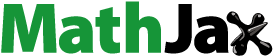
ABSTRACT
Bacterial 1-aminocyclopropane-1-carboxylate (ACC) deaminase is a key factor for alleviating the plant ethylene biosynthesis, which is induced by stress. The ACC deaminase-improved strains of the rice endophytic Bradyrhizobium sp. SUTN9-2, SUTN9-2 (ACCDadap) and SUTN9-2:pMG103::acdRS, exhibit 1.4- and 8.9-fold higher ACC deaminase activity than the wild type, respectively (Sarapat S, Songwattana P, Longtonglang A, Umnajkitikorn K, Girdthai T, Tittabutr P, Boonkerd N, Teaumroong N. 2020. Effects of Increased 1-Aminocyclopropane-1-Carboxylate (ACC) Deaminase Activity in Bradyrhizobium sp. SUTN9-2 on Mung Bean Symbiosis under Water Deficit Conditions. Microbes Environ. 35). The effects of these on rice growth under water deficit conditions were evaluated. The bacterial inoculations reduced ethylene synthesis, leading to a reduction in membrane destruction and the chlorophyll content of rice. Furthermore, the bacterial inoculations improved the leaf relative water content, survival, recovery rates, and improved the crop yield in field conditions. Therefore, the water deficit tolerance of rice was improved by controlling ethylene biosynthesis by improving ACC deaminase activity with endophytic SUTN9-2. Moreover, the SUTN9-2 (ACCDadap) strain can be used as a bio-inoculant under field conditions to enhance rice growth, grain yield, and enhance drought tolerance.
Introduction
Drought is a major limiting factor in rice production (Farooq et al. Citation2009). Generally, under water deficit, plants suffer changes in their morphological, physiological, biochemical, and molecular responses, which ultimately result in yield reduction (Blum Citation1996; Pandey and Shukla Citation2015; Ullah et al. Citation2017). Briefly, plants respond to water deficiency in the soil by root perception before sending corresponding signals to other parts of the plant, particularly the leaves. To decrease water loss, plants undergo stomatal closure. However, this phenomenon is a cause of reduced photosynthesis, leading to increased photorespiration and the accumulation of reactive oxygen species (ROS), which damage the cell membrane (lipid peroxidation) (Ahmad Citation2016). Furthermore, plant hormones play an important role as signaling molecules in plant physiological responses to unfavorable conditions (Bleecker and Kende Citation2000; Ahmad and Prasad Citation2011; Abeles et al. Citation1992; Dubois et al. Citation2018). One plant hormone, ethylene, plays a vital role in plant survival when plants encounter with unfavorable conditions by stimulating adaptive mechanisms (Wang et al. Citation2002; Druege Citation2006). Ethylene production in plants elevates a few hours after exposure to unfavorable environmental conditions, and at low concentrations initiates plant defense mechanisms (Wang et al. Citation2002; Druege Citation2006; Glick et al. Citation2007). However, the hormone can have negative effects at high concentrations. Nevertheless, ethylene production can be decreased by controlling its substrate, 1-Aminocyclopropane-1-carboxylic acid (ACC), which is rate-limiting in ethylene production (Honma and Shimomura Citation1978; Yu et al. Citation1979; Apelbaum and Yang Citation1981; Glick et al. Citation2007). Microorganisms containing ACC deaminase can catalyze the cleavage and deamination of ACC to form ammonia and α-ketobutyrate (Honma and Shimomura Citation1978; Glick et al. Citation1998), which are utilized as a nitrogen and a carbon source by the microorganism, respectively (Van de Poel and Van Der Straeten Citation2014; Vanderstraeten and Van Der Straeten Citation2017). These microorganisms have the potential to reduce ACC contents in plant cells and reduce stress to the plant from ethylene. The ACC deaminase enzyme is encoded from the acdS gene and is controlled by the leucine-responsive regulatory (Lrp) protein, encoded from the acdR gene; this property is classified as a trait of plant growth-promoting bacteria (Grichko and Glick Citation2000; Li and Glick Citation2001; Glick et al. Citation2007).
Rice (Oryza sativa L.) is the staple food and a major economic crop of many Asian countries, including Thailand. The main rice production area in Thailand is around 5.6 million hectares, situated in the northeastern region, and approximately 74.6% of this region is outside of the irrigation area (Rice Department Citation2016). Based on these data, it is implied that half of the rice is cultivated in rain-fed farming systems. However, productivity is generally related to the amount and distribution of rainfall, which means rice production in Thailand this is at risk from adverse climate conditions due to climate change, especially increased heat and drought. Therefore, it is of interest to apply the strategy of the bacteria containing ACC deaminase activity that are associated with rice plants which encounter delayed rainfall or are distributed outside of the irrigated areas in northeast Thailand. Previous studies demonstrated that endophytic bacteria containing ACC deaminase activity enhanced plant tolerances to stresses or alleviated deterioration when their host was exposed to environmental stress (Hardoim et al. Citation2008; Santoyo et al. Citation2016). In maize seedlings under drought stress conditions, ACC deaminase producing Burkholderia phytofirmans PsJN and Enterobacter sp. FD17 promoted the biomass, photosynthesis, and photochemical efficiency of photosystem II (PSII) in the seedlings when compared with uninoculated seedlings (Sun et al. Citation2009; Naveed et al. Citation2014a; Naveed et al. Citation2014b). Furthermore, both endophytes improved the leaf relative water content and decreased the membrane permeability of the inoculated maize seedlings.
However, these studies mostly focused on salinity stress, while the effects of water deficit or drought received less attention in rice production. Recently, rice endophytic Bradyrhizobium sp. SUTN9-2 was used to investigate growth promotion in rice seedlings; SUTN9-2 (wild type strain) was used as a bio-inoculant to promote rice growth at the seedling stage (seven and 14 days after inoculation) in N-free and with NH4NO3 in vivo conditions (Greetatorn et al. Citation2019). In addition, our previous study on SUTN9-2 showed it improved the ACC deaminase activity through enhancing the symbiotic interaction, plant biomass and drought tolerance in the mung bean (Sarapat et al. Citation2020). We hypothesized that the improved ACC deaminase activity strains, SUTN9-2 (ACCDadap) and SUTN9-2:pMG103::acdRS, which contain high ACC deaminase activity that is 1.4 and 8.9 fold higher than the wild type, respectively, may provide beneficial effects to rice plants under water deficit conditions. The ACC deaminase activities of both strains were improved by different strategies, including adaptive laboratory evolution and genetically modified organism approaches (Sarapat et al. Citation2020). In this study, we examined the potential of using rice endophytes to promote rice growth under water deficit and well-watered treatments under laboratory, greenhouse, and field conditions.
Materials and methods
Bacterial strains and inoculant preparations
Bradyrhizobium sp. SUTN9-2 (Piromyou et al. Citation2015b) and its ACC deaminase-improved strains from a previous study, SUTN9-2 (ACCDadap) and SUTN9-2:pMG103::acdRS (Sarapat et al. Citation2020), were grown in 100 ml HEPES-MES Salt medium (HM) (Cole and Elkan Citation1973; Kanbe et al. Citation2007) broth at 30°C, with shaking at 200 rpm for seven days prior to use. The medium for the growth of the SUTN9-2:pMG103::acdRS strain was HM supplemented with 20 μg/ml cefotaxime. After seven days, the bacterial culture was washed twice with minimal medium (Rhizobium mimosine (RM) media) (Soedarjo et al. Citation1994; Tittabutr et al. Citation2008) by centrifugation at 8233 × g 25°C for 15 min, and then induced with 3.0 mM ACC in minimal media, which contained 10% (v/v) of HM medium, for 24 h at 30°C, 200 rpm (Siddikee et al. Citation2011). Finally, the induced bacterial culture was collected and washed twice with 0.03 M MgSO4 at 8233 × g, 25°C for 15 min. Before the inoculation, the bacterial suspension was adjusted to the same optical density (OD600nm = 1.0).
