ABSTRACT
Volatile organic compounds displayed biological activities on a wide range of organisms, including plants and microbes. Investigating their role in the plant-microbe interaction processes occurring in the soil is challenging. By simulating belowground communication conditions between plant and microbes, in this study, we aimed to investigate the effects of the volatiles emitted by Serratia plymuthica and Fusarium culmorum on the nutrient status of maize plants. Plants were grown in potting soil and exposed to volatiles emitted by microbes inoculated in Petri dishes at the bottom of a jar. Nutrients content of plant tissues as well as soil volatiles were analyzed by ICP-MS and GC-MS, respectively. Our results showed that volatiles emitted belowground by Serratia plymuthica and Fusarium culmorum, in monoculture or interaction, differentially impacted on the content of some nutrient in plants, indicating that microbial volatiles-emitted belowground can affect the nutritional status of plants from a distance.
1. Introduction
Plants are involved in intimate interactions with microbes during their entire life, and these interactions are essential for plant growth and health. To cope with different environmental stresses (biotic and abiotic), plants have developed sophisticated mechanisms, involving the production and exudation/emission of an arsenal of chemical compounds able to activate defence strategies. Such compounds define the communication processes between plants and other organisms in the surrounding environment both above and belowground. In the soil, plant-microbe interactions mainly occur in the rhizosphere, where a variety of microbes are attracted by root exudates (primary and secondary plant metabolites) (van Dam and Bouwmeester Citation2016). The rhizosphere microbiome has important effects on plant health including facilitation in nutrient acquisition, improved abiotic stresses tolerance, plant growth promotion and increased defences against pathogens and insects (Mendes et al. Citation2011; Pieterse et al. Citation2014). Soil microbes are able as well to produce and emit a wide array of volatile and non-volatile compounds (Caulier et al. Citation2019). Recent studies reported that volatile compounds displayed significant biological activities on a wide range of organisms, including plants and microbes (Groenhagen et al. Citation2013; Piechulla et al. Citation2017). Volatile organic compounds (VOCs) are small molecules (<300 Da) with high vapor pressure, low boiling point and containing a lipophilic moiety (Schulz and Dickschat Citation2007). These molecules can spread through the air-filled pores of the soil, allowing long-distance communication among organisms. The chemical structure of VOCs ranges from small aliphatic and aromatic molecules to ketones, alkanes, alkenes and terpenes (Garbeva and Weisskopf Citation2019). The ‘volatilome’ of an organism consists of a mixture of different chemical compounds which composition and abundance depend on the growth stage of the organism (Kai et al. Citation2010; Blom et al. Citation2011a) and, in the case of plants, also on the organ under consideration (e.g. root or leaf).
The different roles of bacterial VOCs on plants have been recently reviewed by Garbeva and Weisskopf (Citation2019). Most studies show a beneficial impact of bacterial VOCs on plant health by promoting a positive effect on plant growth, induce resistance to biotic stresses, and increase tolerance to abiotic stresses. For example, volatiles emitted by some plant-growth-promoting rhizobacteria (PGPR) stimulate plant growth and induce systemic disease resistance against plant pathogens (Ryu et al. Citation2003; Lee et al. Citation2012; Park et al. Citation2015; Raza et al. Citation2016; Tahir et al. Citation2017).
Most of the data showing the beneficial effects of bacterial VOCs on plants have been obtained in artificial environments (Bailly and Weisskopf Citation2012; Liu and Zhang Citation2015). A dual plate system, which relies on the avoidance of direct contact between plant and microbes, is commonly used for testing the effect of microbial volatiles on plant growth (Liu and Zhang Citation2015). However, this in vitro system largely differs from the belowground communication conditions. To overcome this point, experimental systems were established, consisting of plants growing in potting soil and exposed to volatiles emitted to microbes growing in Petri dishes at the bottom of a jar/box (Park et al. Citation2015; Tahir et al. Citation2017; Cordovez et al. Citation2018).
