is brought by the reduction of silver ions to metallic silver nanoparticles.
2.4. Preparation of AgNPs-based PMMA film nanocomposite (Ag-PMMA NC)
The protocol for the preparation of a silver nanoparticle-based PMMA film nanocomposite Ag-PMMA NC was pursued according to [Citation2]. A total of 6 g of PMMA was dissolved in 50 ml of DMF. To form the PMMA solution, the producer added one with a freshly prepared solution of Ag NPs while still being stirred and heated at 80°C under the fume hood. The precursor-materials reaction was under process allowed to continue for one hour and resulted in the formation of light brown solutions of ionized Ag NPs. Following this, 3 ml of the synthesized Ag-PMMA NC mixture was poured and casted into a glass petri-dish, and the nanocomposite film was produced through the room-temperature evaporation of DMF. Notably, this is the first study to synthesise silver nanoparticles and AgNPs-PMMA nanocomposites utilizing A. bracteolate extract to the best of our knowledge (Figure ) [Citation15].
2.5. Instrumentation
UV–visible spectrophotometry, employing a JASCO V-770 UV-Visible/NIR instrument (Tokyo, Japan) within the 200–800 nm range, was used to monitor the formation of green AgNPs in the aqueous A. bracteolate extract, and their emission spectrum from 300 to 900 nm were acquired using a Shimadzu RF-6000 spectrofluorometer (Kyoto, Japan). The chemical composition of green AgNPs along with AgNPs-PMMA nanocomposites and PMMA films underwent thorough analysis using sophisticated instrumentation. Fourier-transform infrared (FTIR) spectroscopy, conducted using a Shimadzu IR Prestige 21 instrument (Kyoto, Japan) with a spectral range of 400–4400 cm−1, provided insights into the molecular structure. X-ray diffractometry, performed using a Bruker D8 Discover instrument (Billerica, Massachusetts, USA) in the 10° to 80° range, elucidated phase purity and crystalline structure. These analyses were conducted at the Physics and Chemistry Department of King Saud University – Female Students Campus in Riyadh, Saudi Arabia. To delve into morphology and particle size, Transmission Electron Microscopy (TEM) analysis was performed using a JEM-1011 from JEOL, (Akishima, Tokyo, Japan). Particle size of the synthesized AgNPs was further evaluated using dynamic light scattering via a zetasizer from the Nano series, HT Laser, ZEN3600 Molvern Instrument, (Malvern, UK). Energy dispersive X-ray spectrometry (EDX) was carried out using a scanning electron microscope (SEM) JSM-7610F from Joel (Akishima, Tokyo, Japan) to inspect the AgNPs-PMMA nanocomposite film. For thermal analysis, approximately 4 mg of dried AgNPs-PMMA NC film underwent Thermogravimetric Analysis (TGA) TGA/DSC 1, Mettler Toledo, (Columbus, United States) in the 0–800°C range under nitrogen flow at a rate of 10°C/min. TGA thermograms were plotted to illustrate weight percentage loss and heat flow against temperature.
2.6. Antimicrobial study
The disc diffusion method was used to test the antibacterial activity of AgNPs/PMMA NC film against a Gram-positive B. cereus clinical isolate (ATCC 11778) and a Gram-negative E. coli isolate (ATCC35218). The nutrient agar medium plates were produced, autoclaved, and solidified. After solidification, the bacterial culture was distributed on the solidified agar plate using a swab stick. A portion of pure PMMA and AgNPs-PMMA nanocomposite film was placed in a nutrient agar plate and incubated for 24 h at 37°C. The zone of inhibition was assessed on a zone scale. The experiments were repeated three times for each sample, and the average value of the zone diameter was computed [Citation14,Citation15].