Plant materials and growth conditions
Rice plants (Oryza sativa L. var. indica cv. Pathumthani 1) were obtained from Lopburi Rice Seed Center, Thailand. The seeds were surface sterilized with 95% (v/v) ethanol for 5 min followed by 3% (v/v) sodium hypochlorite for 10 min, then washed 2–3 times in sterile distilled water and were washed again 5–10 times in sterile distilled water, followed by soaking in sterile distilled water for 48 h. The seeds were germinated on sterilized wet germination paper for two days at room temperature. The seedlings were soaked in each bacterial suspension (SUTN9-2 wild type, SUTN9-2 (ACCDadap) and SUTN9-2:pMG103::acdRS), while a control treatment (uninoculated plants) was soaked in 0.03 M MgSO4 before planting. In the laboratory experiments, the inoculated and uninoculated seedlings (three plants per tube) were transferred and grown in pots (8 cm diameter and 17 cm depth), which contained a sterilized soil and sand mixture (1:1, v/v) as planting material, and were kept under controlled conditions of white fluorescent lighting (approximately 300 µE m−2 S−1) at 28 ± 2°C, 60% relative humidity, and a 12 h light/dark cycle. The physical and chemical parameters of the soil are summarized in Table S1. Plants were watered with Hoagland nutrient solution (Mae and Ohira Citation1981).
The greenhouse experiments was performed at Suranaree University of Technology Farm (SUT farm; latitude 14°88ʹ83.49″N, longitude 102°00ʹ42.47″), Nakhon Ratchasima, Thailand. The rice seedlings (10 plants per pot) were planted in plastic pots (30 cm diameter and 23 cm depth) containing 8 kg of the sterilized soil/sand mixture described for the laboratory experiment. The pots were placed in a completely randomized design in a factorial arrangement with five replicate pots. The rice plants were grown at 23 ± 2°C day/19 ± 2°C night, with 82% average relative humidity under natural light. Rice seedlings were irrigated with tap water.
In the third experiment, the rice seedlings inoculated with each bacterial strain were tested in field conditions at the SUT farm (latitude 14°87ʹ79.71″, longitude 102°00ʹ69.34″) during dry season (November to February). A randomized complete block design (three replications) was used in this experiment, and the same soil used in the laboratory and greenhouse experiments was used. The size of the experimental plot was 100×300 cm, which contained three rows that were separated by 25 cm. Each plant was spaced 20 cm apart in the rows.
Water deficit and recovery treatments
Fourteen days after inoculation (DAI), the rice seedlings were exposed to water deficit stress. In the laboratory and greenhouse experiments, the supplementation of Hoagland nutrient solution was stopped until the seedlings showed leaf wilting and the water in the pot was removed. The water stress treatment in the field experiment was performed at the tillering stage by withholding tap water. Leaf wilting or leaf rolling in the uninoculated plants was used as a sign of water deficit stress. Watering was recommenced after all treatments displayed leaf wilting, where the Hoagland nutrient solution was supplied to the rice seedlings in the laboratory experiment, while plants in the greenhouse and field experiments were recovered with water. Moreover, the rice plants in the field experiment were further grown in under well-watered conditions until the maturity stage.
Determination of ethylene emissions
Bacteria-inoculated and uninoculated seedlings (three plants per test tube) were transferred and grown in a glass test tube (15 × 150 mm) as described by Greetatorn et al. (Citation2019). At 14 DAI, water stress was induced in the glass test tubes by replacing the Hoagland nutrient solution with a nutrient solution containing polyethylene glycol (PEG8000) (Santa Cruz Biotechnology, Inc., USA). The tubes were tightly closed with a rubber septum prior to sealing them with parafilm. After five days of incubation at 25°C under the laboratory conditions described above, the production of ethylene was determined by the method described in Fukao et al. (Citation2006). Briefly, the 5 ml of headspace air of each tube was injected into a gas chromatograph (GC) (Model 310, SRI Instruments Ltd., UK) equipped with a flame ionization detector (6′ × 1/8″ SS column; Valco Instruments Co. Inc). The PEG solution was provided at −5.11 bars, with the osmotic pressure (OP) of the PEG 8000 solution calculated using the following equation of Michel (Citation1983): OP = 1.29×C2×T − 140×C2 − 4.0×C (where C = PEG concentration and T = temperature). In the greenhouse experiment, the rice seedlings (14 DAI) were exposed to water deficit stress for 20 days (until 34 DAI) and showed the visible wilting. The rice plants were then incubated in a 20.32 × 30.48 cm2 vacuum plastic bag (1 plant per bag) and were vacuum-packed by a vacuum packing machine. The vacuumed sample bags were incubated for three days under light as explained above for the PEG stress induction. The ethylene emission was determined by injecting 5 ml of airspace from each sample bag into a GC (Tittabutr et al. Citation2013). The ethylene emission of each sample was calculated per one milliliter and presented as pmol mg−1 fresh weight day−1 compared with the standard curve generated with pure ethylene. Five replicates were used for each treatment.
Determination of malondialdehyde concentrations
The concentration of malondialdehyde (MDA) was determined using the thiobarbituric acid (TBA) reaction. Briefly, the rice samples (14 DAI) were water stressed by PEG for five days (until 19 DAI) according to ethylene induction conditions described above. The whole leaves of the rice seedlings (25 mg) were ground by a mortar and liquid nitrogen with a pestle until the plant sample was a fine powder. The powder was continuously transferred into a 50 ml tube for mixing with 5 ml of 50 mM sodium phosphate buffer (pH 7.5) and was then centrifuged at 24,700 × g for 15 min. The supernatant (1 ml) was collected to react with 4 ml of 20% trichloroacetic acid containing 0.5% TBA. The reaction mixture was incubated at 95°C for 30 min and the reaction was stopped by cooling in an ice bath and was then centrifuged at 16,467 × g for 10 min (Du and Bramlage Citation1992; Hodges et al. Citation1999). The MDA content was recorded at 532 and 600 nm (nonspecific turbidity) and was calculated by comparing it with a standard curve generated from pure MDA (Sigma-Aldrich, USA). The MDA concentration was determined by the following formula:
Five replicates were used for each treatment. The protein content of the leaves was determined by following Lowry’s method (Lowry et al. Citation1951).