In the present study, we aimed to test the effect of microbial volatiles emitted belowground on the nutritional status of maize plants by using a pot-in-jar system. We tested the ability of volatiles to diffuse freely in soil, by trapping the volatiles in the soils. As model microbes, we used the beneficial bacteria Serratia plymuthica and the plant-pathogenic fungus Fusarium culmorum. Serratia plymuthica PRI-2C is an enterobacterium isolated from maize rhizosphere that shows strong antimicrobial activity against several fungal plant pathogens (Garbeva et al. Citation2014). F. culmorum is one of the most important fungal soilborne plant pathogens that causes diseases on a wide diversity of cereal crops, including maize. When exposed to the volatiles emitted by this fungus, S. plymuthica experimented an increased emission of the volatile terpene sodorifen (Schmidt et al. Citation2017). Our results showed that volatiles emitted belowground by these two microbes in monoculture or interaction affected the content of some nutrients in the maize plants.
2. Material and Methods
2.1. Strains and growth conditions
The bacterial strain S. plymuthica PRI-2C was isolated from rhizosphere of maize plants at different phenological stages and grown in the Wildekamp field, located in Bennekom, The Netherlands (Garbeva et al. Citation2004). The soil in this field was a loamy sand rich in organic matter (2.5%) with slightly acidic pH (5.5–6.5). The S. plymuthica PRI-2C strain was pre-cultured from frozen glycerol stocks on 0.1 Tryptic Soy Broth agar plates (TSB) and grown for three days at 20°C before it was used (Garbeva and De Boer Citation2009). The fungal strain F. culmorum PV was isolated from a sandy dune soil in The Netherlands (De Boer et al. Citation1998), pre-cultured on 0.5 Potato Dextrose Agar plates (PDA) (Fiddaman and Rossall Citation1993) and incubated for five days at 20°C before use.
2.2. Seeds priming
Maize seeds (Zea mays cultivar PR39F58) were surface sterilized and germinated on Petri dishes with moistening filter paper in the dark and at 25°C for two days. Seedlings were primed via volatiles by exposing them to the volatile compounds produced by S. plymuthica PRI-2C, F. culmorum PV, or both in interaction. Seedlings were placed on the lid of a petri dish (10 cm diameter) containing 0.5 Murashige & Skoog (M&S) agar. F. culmorum PV and S. plymuthica PRI-2C were grown on small (3.5 cm) agar plates containing 3 mL 0.5 PDA medium or 1.5% water-agar supplied with artificial root exudates (WA + ARE). The composition of ARE stock solution was as follows: 18.4 mM glucose; 18.4 mM fructose; 9.2 mM saccharose; 4.6 mM citric acid; 9.2 mM lactic acid; 6.9 mM succinic acid; 18.4 mM L-serine; 11 mM L-glutamic acid and 18.4 mM L-alanine (C/N 10.4) according to (Schmidt et al. Citation2016). F. culmorum PV plugs (6 mm in diameter) were inoculated on 0.5 PDA plates. A volume of 150 µl of 107 cells/ml S. plymuthica PRI-2C suspension was inoculated on WA+ARE plates (1.5% water agar supplied with artificial root exudates (ARE)) (Schmidt et al. Citation2016). The seedlings and the small agar plates containing the bacteria and/or the fungus were all placed inside a 120×120 mm square plate. The plate was closed and sealed with parafilm. A control treatment was set up by exposing the seedlings only to agar media. Seeds were primed for 5–6 days in the greenhouse with the following conditions: temperature during the day (16 h): 21°C; temperature during the night: 16°C; an average level of humidity: 60% Rh.
2.3. Effect of microbial volatiles on maize plants
In order to investigate the response of plants to volatiles emitted by F. culmorum and/or S. plymuthica PRI-2C, a pot-in-jar system was used, based on the protocol described in Tahir et al. (Citation2017) with a few modifications (). Small Petri dishes (diameter: 3.5 cm) inoculated with the bacteria, the fungus or both, were placed in the bottom of the jars. A sterile mesh was used to contain the soil (200 g of gamma-sterilized sand) in the pots. Primed seedlings were transferred to the sandy soil and exposed to the microbial volatiles for two weeks in total. Plants were grown in the greenhouse at the above-mentioned conditions, and 10 ml/pot of 0.5 Hoagland solution was added every two days. After a week, small Petri dishes containing the bacterial suspension and the fungal plugs were removed and replaced with new ones. Six replicates for every treatment were used. For the volatile collection in the soil, traps containing 150 mg Tenax TA and 150 mg Carbopack B (Markes International Ltd, Llantrisant, UK) were used. After overnight collection, the Tenax traps were stored at 4°C until subsequent analyses. After two weeks, all the plants were harvested, and growth parameters (whole plant weight, shoot weight, root weight and root length) were determined. ImageJ was used to measure root length.