2.7. Water microbiological testing
The plate count method checked the presence of microorganisms in tap water. In classic microbiology, this approach determines the number of bacterial colonies developed on nutritional medium, which are observed with the naked eye. It is important to dilute the original sample to obtain colonies on the Big but counting on the Big in the range from 30 to 300 in order to measure it with confidence. The culturing of several dilutions is the standard laboratory method for evaluating the efficacy of water treatment against microbiological pollution such as E. coli [Citation16,Citation17]. To determine the growth of microbes in tap water, 1 × 1 cm squares of Ag-PMMA NC was immersed in 50 ml of tap water in Pyrex glass Erlenmeyer flasks for 48 h. This includes the manufacturing of Nutrient agar, Eosin methylene blue agar, and Mueller-Hinton agar not the necessary quantity was added and everything was incubated. After stirring 100 ml of inoculums of tap water, treated in or untreated, and tapping it into petri plates and incubating at 37°C for 24–48 h, various colonies of the microorganisms are grown. This allows for a comprehensive assessment of both normal and treated tap water samples, resulting in a more accurate microbiological analysis [Citation14,Citation15].
3. Result and discussion
Fluorescence (Fluo) spectrum of green AgNPs serves a significant tool enabling a deeper understanding of the optical intricacies and activities of silver nanoparticles and broaden its viable applications. The Fluo properties of the green-synthesised AgNPs must be analysed using fluorescence emission spectroscopy to obtain a better understanding of its optical properties. Emission spectroscopy is a very crucial tool in the assessment of the optical behaviours of AgNPs as photonic materials for different applications. Aqueous green colloidal AgNPs suspension was used to analyse Fluo emission spectrum at λem 777 nm (), following which an excitation wavelength was set that has and demonstrated a red-shifted peak. In the visible spectrum, the localization of a 770 nm wavelength within the red region signifies the emission range of silver nanoparticles. This wavelength intricately reflects the size, shape, surface chemistry, and ambient conditions surrounding the nanoparticles, as eloquently stated by K. Lance Kelly, particularly noting the sensitivity of the red-most peak, specifically at 770 nm, to the shapes and structural nuances for the synthesized nanoparticles [Citation18].
The TEM images in Figure (A,B) depict various shapes of silver nanoparticles (AgNPs), including triangles, snipped, and spherical monodisperse structures. Many factors, as reported by previous studies, can affect the sizes and distribution of green synthesized NPs. The reported variables include the type of plant extract, solution pH, and temperature. Since biomolecules act as protective and capping agents, Ag nanoparticles have shown that the extract can reduce silver ions and protect them from aggregation and assemblage. Additionally, the efficiency of the reducing agents present in the plant extract is the primary factor in determining the shape of the nanoparticles [Citation19,Citation20].
Figure 3. (A, B) display TEM images, (C) the average particle size distribution curve of green AgNPs obtained from DLS measurements.
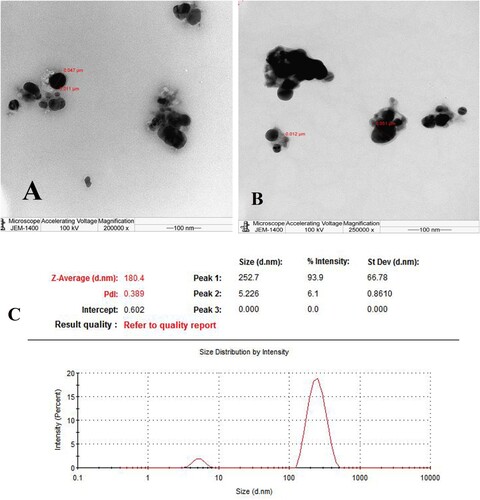
Additionally, Figure (C) presents the DLS Zetasizer results, revealing a remarkably uniform distribution of AgNPs with an average particle size of 180 nm. The size distribution graph further demonstrates monodispersity among the nanoparticles, ensuring exceptional stability over prolonged periods. Also, the Poly Dispersity Index (PDI), measured at 0.302, corroborates the high stability and slight uniformity of the produced AgNPs. These findings are consistent with and validate the results obtained from TEM analysis. They align with previous research by Goyal et al. [Citation21,Citation22].