Leaf relative water content
The leaf relative water content (RWC) measurement of rice seedling was performed after rice seedlings (14 DAI) exposed to water deficit for 5 days (19 DAI). The rice plant leaves were cut by a third (10 cm length) and the fresh weight was measured before the leaves were soaked in a petri dish containing double-distilled water at 4°C for 24 h in darkness. The fully turgid weight of the soaked leaves was measured while the dry weight was determined after drying the leaves at 70°C for 72 h (Naveed et al. Citation2014a). These were used for preparing all treatments of rice. Five replicates were used for each treatment, and the RWC was calculated according to Teulat et al. (Citation2003) as:
Chlorophyll a, chlorophyll b, and carotenoid contents in leaves
Measurements of the photosynthetic pigment chlorophyll were performed after rice seedlings (at 14 DAI) were exposed to water deficit stress for five days (until 19 DAI). The whole rice leaf of from both the well-watered and water stressed rice (three replicates) were used for measuring the pigment content (Lichtenthaler Citation1987). Briefly, the leaf samples were lyophilized with a freeze drier, and then ground in a mortar with a pestle and liquid nitrogen. The fine powder was transferred into a test tube supplemented with 96% (v/v) ethanol followed with vortex. The extracted solutions were incubated at room temperature for 16 h and then centrifuged at 21,407 × g for 1 min at 4°C. The supernatants were used for the estimation of chlorophyll a and chlorophyll b, and carotenoid contents using a spectrophotometer at 664.2, 648.6, and 470 nm, respectively. The pigment content was expressed in terms of mg−1 plant dry weight (DW). The concentration of each pigment content was calculated according to the following equations:
Rice survival rate and drought scoring
After the rice seedlings (14 DAI) were exposed to water stress for five days (19 DAI), the seedlings were assessed for survival rate after recommencing watering for three more days. The re-watering of rice plants was conducted in the laboratory and greenhouse experiments. However, in the greenhouse, the survival rate was not assessed; instead, plants were evaluated by the scoring system defined by the Standard Evaluation System for rice (IRRI Citation1996). The scoring system was divided into the drought resistance, drought recovery score, and leaf rolling score, the details of which are described in Table S2 (IRRI Citation1996). Five replicates of each treatment were used.
Rice growth and yield
In the laboratory experiment, the growth parameters including plant dry weight and plant fresh weight were recorded (with five replicates from each treatment) at 14 DAI under well-watered conditions. For the dry weight estimations, the plant samples were dried at 70°C for three days. Moreover, the plant dry weights of the rice in the field experiment were measured in both the seedling and tillering stages, and their tiller numbers were counted. After the rice plants were harvested in the field experiment, the number of seeds and infertile grains, grain yield, and 100-grain weight were measured. Ten replicates were used from each treatment.
Statistical analysis
The data were normalized prior to the analysis. Each parameter was analyzed by a one-way analysis of variance in the Statistical Package of the Social Sciences (SPSS) program version 16.0 (SPSS Inc., US). Significant differences of the means of each treatment were compared by Duncan’s multiple range tests at p ≤ 0.05 (Duncan Citation1957). The data are presented as means ± S.E. (standard error of the mean).
Results
Effects of ACC deaminase on plant growth under well-watered conditions in the laboratory
The fresh weight of the bacteria-inoculated rice seedlings at 14 DAI was significantly greater than that of the uninoculated seedlings (control), which was the same for the shoot and root fresh weights (A–C) and the dry weights of the shoots and roots (D–F). However, the rice inoculated with SUTN9-2:pMG103::acdRS had significantly higher plant dry weight and shoot dry weight than SUTN9-2 WT and SUTN9-2 (ACCDadap) (D and E).
Figure 1. The average (A) plant fresh weight, (B) shoot fresh weight, (C) root fresh weight, (D) plant dry weight, (E) shoot dry weight, and (F) root dry weight of rice seedlings at 14 days after inoculation (DAI) with the different bacterial strains SUTN9-2 WT, SUTN9-2 (ACCDadap), and SUTN9-2:pMG103::acdRS. These were compared with uninoculated plants under well-watered conditions in the laboratory. Significant differences between treatments are indicated by different letters at P ≤ 0.05 according to Duncan’s multiple range tests. The data are presented as the mean of five replicates and the vertical bars indicate the standard error.
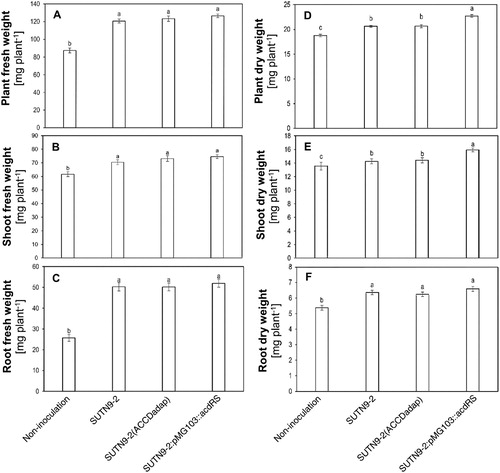
Effects of improved ACC deaminase activity on ethylene production and malondialdehyde concentrations in the laboratory
In the laboratory experiment, only small concentrations of ethylene emissions were and there was no significant differences between the inoculated plants compared with the uninoculated plants when water was not withheld (A). The water stress condition induced by PEG significantly increased the ethylene production in rice. However, the ethylene emissions were reduced around 0.05-fold in rice seedlings inoculated with SUTN9-2 WT and SUTN9-2 (ACCDadap), while SUTN9-2:pMG103::acdRS decreased the ethylene emission around 1.0-fold or 0.85-fold when compared with uninoculated rice and SUTN9-2 WT strain inoculated rice, respectively (A).
Figure 2. The effects of polyethylene glycol (PEG-8000) on (A) ethylene emissions and (B) malondialdehyde (MDA) content in rice seedlings either inoculated with bacteria or uninoculated at 19 days after inoculation under laboratory conditions. Water stressed rice were compared with well-watered rice. Significant differences between treatments are indicated by different letters at P ≤ 0.05 according to Duncan’s multiple range tests. The data are presented as the mean of five replicates and the vertical bars indicate the standard error.
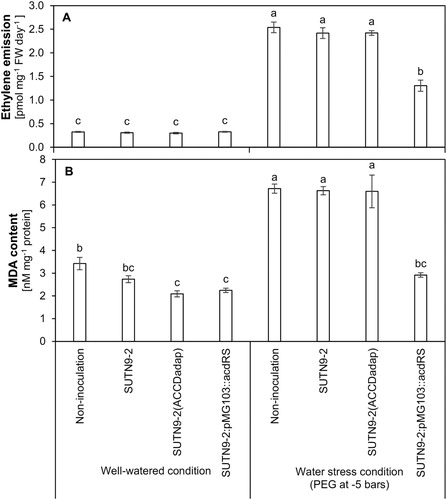
The MDA concentration was used to estimate the lipid peroxidation in the leaves of the rice seedlings when exposed to water deficit stress. The MDA content in rice was significantly higher in the water deficit condition than when rice were well-watered (B). When water was not withheld, there were no significant differences in the MDA contents among the inoculated treatments compared to the uninoculated control, while the rice inoculated with bacteria from the two improved ACC deaminase activity strains had reduced MDA when compared with the uninoculated plants. In addition, under water deficit stress conditions, the MDA contents of the rice leaves was reduced by around 1.3-fold after inoculation with SUTN9-2:pMG103::acdRS compared with the uninoculated plants (B).
Effects of the improved ACC deaminase activity on the recovery efficiency, survival rate and relative water content in the laboratory
After five days without the addition of the nutrient solution, the rice seedlings showed wilting symptoms in all treatments, especially in the uninoculated control (A). However, after re-watering for three days, the rice recovered, which was observed from changes to the plant morphology and/or their survival rates (A and B). The survival rate of rice seedlings inoculated with SUTN9-2 WT, SUTN9-2 (ACCDadap), and SUTN9-2:pMG103::acdRS increased significantly by around 0.3–0.4-fold, respectively, when compared with uninoculated rice. The water deficit condition affected the leaf RWC after four days of the draining in the uninoculated plants (C), whereas the rice seedlings inoculated with the endophytes could maintain the percentage of leaf RWC during the soil drying period. The water deficit severely reduced the leaf RWC five days after the water was drained in all treatments, with RWC values around 25.6% in uninoculated plants, 42.5% in SUTN9-2 WT plants, 54.0% in SUTN9-2 (ACCDadap) plants, and 55.4% in SUTN9-2:pMG103::acdRS plants (C), which corresponded with their phenotype (A). The RWC of the leaves also increased in rice inoculated with SUTN9-2, SUTN9-2 (ACCDadap), and SUTN9-2:pMG103::acdRS by 70.2%, 76.0%, and 76.2%, respectively, after 24 h, when compared with uninoculated rice (C).