2.4. Volatiles analysis
Volatile organic compounds were desorbed from the traps using an automated thermal desorption unit (Unity TD-100, Markes International Ltd., UK) at 210°C for 12 min (He flow 50 ml/min) and trapped on a cold trap at −10°C. The volatiles were introduced into a GC-MS-QTOF (model Agilent 7890B GC and the Agilent 7200A QTOF, Santa Clara, USA) by heating the cold trap for 3 min to 280°C. The split ratio was set to 1:5, and the column used was a 30 × 0.25 mm ID RXI-5MS, film thickness 0.25 μm (Restek 13424–6850, Bellefonte, PA, USA). The temperature program was as follows: 39°C for 2 min, from 39°C to 95°C at 3.5°C/min, then to 165°C at 6°C/min, to 250°C at 15°C/min, and finally to 300°C at 40°C/min, hold 20 min. The volatiles were detected by the MS operating at 70 eV in EI mode. Mass spectra were acquired in full-scan mode (30–400AMU, four scans/s) and extracted with MassHunter Qualitative Analysis Software VB.06.00 Build 6.0.633.0 (Agilent Technologies, Santa Clara, USA) using the GC-Q-TOF qualitative analysis module. Statistical analyses were performed using Metaboanalyst 4.0.
2.5. Ionome profile
Shoot, and root samples were oven-dried at 70°C for six days. Dry weight was measured. Sampled tissues were dried and then mineralized in HNO3 by using a Microwave Digestion System (Multiwave ECO). Macronutrients and micronutrients content was determined by Inductively Coupled Plasma-Mass Spectrometry (ICP-MS, aurora M90 BRUKER). The data collected are from three independent biological replicates.
Statistical differences between treatments were determined using one-way ANOVA followed by pairwise post hoc Tukeýs test, in the case of multiple comparisons. For pairwise comparisons T-test has been used. All statistical analysis has been performed using the software Past3.
3. Results and discussion
3.1. Microbial-emitted volatiles belowground affect nutrient content in maize plants
The volatiles emitted by either S. plymuthica and F. culmorum (in monoculture or in interaction) did not significantly affect plant growth after 14 days, even though the shoot biomass of the plants exposed to S. plymuthica´s volatiles slightly increased compared to the control plants (Table S1). However, changes in the nutrient content were detected both in shoot and root of maize plants exposed to the different microbial volatiles.
The PCA performed on the macronutrients content of shoot revealed that the first and second component (PC1 and PC2) explained about 98% of the total variability (PC1:96,5% and PC2: 2.18%) (). The separation observed between the control samples from all treatments along the PC1 indicated that the presence of microbe-emitted volatiles had an impact on the content of macronutrients. Additionally, a separation of the F. culmorum monoculture from the other treatments was evident along the PC2, suggesting that the volatile blend produced by pathogenic F. culmorum affected the macronutrient content in different ways ().
Figure 2. Principal component analysis (PCA) of macronutrients and micronutrients content in both shoot (upper panels) and root (lower panels) tissues of maize plant grown under treatments: C, control; F, F. culmorum in monoculture; S, S. plymuthica in monoculture; SF, both S. plymuthica and F. culmorum in interaction.
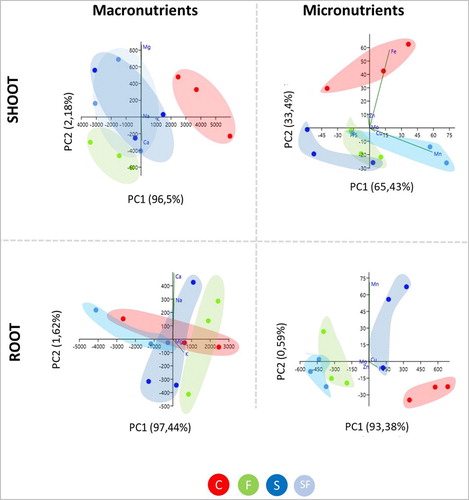
At the root level, the PCA performed on macronutrients content identified two principal components, PC1 and PC2, which explained the 97,44% and the 1,62% of the total observed variance, respectively. However, great variability was observed for each treatment ().