During the reduction process, a notable change in colour is observed: initially light yellow, suggestive of the growth of AgNPs, gradually intensifying to dark brown as the reaction progresses (nucleation), indicative of the production of larger silver nanoparticles. These colour transformations are emblematic of the plasmon resonance characteristic of silver nanoparticles, a phenomenon influenced by their size, shape, and concentration within the solution. UV-visible spectra are helpful and instrumental in elucidating surface plasmon resonance phenomena. UV–Vis spectroscopy analysis of the spectra (refer to Figure ) reveals distinct peaks for different components: AgNPs exhibit a peak at 440 nm, while PMMA exhibits a broad peak spanning between 400 and 450 nm. The occurrence of Ag-PMMA nanocomposites is confirmed by a notable peak at 419 nm. Furthermore, the integration of AgNPs and the PMMA matrix is corroborated by UV–Vis spectroscopy. The UV–Vis spectra (see Figure ) reveal the surface plasmon resonance (SPR) peak of AgNPs within the PMMA matrix. Additionally, absorption peaks for both nanoparticles are observed to broaden upon their incorporation into the PMMA matrix [Citation23].
Understanding the crystallinity of nanoparticles is elucidated through XRD spectra. Figure depicts the XRD spectra of AgNPs, PMMA, and Ag-PMMA NC. AgNPs synthesized using bud extract exhibit distinct X-ray diffraction peaks, as observed in Figure (A), notably at 38.2°, 44.4°, 64.6°, and 77.6°, corresponding to hkl values of (111), (200), (220), and (311) confirming are is confirmed the presence of a cubic (fcc) structure in AgNPs. Meanwhile Additionally, there are unidentified peaks, possibly attributed to organic compounds within the bud extract [Citation13,Citation14]. PMMA exhibits a diffraction peak at 14.2° with hkl values of (001), as presented in Figure (B). Figure (C) suggests the fcc crystal structure of Ag-PMMA NC, evidenced by four distinct diffraction peaks at 2θ values of 14.2°, 34.9°, and 41.7°, corresponding to lattice planes of (001), (111), and (220). The diffractogram has been compared with the standard powder diffraction cards of JCPDS (COD1509146 (AgNPs), COD1007035 (AgNPs-PMMA NC), and COD4501412 (PMMA)). Comparing the diffraction characteristics of AgNPs, PMMA, and Ag-PMMA NC nanocomposite reveals that the smaller AgNPs embedded in PMMA broaden and attenuate the peaks associated with PMMA. Using the Debye-Scherrer equation [Citation24], the average crystalline size is determined to be 60 nm for green AgNPs.
In the current work, the AgNPs, PMMA, and Ag-PMMA NC functional groups were identified using the IR spectrum shown in Figure . The broad band of the AgNPs depicted in Figure (A) peaks at 3760, 3417, 2370, 1627, and 1388 cm−1. These peaks at 3760 cm−1 denote –OH (alcohol compounds), in the FTIR spectrum of AgNPs, because of that the extract assisted accountable for the creation of the nanoparticles, the absorption peak at wave number of 1388 cm−1 could be for aqueous extract represents the C=O group of an amide or the C=C of non-conjugated alkenes. The broad band at 3417, 2948, 1725, 1442, 1157, 980, 836, 743, and 362 cm−1 in Figure (B) according to FTIR examination is of a pure PMMA. An asymmetric C=C band corresponds to the peak at 3417 cm−1. These functional peaks were connected to the PMMA and operational points [Citation15]. Figure (C) FTIR spectrum of the Ag-PMMA NC, exhibits a broad band around wavelengths of 3760, 3417, 2948, 2370, 1725, 1442, 1157, 980, 836, 743, and 362 cm−1. In contrast to seen in the image, there is silver ion present in the band at 3760 and 2370 cm−1. As a final conclusion, Figure (C) demonstrates that the Ag-PMMA NC film had peaks at 300–700 cm−1 that were caused by the Ag-C extending movement, among Ag and the long-chain alkyl compound of PMMA. Ag–Ag metallic connections could already form below 400 cm−1, according to Leila Gharibshahi's report [Citation25].