Figure 3. The effects of water deficit stress and re-watering on (A) rice seedling morphology, (B) survival rate (%),(C) the relative water content (RWC) of leaves (%), (D) chlorophyll a, chlorophyll b and carotenoid contents, and (E) the chlorophyll a/b ratio in rice seedlings either inoculated with bacteria or uninoculated and grown in the soil and sand mixture (1:1) under laboratory conditions. The water deficit stress plants were compared with the well-watered plants. Significant differences between treatments are indicated by different letters at P ≤ 0.05 according to Duncan’s multiple range tests. The data are presented as the mean of three replicates and the vertical bars indicate the standard error. The statistical comparison was performed separately for the well-watered and water stress conditions.
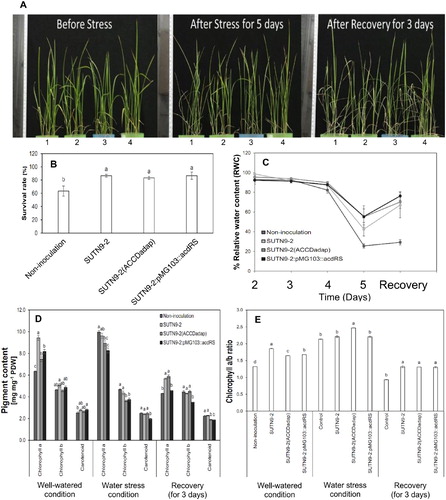
Effects of improved ACC deaminase activity on photosynthetic pigment production in the laboratory
The effects of water deficit stress on the photosynthetic pigments of rice seedlings (at 14 DAI) inoculated with SUTN9-2 and its improved strains are depicted in D–E. The amounts of chlorophyll a in rice inoculated with bacteria were significantly greater than in uninoculated rice. There were no significant differences in the amounts of chlorophyll b and carotenoids in inoculated compared with uninoculated rice under well-watered conditions (D). Under water deficit conditions, rice with the bacterial inoculations had decreased chlorophyll a and chlorophyll b, and carotenoid production; these were not significantly different when compared with uninoculated rice, with the exception of the chlorophyll b content in SUTN9-2 (ACCDadap) and SUTN9-2:pMG103::acdRS inoculated rice, which were significantly lower than in the uninoculated rice. The amount of carotenoids was also significantly lower when rice inoculated with SUTN9-2:pMG103::acdRS than in the other treatments. After three days of recovery, the amounts of the chlorophyll a in the rice inoculated with SUTN9-2 and SUTN9-2 (ACCDadap) were significantly different compared with uninoculated rice, while the chlorophyll b content was not significantly different except in rice inoculated with SUTN9-2:pMG103::acdRS, and there was a significant decrease in the carotenoid content when rice plants were inoculated with SUTN9-2 (ACCDadap) and SUTN9-2:pMG103::acdRS compared with uninoculated rice (D). The chlorophyll a/b ratios, which are an indicator showing the change of the photosynthetic system for light harvesting, were significantly higher in rice inoculated with bacteria than in uninoculated control rice when water was not withheld. However, under the water deficit stress condition, the inoculation with SUTN9-2 (ACCDadap) resulted in the highest chlorophyll a/b compared with the other treatments. The ratios of chlorophyll a/b after the recovery were significantly different in the rice with bacterial inoculations compared with the uninoculated rice (E).
Effects of water deficit on rice inoculated with improved ACC deaminase activity in the greenhouse
After 20 days without the addition of water, the rice plants displayed wilting symptoms due to the lowering of the soil moisture in the pot. There was also a significant increase in ethylene emissions compared with when the rice were well-watered (). However, the ethylene production from rice plants inoculated with bacteria was significantly lower than uninoculated rice by around 0.26-, 0.78-, and 0.35-folds in SUTN9-2 WT, SUTN9-2 (ACCDadap), and SUTN9-2:pMG103::acdRS, respectively. Moreover, the ethylene production of each treatment showed a similar tendency under well-watered conditions ().
Figure 4. Effects of soil drying (withholding water for 20 days) on ethylene emissions in rice either inoculated with bacteria and uninoculated at 34 days after inoculation (DAI) under greenhouse conditions. Rice under water stress conditions were compared with well-watered rice. Significant differences between treatments are indicated by different letters at P ≤ 0.05 according to Duncan’s multiple range tests. The data are presented as the mean of five replicates and the vertical bars indicate the standard error.
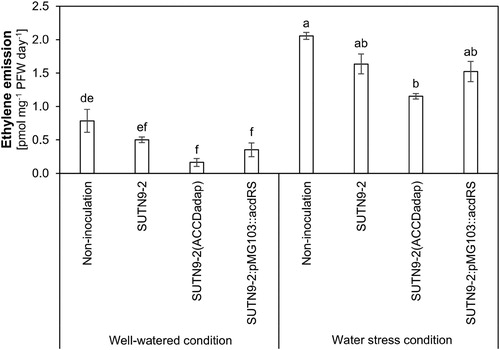
In addition, the efficiency of SUTN9-2 and its derivative strains were observed in the rice morphology while the soil moisture was lowered as well as during the recovery period. The uninoculated plants showed more severe leaf rolling than the rice inoculated with SUTN9-2 (ACCDadap), SUTN9-2:pMG103::acdRS, and SUTN9-2. Furthermore, the inoculated plants also showed greater tendencies toward drought resistance than the un-inoculated plants when we assessed leaf rolling, drought recovery, and drought resistance scores (A and B).
Figure 5. Effects of soil drying (withholding water for 20 days) on (A) rice water stress resistance, rice re-watering, and leaf rolling and (B) rice morphology after re-watering for one day of rice plants either inoculated with bacteria or uninoculated and grown in soil under greenhouse conditions. The numbers 1, 2, 3 and 4 represent the uninoculated, SUTN9-2 inoculated, SUTN9-2 (ACCDadap) inoculated, and SUTN9-2:pMG103::acdRS inoculated treatments, respectively. Significant differences between treatments are indicated with different letters at P ≤ 0.05 according to Duncan’s multiple range tests. The data are presented as the mean of five and ten replicates in the laboratory and greenhouse experiments, respectively, and the vertical bars indicate the standard error.
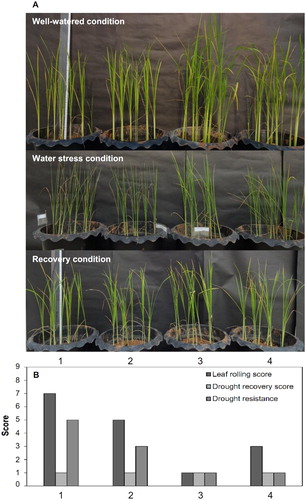
Effect of water deficit stress on rice inoculated with bacteria under field conditions
Since SUTN9-2:pMG103::acdRS is a mutant derivative strain of SUTN9-2 and was generated by genetic engineering technique, it is restricted and cannot be applied in all open field experiments. There was a significant difference in the rice inoculated with SUTN9-2 (ACCDadap) when water was not withheld compared with SUTN9-2 and uninoculated rice, while no significant difference was found during drought conditions. This demonstrated that water deficiency affected plant growth during the seedling stage (A). At the tillering stage, the plant dry weight did not significantly differ between treatments under well-watered conditions, but the rice inoculated with SUTN9-2 (ACCDadap) had significantly higher dry weights in comparison with the uninoculated plants (A). A significant increase in the dry weights were found in rice inoculated with the bacterial strains SUTN9-2 WT and SUTN9-2 (ACCDadap) in both well-watered and water deficit conditions (B). Moreover, the number of seeds, number of infertile grains, 100-grain weight values, and grain yield were not significantly differences between non-inoculation and rice inoculated with bacteria under the well-watered conditions (C-F). In drought conditions, these parameters significant differently in the rice inoculated with bacteria, especially, SUTN9-2 (ACCDadap), when compared with uninoculated rice (C–F), with differences observed in their morphology ().