The PCA performed on the micronutrient content in shoot identified two principal components, PC1 and PC2, which explained the 65,43% and 33,4% of the total variance observed, respectively (). The PCA scatterplot revealed a clear separation between the control and the treatments along the PC2, indicating that microbial volatiles affect the micronutrient content in shoot tissues. Furthermore, a separation between the treatments was observed along the PC1, indicating that the volatiles emitted during the interaction of S. plymuthica and F. culmorum provide a different effect compared to the volatiles produced in monoculture. The PCA performed on the micronutrient content of root samples identified two principal components, PC1 and PC2, which explained 99,38% and 0,59% of the observed variance, respectively. The scatterplot revealed a clear separation among all the treatments along the PC1, indicating that the volatiles produced by the different microbes in monoculture and interaction, differentially affected micronutrients content in maize root ().
One-way ANOVA analysis revealed significant differences among the treatments (F, p < 0,05) in K Fe, Zn and Mo content in shoot samples, while significant differences in Mn, Fe and Cu were observed at the root level (). Among the essential macronutrient, K contents decreased in the plants exposed to microbial volatiles (monoculture and interaction) when compared to the control (Tukey's test, p < 0,05) (). Furthermore the content of non-essential nutrients, like Na, was affected in shoot samples of plants exposed to microbial volatiles (monoculture and interaction) when compared to the control (F, p < 0,05) ().
Table 1. Mineral nutrients content (expressed as µg g−1 DW) in shoot and root tissues of maize plants grown in pot-in-jar system under the following treatments: C, control (plant not exposed to volatiles); S, plant exposed to Serratia-emitted volatiles, F, plant exposed to Fusarium-emitted volatiles; SF, plant exposed to both Serratia-emitted and Fusarium – emitted volatiles. Data are shown as the mean (±SE) from three independent biological replicates.
Among the micronutrients, Fe content decreased in the shoot of all maize plants exposed to the microbial volatiles when compared to the control, while plants exposed to F. culmorum volatiles (in monoculture and interaction with S. plymuthica) displayed a lower Fe content at the root level compared to the ones exposed to S. plymuthica alone and in control (). Zinc content decreased in the plants treated with F. culmorum compared to the control only in shoot tissues. By contrast, Cu content decreased in plants exposed to F. culmorum-emitted volatiles only at the root level. Additionally, microbial volatiles differentially affected Mo content mainly at the shoot level (). Among the nutrients considered, only Fe content was affected both in root and shoot, suggesting that Fe homeostasis might be one of the main targets of the VOCs produced by these microbes. Iron deficiency is a nutritional disorder which impairs plant growth. Although, the shoot Fe content decreased about 40%, 35% and 27% in maize plants under S, F and SF treatments respectively, the plant biomass was not affected. Shi et al. (Citation2018) observed that the shoot biomass significantly decreased in B73 and Mo17 maize lines (differing in chlorosis susceptibility) with a decrease of Fe concentration by about 62% and 30% respectively, while small (B73) and any (Mo17) difference in root biomass was observed. Such findings highlight the concept that the critical Fe concentration in plants depends on the genotype. However, such low content of Fe might negatively impact on maize plants later, in a more advanced developmental stage.
Furthermore, plants exposed to volatiles emitted by F. culmorum lead to a content decrease of other nutrients like Zn and Mo in the shoot. Molybdenum variation might be associated with the sulfur status of the plants. Transporters mediating Mo and S uptake in plants belong to the same protein family of SULTR transporters (Bittner Citation2014). Variation of Mo content in plants is associated with the variation of S availability as well as of Fe availability (Courbet et al. Citation2019 and references therein). At the root level, volatiles-emitted by F. culmorum strongly affected also Cu content, which is known to interact with Fe homeostasis in plants (Bernal et al. Citation2012).
The mineral content profile (ionome) of plants is a complex network of nutrients, and an impaired availability of a given element has an impact on the whole ionome profile (Pii et al. Citation2015). Therefore, the changes observed in the nutrient status of plants under different microbial volatiles exposure might result from the nutrient interaction that occurs in plants. It is not possible to identify the direct nutrient target of volatiles blend at this stage.