SEM-EDX micrographs depicting the Ag-PMMA nanocomposites are presented in Figure . Figure (A) reveals the structure of the polymer, characterized by hard, smooth films interwoven together, devoid of vacuole formation. Subsequent observation indicates a biological reduction process within the nanocomposite film, as evidenced by the adornment of the PMMA polymer film network with minute AgNPs resembling spherical dots. Notably, AgNPs appeared well-dispersed in the homogenous polymer matrix [Citation26]. In a separate investigation highlighting the presence of AgNPs, Figure (B) presents the EDS spectrum, wherein Ag signals originating from the Ag-PMMA nanocomposites in the sample are clearly discernible, along with the carbon, nitrogen, and oxygen signals indicative of their respective sources.
The thermal properties of both PMMA and Ag-PMMA nanocomposites exhibit distinct behaviours, as evidenced by Thermo Gravimetric Analysis (TGA) shown in Figure .
During the initial stages of thermal degradation, PMMA and Ag-PMMA nanocomposites were subjected to heating at a rate of 100°C from ambient temperature up to 800°C. This temperature range, crucial due to the solvent's boiling point and the polymer's degradation temperature, influenced the degradation rate and provided thermal stability against degradation at higher temperatures. Beyond 800°C, the Ag-PMMA nanocomposites disintegrated, while pure PMMA started degrading around 360°C and underwent complete decomposition at 400°C. The significant weight loss observed in the polymer was attributed to fundamental degradation processes, signifying the second notable weight loss.
Furthermore, researchers have noticed the unsaturated ends of the PMMA that influence the two-step weight losses of the silver-polymer nanoparticles at 180°C and 270°C. With the help of silver, the scientists mostly noticed two-step weight losses between 158°C and 506°C using silver-polymer nanoparticles. They concluded that the silver improves the thermal stability of the PMMA such that “Even a tiny amount of Ag added into the polymer matrix, which restrains the mobility of polymer chains, not only promotes but also aids in boosting thermal stability” [Citation27–29].
Figure (A,B) depicts the antibacterial activity of PMMA and Ag-PMMA NC; respectively assessed via the disc diffusion method. Numerous studies [Citation30–32], have consistently shown that nanocomposite films possess potent antibacterial properties against diverse microorganisms. In this figure, we observe the antibacterial effectiveness of PMMA and Ag-PMMA NC against two pathogens: the gram-positive bacteria B. cereus and the gram-negative bacteria E. coli. The inhibition zones of AgNPs-PMMA NC against E. coli and B. cereus bacteria were measured at 26 and 15 mm, respectively. In contrast, the PMMA films exhibited no antibacterial activity against either E. coli or B. cereus bacteria. Remarkably, Ag-PMMA NC displays a superior antibacterial efficacy compared to PMMA alone. Evidently, gram-negative bacteria such as E. coli exhibit notably robust inhibition zones, although both types of bacteria demonstrate susceptibility to both sample types.
Figure 9. The antibacterial activity of (A) pure PMMA film, and (B) Ag-PMMA NC film against E. coli and B. cereus bacteria.