Figure 6. The average (A) plant dry weight, (B) number of tillers of rice plants at the seedling and tillering stage, (C) number of seeds, (D) number of infertile grains per spike, (E) 100-grain weight, and (F) grain yield of rice plants either inoculated with bacteria and uninoculated and grown in soil under field conditions. Water stressed plants were compared with well-watered plants. Significant differences between treatments are indicated by different letters at P ≤ 0.05 according to Duncan’s multiple range tests. The data are presented as the mean of ten replicates and the vertical bars indicate the standard error. The statistical comparison was performed separately in the well-watered and water stress conditions.
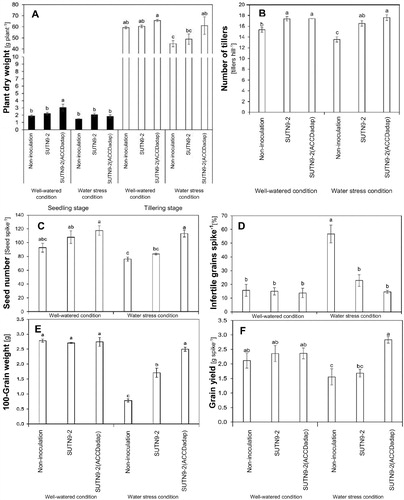
Discussion
In this study, the rice inoculated with endophytic bacteria that produce ACC deaminase (SUTN9-2, SUTN9-2 (ACCDadap), and SUTN9-2:pMG103::acdRS strains) had enhanced plant growth in the laboratory, especially the rice inoculated with SUTN9-2:pMG103::acdRS (A–F), while SUTN9-2 (ACCDadap) exerted beneficial effects on its host at 34 DAI under well-watered conditions in the field experiment and at the tillering stage under the same conditions (A). We suggest that the growth promotion of rice seedlings inoculated with bacteria might be due to the plant growth promoting traits the bacteria supplied to the rice plants. Rice inoculated with SUTN9-2 had promoted growth at seven DAI under NH4NO3 as a nitrogen (N) source and under N-free conditions at 14 DAI; SUTN9-2 also enhanced the biomass of rice seedlings (especially Thai rice cultivars such as O. sativa L. var. indica cv. Pathumthani 1, O. sativa L. cv. KDML 105, and O. sativa Leuang Yai 148) at 30 DAI and 28 DAI when supplemented with 0.1 and 1.0 mM NH4NO3 compared with uninoculated control rice (Piromyou et al. Citation2015a; Piromyou et al. Citation2015b; Greetatorn et al. Citation2019). The same trend was observed in rice treated with 1.0 mM KNO3, NH4NO3 and urea supplementation (Piromyou et al. Citation2017). The production of IAA and ACC deaminase were reported as essential mechanisms for facilitating rice endophytic bacteria and rhizobacteria root colonization as well as plant growth promotion (Bal et al. Citation2013; Etesami et al. Citation2014a, Citation2014b). Both endogenous IAA (produced by plants) and exogenous IAA (produced by bacteria) are absorbed and accumulated in the plant cell, and then rise to high concentrations, resulting in the stimulation of the ACC synthase gene expression. The ACC synthase enzyme converts S-adenosyl methionine into ACC in the ethylene biosynthesis pathway, which is a cause of high ethylene production that acts in cell division or plant growth inhibition (Glick et al. Citation1998). However, the phenomenon is interrupted by bacterial ACC deaminase, which degrades the ethylene’s substrate (ACC) and thus decreases ethylene production. Therefore, the IAA production loop does not affect cell division and/or plant growth, but instead acts as positively by stimulating shoot/root elongation in its host plant along with lowering ACC by ACC deaminase-containing bacteria. In this regard, it could be that the inoculation of rice with SUTN9-2 and its derivative strains may enhance rice growth via this mechanism. Moreover, the levels of enzymatic activity of ACC deaminase might influence plant growth at the seedling stage. We previously showed that the ACC deaminase activity in SUTN9-2:pMG103::acdRS, at 34.72 μmol of α-ketobutyrate h−1 mg−1 protein, was higher than SUTN9-2 by 8.9-fold, while SUTN9-2 and SUTN9-2 (ACCDadap) had 3.88 and 5.58 μmol of α-ketobutyrate h−1 mg−1 protein, respectively (Sarapat et al. Citation2020); this, with the influence of bacterial ACC deaminase, was induced with ACC before planting. Thus, it suggests that the plant growth promotion trait could be enhanced by increasing the efficiency of ACC deaminase activity.
The alleviation of stress in plants is a beneficial effect of the bacteria that produce ACC deaminase activity, and is considered as a mechanism that improves the stress tolerance in the host plants. Significantly, under water-deficit conditions, the rice seedlings inoculated with SUTN9-2:pMG103::acdRS had decreased ethylene emissions compared to the other treatments (A) and also had reduced MDA concentrations (B). This data showed that the bacterial ACC deaminase could influence the antioxidative defense mechanism in rice plants via controlling ethylene synthesis. Under different water deficit conditions, the MDA contents of alfalfa plants were parallel with the levels of the ethylene evolution rate as well as H2O2 levels (Irigoyen et al. Citation1992). This indicated that the ethylene emission levels were directly connected with ROS production, and a large amount of ethylene led to the deterioration of cell membranes, which was determined by the MDA content. Ethylene may act as a plant signaling molecule that not only regulates plant development at different stages, but also is a plant stress response signal. Thus, it could be that SUTN9-2:pMG103::acdRS relieved the negative effects of ROS generation (lipid peroxidation), which was induced by PEG. Moreover, under well-watered conditions, the MDA contents of the rice seedlings inoculated with bacteria were lower than those that were uninoculated (B), which was due to the influence of the ACC deaminase enzyme. However, it seems that the overproduction of ACC deaminase by SUTN9-2:pMG103::acdRS did not exert increased beneficial effects when plants were exposed to water deficit stress in the greenhouse experiment. In this case, we postulate that the water deficit condition induced by PEG, which acted as an osmotic agent to mimic water shortage, leads to what is normally considered as a large secondary peak in ethylene biosynthesis within a few hours without necessarily inducing the first ethylene peak (Glick et al. Citation2007). Therefore, PEG-induced stress could induce large amounts of ACC to be produced, resulting in the increased production of ACC deaminase by SUTN9-2:pMG103::acdRS. This resulted in the highly efficient decrease in ethylene emissions. In contrast, SUTN9-2:pMG103::acdRS did not lower the ethylene emissions of its host plants in the greenhouse experiment, while rice seedlings inoculated with SUTN9-2 (ACCDadap) showed the lowest ethylene emissions (). In this regard, we hypothesized that under greenhouse conditions where the soil used would have induced a dryness stress, the rice plants were exposed to a slow stress condition that resulted in the production of a small amount of ethylene in the first peak to stimulate plant defense mechanisms. The ACC deaminase gene (acdS) in SUTN9-2 (ACCDadap) was mutated at position 419 of the nucleic acid sequence. The point mutation changed the amino acid residue encoding the ACC deaminase enzyme at region 140, which was predicted to be located in its binding site and probably led to a higher affinity for ACC binding than the original SUTN9-2 and higher ACC deaminase activity (Sarapat et al. Citation2020). In contrast, SUTN9-2:pMG103::acdRS, a strain that overproduces ACC deaminase, significantly reduced ethylene emissions due to the increased amount of the enzyme. Even though the ACC deaminase activity of SUTN9-2 (ACCDadap)was lower than that of SUTN9-2:pMG103::acdRS, it was still adequate to promote water deficit tolerance in both field and greenhouse experiments.