3.2. Analysis of volatiles in soil
Volatiles were collected from the soil for each treatment using Tenax traps and were analyzed by high-resolution GC/MS. A separation of the volatile metabolites trapped in the different soil treatments was observed in the PLS-DA plot (). Several mass features in the heatmap were only present in some treatments (). Nevertheless, there are no significant differences in the volatile compounds trapped in the treatments and control. It is known that different blends of VOCs are produced by diverse soil microbes in monocultures and interactions (Tyc et al. Citation2015; Schulz-Bohm et al. Citation2017). In soils, the production levels of volatile compounds emitted by microbes are presumed to be low (Insam and Seewald Citation2010) and variations in soil physico-chemical characteristics (for instance, the change of humidity levels when the nutrient solution was added in the pots) can lead to rapid evaporation of volatile compounds resulting in inconsistent outcomes (Ryu Citation2015).
Figure 3. PLS-DA 2D plot of the volatiles collected from the different treatments. C, control; F, F. culmorum in monoculture; S, S. plymuthica in monoculture; SF, both S. plymuthica and F. culmorum in interaction. The explained variance is shown in brackets.
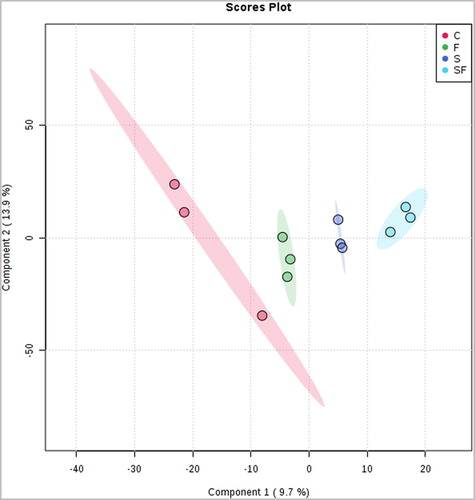
Figure 4. Heatmap showing the hierarchical clustering of the samples based on Euclidean distance. The top 75 mass features for the different treatments ranked by t-tests are shown. Columns represent the samples, in three replicates. Rows represent mass features. Blue, low abundance; Red, high abundance. C, control; F, F. culmorum in monoculture; S, S. plymuthica in monoculture; SF, both S. plymuthica and F. culmorum in interaction.
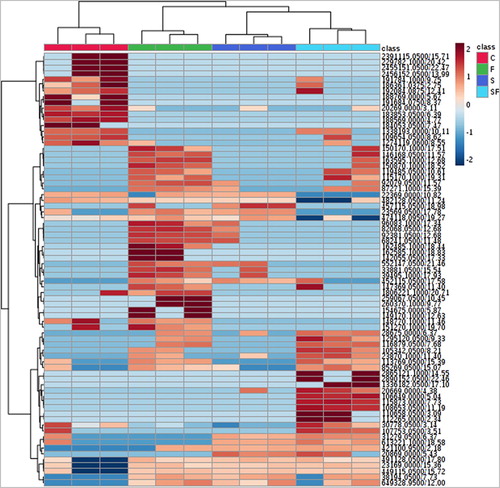
Next to the volatile organic compounds bacteria also emit inorganic volatiles such as nitric oxide (NO), hydrogen sulfide (H2S), ammonia or hydrogen cyanide (HCN) (Audrain et al. Citation2015) which cannot be detected with our trapping system, and that might be responsible for the differences in nutrient content observed in the present study. For example, it is known that NO regulates several processes in plant cells (Moreau et al. Citation2010) as well as Fe homeostasis (Chen et al. Citation2010). Furthermore, it has been reported that inorganic volatile compounds affect plant and microbe growth. For instance, the volatile compound HCN, produced by Pseudomonas spp., Rhizobium spp., Chromobacterium violaceum and S. plymuthica IC14, has a direct inhibitory effect on plants, fungi and bacteria (Castric et al. Citation1981; Antoun et al. Citation1998; Blumer and Haas Citation2000; Blom et al. Citation2011b).
4. Conclusion
The interactions among soil organisms contribute to the proper functioning of an ecosystem. However, the role of belowground VOCs on plant nutritional status is largely underexplored.