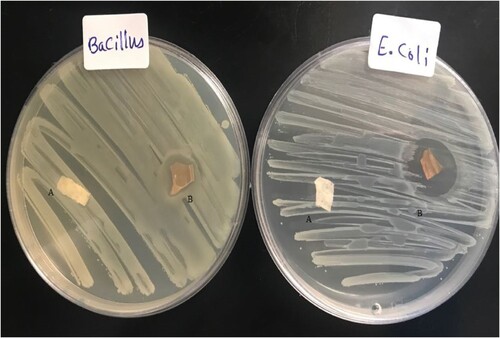
This study employed various media to conduct microbiological tests on both untreated tap water and tap water treated with Ag-PMMA NC (Figure (A,B); respectively). The findings revealed a stark contrast: while plates containing regular tap water exhibited microbial growth, those containing water treated with the nanocomposite film showed no signs of microbial proliferation. This outcome can be accredited to the presence of AgNPs in the treated water, which along with biological chemicals like nitrogen and phosphorus, are crucial for bacterial metabolic responses. Previous studies indicate that AgNPs exhibit multifaceted antimicrobial actions. They release silver ions (Ag+), disrupting bacterial cell membranes, inhibiting enzymes, and interfering with DNA replication leading to cell death. AgNPs interact directly with cell membranes, inducing damage and leakage of cellular contents. Furthermore, they generate reactive oxygen species (ROS), resulting in oxidative stress and cellular damage. The high surface area-to-volume ratio enhances contact with bacteria. Combined with polymers in nanocomposites, AgNPs bolster stability and antimicrobial activity. Gram-positive bacteria, with thicker cell walls, show lower permeability to silver ions than Gram-negative strains [Citation2,Citation33,Citation34]. Figure presents the schematic of the possible mechanisms of the antibacterial action that belongs to AgNPs/PMMA NC.
4. Conclusion
This innovative approach successfully employed the Aristolochia bracteolata bud extract to assist the eco-friendly synthesis of AgNPs, a necessary precursor for the development of the AgNPs-PMMA nanocomposite. These AgNPs exhibited strong fluorescence at an emission wavelength of 777 nm, indicating excellent optical features. Advanced instruments such as TEM and DLS further confirm the formation of spherical AgNPs, as do the UV-Vis spectroscopy results of the 440 nm peak. Starting with the DLS which revealed the formation of a more homogeneously dispersed population of AgNPs, with an average size of 180 nm showing to ensure homogeneity in the nanostructure. Furthermore, the FT-IR spectrum and XRD analysis have identified the properties of the NPs. Finally, SEM imaging revealed that many AgNPs adorned the PMMA’s surface. These results were verified by using an EDX spectroscopy. Thermogravimetric Analysis (TGA) highlighted the superior thermal stability of AgNPs-PMMA NC over PMMA. Antibacterial assays demonstrated the exceptional efficacy of Ag-PMMA against resilient Gram-negative bacteria like E. coli, with notable inhibition zones. Impressively, Ag-PMMA nanocomposite films effectively suppressed microbial growth in water treatment applications. This study underscores the industrial potential and significance of Ag-PMMA nanocomposites, paving the way for sustainable antimicrobial materials and accelerating heralding a new era in material science.
Acknowledgements
Researchers Supporting Project number (RSPD2024R1065), King Saud University, Riyadh, Saudi Arabia.
Disclosure statement
No potential conflict of interest was reported by the author(s).