After the nutrient solution or water was withheld, wilting of the leaf rice seedlings continuously developed while the leaf RWC (%) decreased (A and C). Wilting is a visual signal of a plant responding to lower turgor pressure (Russell Jones et al. Citation2013; Fang and Xiong Citation2015), and the RWC values of leaves can be used to explain the intensity of the water potential sensing from the roots to the leaves for photosynthesis. Smirnoff (Citation1993) explained that when the reduction of RWC is approximately 70% (medium loss of water), carbon dioxide assimilation is affected and ROS are generated or oxidative stress is induced due to the dark reactions that occur during stomatal closure; when the RWC is lower than 30% (severe water loss), it leads to the drying of plant tissues and plant death, which cannot be recovered. Here, we demonstrated that rice inoculated with bacteria could influence the water retention of plant tissues, with decreased water loss via transpiration during water deficit conditions. Obviously, SUTN9-2 (ACCDadap) and SUTN9-2:pMG103::acdRS enhanced the leaf RWC during both water deficit stress for five days and afterward when re-watering occurred (A and C), while SUTN9-2 (ACCDadap) impacted the lowering of soil moisture conditions (). This water retention led to higher survival rates (B and ). We speculated that the water content in plant tissues, which is regulated by stomatal closure, might be controlled by bacterial ACC deaminase activity via ethylene signaling, which would be produced at different levels depending on ACC deaminase activity. The amount of ethylene produced can affect the signaling pathway ratio between ethylene and abscisic acid, the latter being a regulator for controlling the stomatal aperture with ethylene crosstalk. This ratio can affect the rapid response of guard cells or the size of the stomatal aperture, leading to stomatal closure, closing more slowly, or incomplete closure (Tanaka et al. Citation2005). We demonstrated that both improved strains could efficiently facilitate drought stress tolerance via controlling ethylene to regulate the stomatal aperture, which prevented the drying of plant tissues and plant death.
The plants inoculated with bacteria containing ACC deaminase only improved the plant growth, germination (%), and vigor index, and decreased ethylene production (Karthikeyan et al. Citation2012) but also enhanced the leaf pigment production (chlorophyll a, chlorophyll b and carotenoids). The results indicated that the inoculation of rice seedlings with bacteria could increase the chlorophyll a content. However, both of the improved bacterial strains seemed to affect the chlorophyll a production in rice seedlings under well-watered conditions, while the uninoculated seedlings displayed a remarkable increase in the chlorophyll a content when exposed to water deficit conditions (D). These results demonstrate that bacterial ACC deaminase activity could influence the photosynthetic pigments, especially chlorophyll a, in opposite directions depending on external conditions. The lowering of chlorophyll a might lead to changes in the ratio of photosystem II (PSII) to PSI to decrease the oxidative stress that occurs from the photosynthetic systems (Saeidi and Zabihi-e-Mahmoodabad Citation2009; Pirzad et al. Citation2011; Ashraf and Harris Citation2013). This was confirmed by the higher chlorophyll a/b ratio under water deficit stress (E), which may be a survival strategy. Therefore, when plants face water deficit conditions, the reduced or unchanged pigment contents can improve plant survival and are a trait of drought tolerance. In this sense, bacterial-inoculation seemed to affect the pigment contents of the rice seedlings (D and E), and we hypothesize that the pigment contents in the inoculated rice might be controlled by the ethylene levels via the bacterial ACC deaminase activity. Ethylene can regulate the chlorophyll contents via ethylene response factors (ERFs), which is an abiotic stress-triggered transcription, and ethylene can exert both beneficial and deleterious effects in plants depending on its concentration. Therefore, the perception of ERFs is important to activate and maintain the optimum levels of ethylene, which lead to beneficial signaling (Sharma et al. Citation2019). Overall, these data were evidence that rice endophytic bacteria could facilitate plant growth and mechanisms to avoid cell damage from water deficit stress, especially the SUTN9-2 (ACCDadap) strain. Bacterial inoculations led to improved rice biomass, crop yield, and drought tolerance.
Conclusions
High concentrations of plant ethylene can inhibit plant growth and plant development, leading to reduced rice yields and death. The rice endophytic bacteria Bradyrhizobium SUTN9-2 possesses the ACC deaminase enzyme, which can alleviate the high levels of ethylene that are normally induced by water deficit stress. The efficiency of ACC deaminase in SUTN9-2 and its derivative strains was studied in rice. The results demonstrated that these bacterial strains could promote plant growth plant biomass under well-watered conditions in the laboratory, greenhouse, and field. Meanwhile, the bacteria facilitated the water deficit tolerance of their hosts via the possession of ACC deaminase, which controlled the ethylene emission levels. The reduction of ethylene led to decreased membrane destruction (measured as the MDA content)from ROS and prevented oxidative stress from photosynthesis inhibition by decreasing the chlorophyll contents. Moreover, the endophytic SUTN9-2 and its derivative strains also improved the RWC in leaves, the rate of survival, and plant recovery from the water deficit conditions, and later improved the crop yield. The SUTN9-2 (ACCDadap) strain significantly exerted effects on its host under greenhouse and field conditions, thus demonstrating that SUTN9-2 (ACCDadap) could sustain the rice plants in water stress conditions in the field.
Supplemental Material
Download MS Word (20.3 KB)Acknowledgments
This research was supported by Suranaree University of Technology (SUT) and the Royal Golden.
Disclosure statement
No potential conflict of interest was reported by the author(s).
Additional information
Funding
Notes on contributors
Sukanlaya Sarapat
Sukanlaya Sarapat is working in School of Biotechnology, Institute of Agricultural Technology, Suranaree University of Technology, Nakhon Ratchasima, Thailand
Aphakorn Longtonglang
Aphakorn Longtonglang is working in Suranaree University of Technology Farm (SUT Farm), Suranaree University of Technology, Nakhon Ratchasima, Thailand
Kamolchanok Umnajkitikorn
Kamolchanok Umnajkitikorn is working in School of Crop Production Technology, Institute of Agricultural Technology, Suranaree University of Technology, Nakhon Ratchasima, Thailand
Teerayoot Girdthai
Teerayoot Girdthai is working in School of Crop Production Technology, Institute of Agricultural Technology, Suranaree University of Technology, Nakhon Ratchasima, Thailand
Nantakorn Boonkerd
Nantakorn Boonkerd is working in School of Biotechnology, Institute of Agricultural Technology, Suranaree University of Technology, Nakhon Ratchasima, Thailand
Panlada Tittabutr
Panlada Tittabutr is working in School of Biotechnology, Institute of Agricultural Technology, Suranaree University of Technology, Nakhon Ratchasima, Thailand
Neung Teaumroong
Neung Teaumroong is working in School of Biotechnology, Institute of Agricultural Technology, Suranaree University of Technology, Nakhon Ratchasima, Thailand
References
- Abeles FB, Morgan P, Saltveit M. 1992. Ethylene in plant biology. San Diego: Academic Press.