An evident variation in the nutrient content of the plants was observed in response to microbial VOCs. The content of nutrients like, K, Fe, Zn and Mo was altered in all treatments compared to the control plants, indicating that the different volatile blends have an impact on the nutrient status of the plants. Particularly, volatiles emitted by S. plymuthica and F. culmorum affected the content of micronutrients, such as Fe, Zn, Cu and Mo.
Overall, such findings highlight that microbial volatiles emitted belowground can affect the nutritional status of plants from the distance.
Further efforts should be directed to investigate the effect of microbial volatiles emitted belowground on plants in order to understand better the communication mechanisms occurring in the soil.
Supplemental Material
Download TIFF Image (246.5 KB)Supplemental Material
Download MS Word (12.6 KB)Acknowledgement
PG, LMS and GV conceived and designed the study. LMS, CA and GV performed the experiments. GV wrote the manuscript. LMS and CA revised the manuscript. PG revised critically the manuscript. All the authors approved the final version of the article.
Disclosure statement
No potential conflict of interest was reported by the author(s).
Additional information
Funding
Notes on contributors
Lara Martín-Sánchez
Lara Martin-Schanzez, since 2017 is a Postdoctoral researcher in microbial ecology at NIOO-KNAW, Wageningen.
Cristiana Ariotti
Cristiana Ariotti, since 2019 is a PhD student in Pharmaceutical and Biomolecular Sciences at University of Turin.
Paolina Garbeva
Paolina Garbeva is senior research scientist and group leader at NIOO-KNAW, Wageningen.
Gianpiero Vigani
Gianpiero Vigani is senior research scientist at University of Turin (since 2017) and guest researcher at NIOO-KNAW, Wageningen (since 2019).
References
- Antoun H, Beauchamp CJ, Goussard N, Chabot R, Lalande R. 1998. Potential of Rhizobium and Bradyrhizobium species as plant growth promoting rhizobacteria on non-legumes: effect on radishes (Raphanus sativus L.). In: Hardarson G, Broughton WJ, editors. Molecular microbial ecology of the soil. Developments in Plant and Soil Sciences. Vol. 83. Dordrecht: Springer; p. 57–67.
- Audrain B, Farag MA, Ryu CM, Ghigo JM. 2015. Role of bacterial volatile compounds in bacterial biology. FEMS Microbiol Rev. 39(2):222–233. doi:10.1093/femsre/fuu013.
- Bailly A, Weisskopf L. 2012. The modulating effect of bacterial volatiles on plant growth: current knowledge and future challenges. Plant Signal Behav. 7(1):79–85. doi:10.4161/psb.7.1.18418.
- Bernal M, Casero D, Singh V, Wilson GT, Grande A, Yang H, & Merchant SS. 2012. Transcriptome sequencing identifies SPL7-regulated copper acquisition genes FRO4/FRO5 and the copper dependence of iron homeostasis in Arabidopsis. Plant Cell. 24(2):738–761. doi:10.1105/tpc.111.09043.
- Bittner F. 2014. Molybdenum metabolism in plants and crosstalk to iron. Front Plant Sci. 5:28. doi:10.3389/fpls.2014.00028.
- Blom D, Fabbri C, Connor EC, Schiestl FP, Klauser DR, Boller T, … Weisskopf L. 2011a. Production of plant growth modulating volatiles is widespread among rhizosphere bacteria and strongly depends on culture conditions. Environ Microbiol. 13(11):3047–3058. doi:10.1111/j.1462-2920.2011.02582.x.
- Blom D, Fabbri C, Eberl L, Weisskopf L. 2011. Volatile-mediated killing of Arabidopsis thaliana by bacteria is mainly due to hydrogen cyanide. Appl Environ Microbiol 77(3):1000–1008. doi:10.1128/AEM.01968-10.
- Blumer C, Haas D. 2000. Mechanism, regulation, and ecological role of bacterial cyanide biosynthesis. Arch Microbiol. 173(3):170–177. doi:10.1007/s002039900127.
- Castric PA, Castric KF, Meganathan R. 1981. Factors influencing the termination of cyanogenesis in Pseudomonas aeruginosa. In: Vennesland B, Conn EE, Knowles CJ, Westley J, Wissing F, editors. Cyanide in Biology. London: Academic Press; p. 263–274.