Additional information
Funding
References
- Oves M, Rauf M, Aslam M, et al. Green synthesis of silver nanoparticles by Conocarpus lancifolius plant extract and their antimicrobial and anticancer activities. Saudi J Biol Sci. 2022;29(1):460–471. doi:10.1016/j.sjbs.2021.09.007
- Manal AA, Hendi AA, Ortashi KM, et al. Greener synthesis, characterization, and antimicrobiological effects of helba silver nanoparticle-PMMA nanocomposite. Int J Polym Sci. 2019;2019:1–7. doi:10.1155/2019/4379507
- Altammar KA. A review on nanoparticles: characteristics, synthesis, applications, and challenges. Front Microbiol. 2023;14:1155622. doi:10.3389/fmicb.2023.1155622
- Oves M, Rauf MA, Qari HA. Therapeutic applications of biogenic silver nanomaterial synthesized from the paper flower of Bougainvillea glabra (Miami, Pink). Nanomaterials. 2023;13(3):615. doi:10.3390/nano13030615
- Mohanta YK, Mishra AK, Nayak D, et al. Exploring dose-dependent cytotoxicity profile of Gracilaria edulis-mediated green synthesized silver nanoparticles against MDA-MB-231 breast carcinoma. Oxid Med Cell Longev. 2022;2022:3863138. doi:10.1155/2022/3863138
- Oves M, Aslam M, Rauf MA, et al. Antimicrobial and anticancer activities of silver nanoparticles synthesized from the root hair extract of Phoenix dactylifera. Sci Eng C. 2018;89:429–443. doi:10.1016/j.msec.2018.03:035
- Jayeoye TJ, Eze FN, Olatunde OO, et al. Multifarious biological applications and toxic Hg2+ sensing potentiality of biogenic silver nanoparticles based on Securidaca inappendiculata Hassk stem extract. Int J Nanomedicine. 2021;16:7557–7574. doi:10.2147/IJN.S325996
- Asif M, Yasmin R, Asif R, et al. Green synthesis of silver nanoparticles (AgNPs), structural characterization, and their antibacterial potential. Dose Response. 2022;20(2):15593258221088709. doi:10.1177/15593258221088709
- Falsaf SR, Topuz F, Bajer D, et al. Metal nanoparticles and carbohydrate polymers team up to improve biomedical outcomes. Biomed Pharmacother. 2023;168:115695. doi:10.1016/j.biopha.2023.115695
- Alshangiti DM, El-Damhougy TK, Zaher A, et al. Revolutionizing biomedicine: advancements, applications, and prospects of nanocomposite macromolecular carbohydrate-based hydrogel biomaterials: a review. RSC Adv. 2023;13(50):35251–35291. doi:10.1039/D3RA07391B
- Jayeoye J, Eze F, Olatunji OJ, et al. Synthesis of biocompatible Konjac glucomannan stabilized silver nanoparticles, with Asystasia gangetica phenolic extract for colorimetric detection of mercury (II) ion. Sci Rep. 2022;12:9176. doi:10.1038/s41598-022-13384-x
- Haghparasti Z, Shahri M. Green synthesis of water-soluble nontoxic inorganic polymer nanocomposites containing silver nanoparticles using white tea extract and assessment of their in vitro antioxidant and cytotoxicity activities. Mater Sci Eng C. 2018;87:139–148. doi:10.1016/j.msec.2018.02.026
- Chen G, Lu J, Lam C, et al. A novel green synthesis approach for polymer nanocomposites decorated with silver nanoparticles and their antibacterial activity. Analyst. 2014;139(22):5793–5799. doi:10.1039/C4AN01301H
- Awad MA, Mekhamer WK, Merghani NM, et al. Green synthesis, characterization, and antibacterial activity of silver/polystyrene nanocomposite. J Nanomater. 2015;2015:5. doi:10.1155/2015/943821
- Manal AGA, Awatif AH, Khalid MOO, et al. Synthesis of silver-PMMA nanocomposite film using herbal extract. Application US15/956,675, 2018-04-18.