- Ahmad P. 2016. Water stress and crop plants: a sustainable approach. John Wiley & Sons.
- Ahmad P, Prasad MNV. 2011. Environmental adaptations and stress tolerance of plants in the era of climate change. Springer Science & Business Media.
- Apelbaum A, Yang SF. 1981. Biosynthesis of stress ethylene induced by water deficit. Plant Physiol. 68:594–596. doi: 10.1104/pp.68.3.594
- Ashraf M, Harris PJ. 2013. Photosynthesis under stressful environments: an overview. Photosynthetica. 51:163–190. doi: 10.1007/s11099-013-0021-6
- Bal HB, Das S, Dangar TK, Adhya TK. 2013. ACC deaminase and IAA producing growth promoting bacteria from the rhizosphere soil of tropical rice plants. J Basic Microbiol. 53:972–984. doi: 10.1002/jobm.201200445
- Bleecker AB, Kende H. 2000. Ethylene: a gaseous signal molecule in plants. Annu Rev Cell Dev Biol. 16:1–18. doi: 10.1146/annurev.cellbio.16.1.1
- Blum A. 1996. Crop responses to drought and the interpretation of adaptation. In: Drought tolerance in higher plants: Genetical, physiological and molecular biological analysis. Springer; p. 57–70.
- Cole MA, Elkan GH. 1973. Transmissible resistance to Penicillin G, Neomycin, and Chloramphenicol in Rhizobium japonicum. Antimicrob Agents Chemother. 4:248–253. doi: 10.1128/AAC.4.3.248
- Druege U. 2006. Ethylene and plant responses to abiotic stress. In: Ethylene action in plants. Springer; p. 81–118.
- Du Z, Bramlage WJ. 1992. Modified thiobarbituric acid assay for measuring lipid oxidation in sugar-rich plant tissue extracts. J Agric Food Chem. 40:1566–1570. doi: 10.1021/jf00021a018
- Dubois M, Van den Broeck L, Inzé D. 2018. The pivotal role of ethylene in plant growth. Trends Plant Sci. 23:311–323. doi: 10.1016/j.tplants.2018.01.003
- Duncan DB. 1957. Multiple range tests for correlated and heteroscedastic means. Biometrics. 13:164–176. doi: 10.2307/2527799
- Etesami H, Hosseini HM, Alikhani HA. 2014a. Bacterial biosynthesis of 1-aminocyclopropane-1-caboxylate (ACC) deaminase, a useful trait to elongation and endophytic colonization of the roots of rice under constant flooded conditions. Physiol Mol Biol Plants. 20:425–434. doi: 10.1007/s12298-014-0251-5
- Etesami H, Hosseini HM, Alikhani HA, Mohammadi L. 2014b. Bacterial biosynthesis of 1-aminocyclopropane-1-carboxylate (ACC) deaminase and indole-3-acetic acid (IAA) as endophytic preferential selection traits by rice plant seedlings. J Plant Growth Regul. 33:654–670. doi: 10.1007/s00344-014-9415-3
- Fang Y, Xiong L. 2015. General mechanisms of drought response and their application in drought resistance improvement in plants. Cell Mol Life Sci. 72:673–689. doi: 10.1007/s00018-014-1767-0
- Farooq M, Wahid A, Kobayashi N, Fujita D, Basra S. 2009. Plant drought stress: effects, mechanisms and management. In: Sustainable agriculture. Springer; p. 153–188.
- Fukao T, Xu K, Ronald PC, Bailey-Serres J. 2006. A variable cluster of ethylene response factor–like genes regulates metabolic and developmental acclimation responses to submergence in rice. Plant Cell. 18:2021–2034. doi: 10.1105/tpc.106.043000
- Glick BR, Cheng Z, Czarny J, Duan J. 2007. Promotion of plant growth by ACC deaminase-producing soil bacteria. In: New perspectives and approaches in plant growth-promoting Rhizobacteria research. Springer; p. 329–339.
- Glick BR, Penrose DM, Li J. 1998. A model for the lowering of plant ethylene concentrations by plant growth-promoting bacteria. J Theor Biol. 190:63–68. doi: 10.1006/jtbi.1997.0532
- Greetatorn T, Hashimoto S, Sarapat S, Tittabutr P, Boonkerd N, Uchiumi T, Teaumroong N. 2019. Empowering rice seedling growth by endophytic Bradyrhizobium sp. SUTN 9-2. Lett Appl Microbiol. 68:258–266.
- Grichko VP, Glick BR. 2000. Identification of DNA sequences that regulate the expression of the Enterobacter cloacae UW4 1-aminocyclopropane-1-carboxylic acid deaminase gene. Can J Microbiol. 46:1159–1165.
- Hardoim PR, van Overbeek LS, van Elsas JD. 2008. Properties of bacterial endophytes and their proposed role in plant growth. Trends Microbiol. 16:463–471. doi: 10.1016/j.tim.2008.07.008
- Hodges DM, DeLong JM, Forney CF, Prange RK. 1999. Improving the thiobarbituric acid-reactive-substances assay for estimating lipid peroxidation in plant tissues containing anthocyanin and other interfering compounds. Planta. 207:604–611. doi: 10.1007/s004250050524
- Honma M, Shimomura T. 1978. Metabolism of 1-aminocyclopropane-1-carboxylic acid. Agric Biol Chem. 42:1825–1831.
- Irigoyen J, Emerich D, Sánchez-Diaz M. 1992. Alfalfa leaf senescence induced by drought stress: photosynthesis, hydrogen peroxide metabolism, lipid peroxidation and ethylene evolution. Physiol Plant. 84:67–72. doi: 10.1111/j.1399-3054.1992.tb08766.x
- [IRRI] International Rice Research Institute. 1996. Standard evolution system for rice, 4th ed. Manila, The Philippines: International Rice Research Institute.
- Kanbe M, Yagasaki J, Zehner S, Göttfert M, Aizawa S-I. 2007. Characterization of two sets of subpolar flagella in Bradyrhizobium japonicum. J Bacteriol. 189:1083–1089. doi: 10.1128/JB.01405-06
- Karthikeyan B, Joe MM, Islam MR, Sa T. 2012. ACC deaminase containing diazotrophic endophytic bacteria ameliorate salt stress in Catharanthus roseus through reduced ethylene levels and induction of antioxidative defense systems. Symbiosis. 56:77–86. doi: 10.1007/s13199-012-0162-6
- Li J, Glick BR. 2001. Transcriptional regulation of the Enterobacter cloacae UW4 1-aminocyclopropane-1-carboxylate (ACC) deaminase gene (acdS). Can J Microbiol. 47:359–367. doi: 10.1139/w01-009
- Lichtenthaler H. 1987. Chlorophylls end carotenoids: pigments of photosynthetic bio membranes. Methods Enzimil. 148:350–382.
- Lowry OH, Rosebrough NJ, Farr AL, Randall RJ. 1951. Protein measurement with the Folin phenol reagent. J Biol Chem. 193:265–275.
- Mae T, Ohira K. 1981. The remobilization of nitrogen related to leaf growth and senescence in rice plants (Oryza sativa L. Plant Cell Physiol. 22:1067–1074.