- Caulier S, Nannan C, Gillis A, Licciardi F, Bragard C, Mahillon J. 2019. Overview of the antimicrobial compounds produced by members of the Bacillus subtilis group. Front Microbiol. 10:302. doi:10.3389/fmicb.2019.00302.
- Chen WW, Yang JL, Qin C, Jin CW, Mo JH, Ye T, Zheng SJ. 2010. Nitric oxide acts downstream of auxin to trigger root ferric-chelate reductase activity in response to iron deficiency in Arabidopsis. Plant Physiol. 154(2):810–819. doi:10.1104/pp.110.161109.
- Cordovez V, Schop S, Hordijk K, de Boulois HD, Coppens F, Hanssen I, … Carrion VJ. 2018. Priming of plant growth promotion by volatiles of root-associated Microbacterium spp. Appl Environ Microbiol 84(22):e01865–18. doi:10.1128/AEM.01865-18.
- Courbet G, Gallardo K, Vigani G, Brunel-Muguet S, Trouverie J, Salon C, Ourry A. 2019. Disentangling the complexity and diversity of crosstalk between sulfur and other mineral nutrients in cultivated plants. J Exp Bot. 70(16):4183–4196. doi:10.1093/jxb/erz214.
- De Boer W, Gunnewiek PJK, Lafeber P, Janse JD, Spit BE, Woldendorp JW. 1998. Anti-fungal properties of chitinolytic dune soil bacteria. Soil Biol Biochem. 30(2):193–203. doi:10.1016/S0038-0717(97)00100-4.
- Fiddaman PJ, Rossall S. 1993. The production of antifungal volatiles by Bacillus subtilis. J Appl Bacteriol. 74(2):119–126. doi:10.1111/j.1365-2672.1993.tb03004.x.
- Garbeva P, De Boer W. 2009. Inter-specific interactions between carbon-limited soil bacteria affect behavior and gene expression. Microb Ecol. 58(1):36–46. doi:10.1007/s00248-009-9502-3.
- Garbeva P, Hordijk C, Gerards S, de Boer W. 2014. Volatile-mediated interactions between phylogenetically different soil bacteria. Front Microbiol. 5:289. doi:10.3389/fmicb.2014.00289.
- Garbeva P, van Veen JA, van Elsas JD. 2004. Assessment of the diversity, and antagonism towards Rhizoctonia solani AG3, of Pseudomonas species in soil from different agricultural regimes. FEMS Microbiol Ecol. 47(1):51–64. doi:10.1016/S0168-6496(03)00234-4.
- Garbeva P, Weisskopf L. 2019. Airborne medicine: bacterial volatiles and their influence on plant health. New Phytol. 226(1):32–43. doi:10.1111/nph.16282.
- Groenhagen U, Baumgartner R, Bailly A, Gardiner A, Eberl L, Schulz S, Weisskopf L. 2013. Production of bioactive volatiles by different Burkholderia ambifaria strains. J Chem Ecol. 39(7):892–906. doi:10.1007/s10886-013-0315-y.
- Insam H, Seewald MS. 2010. Volatile organic compounds (VOCs) in soils. Biol Fertil Soils. 46(3):199–213. doi:10.1007/s00374-010-0442-3.
- Kai M, Crespo E, Cristescu SM, Harren FJ, Francke W, Piechulla B. 2010. Serratia odorifera: analysis of volatile emission and biological impact of volatile compounds on Arabidopsis thaliana. Appl Microbiol Biotechnol. 88(4):965–976. doi:10.1007/s00253-010-2810-1.
- Lee B, Farag MA, Park HB, Kloepper JW, Lee SH, Ryu CM. 2012. Induced resistance by a long-chain bacterial volatile: elicitation of plant systemic defense by a C13 volatile produced by Paenibacillus polymyxa. PloS one. 7:11. doi:10.1371%2Fjournal.pone.0048744.
- Liu XM, Zhang H. 2015. The effects of bacterial volatile emissions on plant abiotic stress tolerance. Front Plant Sci. 6:774. doi:10.3389/fpls.2015.00774.