- Rajapaksha P, Elbourne A, Gangadoo S, et al. A review of methods for the detection of pathogenic microorganisms. Analyst. 2019;144(2):396–411. doi:10.1039/C8AN01488D
- Hanaor D, Sorrell C. Sand supported mixed-phase TiO2 photocatalysts for water decontamination applications. Adv Eng Mater. 2014;16(2):248–254. doi:10.1002/adem.201300259
- Kelly K, Coronado E, Zhao LL, et al. The optical properties of metal nanoparticles: the influence of size, shape, and dielectric environment. J Phys Chem B. 2003;107(3):668–677. doi:10.1021/jp026731y
- Alomar TS, AlMasoud N, Awad MA, et al. Designing green synthesis-based silver nanoparticles for antimicrobial theranostics and cancer invasion prevention. Int J Nanomedicine. 2024;19:4451–4464. doi:10.2147/IJN.S440847
- Roy A, Bulut O, Some S, et al. Green synthesis of silver nanoparticles: biomolecule-nanoparticle organizations targeting antimicrobial activity. RSC Adv. 2019;9(5):2673–2702. doi:10.1039/C8RA08982E
- Klein W, Ismail E, Maboza E, et al. Green-synthesized silver nanoparticles: antifungal and cytotoxic potential for further dental applications. J Funct Biomater. 2023;14(7):379. doi:10.3390/jfb14070379
- Awad MA, Alkhulaifi M, Aldosari NS, et al. Novel eco-synthesis of PD silver nanoparticles: characterization, assessment of its antimicrobial and cytotoxicity properties. Materials. 2019;12(23):3890. doi:10.3390/ma12233890
- Thomas R, Snigdha S, Bhavitha K, et al. Biofabricated silver nanoparticles incorporated polymethyl methacrylate as a dental adhesive material with antibacterial and antibiofilm activity against Streptococcus mutans. 3 Biotech. 2018;8:1–10. doi:10.1007/s13205-018-1420-y
- Awad MA, Hendi AA, Ortashi KM, et al. Utilizing Cymbopogon proximus grass extract for green synthesis of zinc oxide nanorod needles in dye degradation studies. Molecules. 2024;29(2):355. doi:10.3390/molecules29020355
- Gharibshahi L, Saion E, Gharibshahi E, et al. Structural and optical properties of Ag nanoparticles synthesized by thermal treatment method. Materials. 2017;10(4):402. doi:10.3390/ma10040402
- Gopalakrishnan S, Mathew TA, Mozetič M, et al. Development of biocompatible and biofilm-resistant silver-poly (methylmethacrylate) nanocomposites for stomatognathic rehabilitation. Int J Polym Mater Polym Biomater. 2019;69(3):186–199. doi:10.1080/00914037.2018.1552863
- Borse S, Temgire M, Khan A, et al. Photochemically assisted one-pot synthesis of PMMA embedded silver nanoparticles: antibacterial efficacy and water treatment. RSC Adv. 2016;6(61):56674–56683. doi:10.1039/C6RA08397H
- Vodnik V, Vuković V, Nedeljković M. Synthesis and characterization of silver – poly (methylmethacrylate) nanocomposites. Colloid Polym Sci. 2009;287:847–851. doi:10.1007/s00396-009-2039-7
- Singho D, Johan R, Lah C. Temperature-dependent properties of silver-poly (methylmethacrylate) nanocomposites synthesized by in-situ technique. Nanoscale Res Lett. 2014;9:1–6. doi:10.1186/1556-276X-9-42
- Sánchez-Valdes S, Ortega-Ortiz H, Ramos-de Valle LF, et al. Mechanical and antimicrobial properties of multilayer films with a polyethylene/silver nanocomposite layer. J Appl Polym Sci. 2009;111(2):953–962. doi:10.1002/app.29051
- Wang L, Periyasami G, Aldalbahi A, et al. The antimicrobial activity of silver nanoparticles biocomposite films depends on the silver ions release behaviour. Food Chem. 2021;359:29859. doi:10.1016/j.foodchem.2021.129859
- Eze N, Jayeoye J, Eze RC. Construction, characterization and application of locust bean gum/Phyllanthus reticulatus anthocyanin-based plasmonic silver nanocomposite for sensitive detection of ferrous ions. Environ Res. 2023;228:115864. doi:10.1016/j.envres.2023.115864
- Eze N, Ovatlarnporn C, Jayeoye J, et al. One-pot biofabrication and characterization of Tara gum/Riceberry phenolics–silver nanogel: a cytocompatible and green nanoplatform with multifaceted biological applications. Int J Biol Macromol. 2022;206:521–533. doi:10.1016/j.ijbiomac.2022.02.140
- Eze N, Tola J, Nwabor F, et al. Centella asiatica phenolic extract-mediated bio-fabrication of silver nanoparticles: characterization, reduction of industrially relevant dyes in water and antimicrobial activities against foodborne pathogens. RSC Adv. 2019;9(65):37957–37970. doi:10.1039/C9RA08618H