- Michel BE. 1983. Evaluation of the water potentials of solutions of polyethylene glycol 8000 both in the absence and presence of other solutes. Plant Physiol. 72:66–70. doi: 10.1104/pp.72.1.66
- Naveed M, Mitter B, Reichenauer TG, Wieczorek K, Sessitsch A. 2014a. Increased drought stress resilience of maize through endophytic colonization by Burkholderia phytofirmans PsJN and Enterobacter sp. FD17. Environ Exp Bot. 97:30–39. doi: 10.1016/j.envexpbot.2013.09.014
- Naveed M, Mitter B, Yousaf S, Pastar M, Afzal M, Sessitsch A. 2014b. The endophyte Enterobacter sp. FD17: a maize growth enhancer selected based on rigorous testing of plant beneficial traits and colonization characteristics. Biol Fertil Soils. 50:249–262. doi: 10.1007/s00374-013-0854-y
- Pandey V, Shukla A. 2015. Acclimation and tolerance strategies of rice under drought stress. Rice Sci. 22:147–161. doi: 10.1016/j.rsci.2015.04.001
- Piromyou P, Greetatorn T, Teamtisong K, Okubo T, Shinoda R, Nuntakij A, Tittabutr P, Boonkerd N, Minamisawa K, Teaumroong N. 2015a. Preferential association of endophytic bradyrhizobia with different rice cultivars and its implications for rice endophyte evolution. Appl Environ Microbiol. 81:3049–3061. doi: 10.1128/AEM.04253-14
- Piromyou P, Greetatorn T, Teamtisong K, Tittabutr P, Boonkerd N, Teaumroong N, Elkins CA. 2017. Potential of rice stubble as a reservoir of bradyrhizobial inoculum in rice-legume crop rotation. Appl Environ Microbiol. 83:e01488–01417. doi: 10.1128/AEM.01488-17
- Piromyou P., Songwattana P., Greetatorn T., Okubo T., Kakizaki K. C., Prakamhang J., Tittabutr P., Boonkerd N., Teaumroong N., Minamisawa K. 2015b. The type III secretion system (T3SS) is a determinant for rice-endophyte colonization by non-photosynthetic Bradyrhizobium. Microbes Environ. ME15080.
- Pirzad A, Shakiba MR, Zehtab-Salmasi S, Mohammadi SA, Darvishzadeh R, Samadi A. 2011. Effect of water stress on leaf relative water content, chlorophyll, proline and soluble carbohydrates in Matricaria chamomilla L. J Med Plants Res. 5:2483–2488.
- Rice Department. 2016. Rice cultivation situation report, 9th in 2016-17. www.ricethailand.go.th/web/images/pdf/situationrice/5-150760.pdf.
- Russell Jones HO, Thomas H, Waaland S. 2013. Environmental interaction. In: The molecular life of plants. Hoboken: John Wiley & Sons; p. 534–582.
- Saeidi M, Zabihi-e-Mahmoodabad R. 2009. Evaluation of drought stress on relative water content and chlorophyll content of sesame (Sesamum indicum L.) genotypes at early flowering stage. Res J Environ Sci. 3:345–350. doi: 10.3923/rjes.2009.345.350
- Santoyo G, Moreno-Hagelsieb G, del Carmen Orozco-Mosqueda M, Glick BR. 2016. Plant growth-promoting bacterial endophytes. Microbiol Res. 183:92–99. doi: 10.1016/j.micres.2015.11.008
- Sarapat S, Songwattana P, Longtonglang A, Umnajkitikorn K, Girdthai T, Tittabutr P, Boonkerd N, Teaumroong N. 2020. Effects of increased 1-aminocyclopropane-1-carboxylate (ACC) deaminase activity in Bradyrhizobium sp. SUTN9-2 on mung bean symbiosis under water deficit conditions. Microbes and Environments. 35. doi: 10.1264/jsme2.ME20024
- Sharma A, Kumar V, Sidhu GPS, Kumar R, Kohli SK, Yadav P, Kapoor D, Bali AS, Shahzad B, Khanna K. 2019. Abiotic stress management in plants: role of ethylene. Mol Plant Abiotic Stress Biol Biotechnol. 185–208.
- Siddikee MA, Glick BR, Chauhan PS, jong Yim W, Sa T. 2011. Enhancement of growth and salt tolerance of red pepper seedlings (Capsicum annuum L.) by regulating stress ethylene synthesis with halotolerant bacteria containing 1-aminocyclopropane-1-carboxylic acid deaminase activity. Plant Physiol Biochem. 49:427–434. doi: 10.1016/j.plaphy.2011.01.015
- Smirnoff N. 1993. The role of active oxygen in the response of plants to water deficit and desiccation. New Phytol. 125:27–58. doi: 10.1111/j.1469-8137.1993.tb03863.x
- Soedarjo M, Hemscheidt TK, Borthakur D. 1994. Mimosine, a toxin present in leguminous trees (Leucaena spp.), induces a mimosine-degrading enzyme activity in some Rhizobium strains. Appl Environ Microbiol. 60:4268–4272. doi: 10.1128/AEM.60.12.4268-4272.1994
- Sun Y, Cheng Z, Glick BR. 2009. The presence of a 1-aminocyclopropane-1-carboxylate (ACC) deaminase deletion mutation alters the physiology of the endophytic plant growth-promoting bacterium Burkholderia phytofirmans PsJN. FEMS Microbiol Lett. 296:131–136. doi: 10.1111/j.1574-6968.2009.01625.x
- Tanaka Y, Sano T, Tamaoki M, Nakajima N, Kondo N, Hasezawa S. 2005. Ethylene inhibits abscisic acid-induced stomatal closure in Arabidopsis. Plant Physiol. 138:2337–2343. doi: 10.1104/pp.105.063503
- Teulat B, Zoumarou-Wallis N, Rotter B, Salem MB, Bahri H, This D. 2003. QTL for relative water content in field-grown barley and their stability across Mediterranean environments. Theor Appl Genet. 108:181–188. doi: 10.1007/s00122-003-1417-7
- Tittabutr P, Awaya JD, Li QX, Borthakur D. 2008. The cloned 1-aminocyclopropane-1-carboxylate (ACC) deaminase gene from Sinorhizobium sp. strain BL3 in Rhizobium sp. strain TAL1145 promotes nodulation and growth of Leucaena leucocephala. Syst Appl Microbiol. 31:141–150. doi: 10.1016/j.syapm.2008.03.001
- Tittabutr P, Piromyou P, Longtonglang A, Noisa-Ngiam R, Boonkerd N, Teaumroong N. 2013. Alleviation of the effect of environmental stresses using co-inoculation of mungbean by Bradyrhizobium and rhizobacteria containing stress-induced ACC deaminase enzyme. Soil Sci Plant Nutr. 59:559–571. doi: 10.1080/00380768.2013.804391
- Ullah A, Sun H, Yang X, Zhang X. 2017. Drought coping strategies in cotton: increased crop per drop. Plant Biotechnol J. 15:271–284. doi: 10.1111/pbi.12688
- Van de Poel B, Van Der Straeten D. 2014. 1-aminocyclopropane-1-carboxylic acid (ACC) in plants: more than just the precursor of ethylene!. Front Plant Sci. 5:640. doi: 10.3389/fpls.2014.00640
- Vanderstraeten L, Van Der Straeten D. 2017. Accumulation and transport of 1-aminocyclopropane-1-carboxylic acid (ACC) in plants: current status, considerations for future research and agronomic applications. Front Plant Sci. 8:38. doi: 10.3389/fpls.2017.00038
- Wang KL-C, Li H, Ecker JR. 2002. Ethylene biosynthesis and signaling networks. Plant Cell. 14:S131–S151. doi: 10.1105/tpc.001768
- Yu Y-B, Adams DO, Yang SF. 1979. 1-Aminocyclopropanecarboxylate synthase, a key enzyme in ethylene biosynthesis. Arch Biochem Biophys. 198:280–286. doi: 10.1016/0003-9861(79)90420-X