- Mendes R, Kruijt M, De Bruijn I, Dekkers E, van der Voort M, Schneider JH, Piceno YM, DeSantis TZ, Andersen GL, Bakker PA, Raaijmakers JM. 2011. Deciphering the rhizosphere microbiome for disease-suppressive bacteria. Science. 332(6033):1097–1100. doi:10.1126/science.1203980.
- Moreau M, Lindermayr C, Durner J, Klessig DF. 2010. NO synthesis and signaling in plants–where do we stand? Physiol Plant. 138(4):372–383. doi:10.1111/j.1399-3054.2009.01308.x.
- Park YS, Dutta S, Ann M, Raaijmakers JM, Park K. 2015. Promotion of plant growth by Pseudomonas fluorescens strain SS101 via novel volatile organic compounds. Biochem Biophys Res Commun. 461(2):361–365. doi:10.1016/j.bbrc.2015.04.039.
- Piechulla B, Lemfack MC, Kai M. 2017. Effects of discrete bioactive microbial volatiles on plants and fungi. Plant Cell Environ. 40(10):2042–2067. doi:10.1111/pce.13011.
- Pieterse CM, Zamioudis C, Berendsen RL, Weller DM, Van Wees SC, Bakker PA. 2014. Induced systemic resistance by beneficial microbes. Annu Rev Phytopathol. 52:347–375. doi:10.1146/annurev-phyto-082712-102340.
- Pii Y, Cesco S, Mimmo T. 2015. Shoot ionome to predict the synergism and antagonism between nutrients as affected by substrate and physiological status. Plant Physiol Biochem. 94:48–56. doi:10.1016/j.plaphy.2015.05.002.
- Raza W, Yousaf S, Rajer FU. 2016. Plant growth promoting activity of volatile organic compounds produced by biocontrol strains. Science Letters. 4(1):40–43.
- Ryu CM. 2015. Bacterial volatiles as airborne signals for plants and bacteria. In: Lugtenberg B, editor. Principles of plant-microbe interactions. Cham: Springer; p. 53–61.
- Ryu CM, Farag MA, Hu CH, Reddy MS, Wei HX, Paré PW, Kloepper JW. 2003. Bacterial volatiles promote growth in Arabidopsis. Proc Natl Acad Sci U S A. 100(8):4927–4932. doi:10.1073/pnas.0730845100.
- Schmidt R, de Jager V, Zühlke D, Wolff C, Bernhardt J, Cankar K, … Riedel K. 2017. Fungal volatile compounds induce production of the secondary metabolite sodorifen in Serratia plymuthica PRI-2C. Sci Rep. 7(1):1–14. doi:10.1038/s41598-017-00893-3.
- Schmidt R, Etalo DW, de Jager V, Gerards S, Zweers H, de Boer W, Garbeva P. 2016. Microbial small talk: volatiles in fungal–bacterial interactions. Front Microbiol. 6:1495. doi:10.3389/fmicb.2015.01495.
- Schulz-Bohm K, Martin-Sanchez L, Garbeva P. 2017. Microbial volatile: small molecules with an important role in intra-and Inter-kingdom interactions. Front Microbiol. 8:2484. doi:10.3389/fmicb.2017.02484.
- Schulz S, Dickschat JS. 2007. Bacterial volatiles: the smell of small organisms. Nat Prod Rep. 24(4):814–842.
- Shi R, Melzer M, Zheng S, Benke A, Stich B, Von Wirén N. 2018. Iron Retention in root Hemicelluloses causes Genotypic variability in the tolerance to Iron deficiency-Induced chlorosis in maize. Front Plant Sci. 9:557. doi:10.3389/fpls.2018.00557.
- Tahir HAS, Gu Q, Wu H, Niu Y, Huo R, Gao X. 2017. Bacillus volatiles adversely affect the physiology and ultra-structure of Ralstonia solanacearum and induce systemic resistance in tobacco against bacterial wilt. Sci Rep. 7(1):1–15. doi:10.1038/srep40481.
- Tyc O, Zweers H, de Boer W, Garbeva P. 2015. Volatiles in inter-specific bacterial interactions. Front Microbiol. 6:1412. doi:10.3389/fmicb.2015.01412.
- van Dam NM, Bouwmeester HJ. 2016. Metabolomics in the rhizosphere: tapping into belowground chemical communication. Trends Plant Sci. 21(3):256–265. doi:10.1016/j.tplants.2016.01.008.