ABSTRACT
Antibiotics as environmental pollutants can affect both the morphology and physiology of higher plants. The first visible symptoms of damage caused by antibiotics are chlorotic changes resulting from the degradation of chlorophyll and the impairment of the chlorophyll biosynthesis pathway, followed by damage to PS II and disturbances in the electron flow between the photosystems. Research on the effects of antibiotics, on plants has recently intensified, however, our understanding of this problem is still far from complete. This paper provides a review of current data on the impacts antibiotics on plant photosynthetic apparatus.
KEYWORDS:
1. Introduction
Modern animal production methods include extensive use of antibiotics. Moreover, the Covid-19 pandemic, although caused by viruses, has further increased the antibiotic use in medicine. Antibiotics are not completely metabolized in the human and animal body, in fact most of the dose taken up is excreted unchanged, thus polluting the environment (Kümmerer Citation2009). The antibiotics most frequently used in animal production and veterinary medicine include tetracyclines, penicillins, and sulphonamides, with their consumption amounting to 33%, 25%, and 11% of all antibiotics used, respectively (EMA Citation2016). In 2020, the countries with the highest antibiotic consumption rates were China (43024 tonnes), Brazil (7533 tonnes), and the United States (6596 tonnes) (Sriram et al. Citation2021).
Although the presence of antibiotic residues in soils and waters is an undisputed fact, there is still no legal requirement to monitor the concentration of antibiotics in the environment. The concentration and persistence of pharmaceuticals in water and soil depends on the physicochemical properties of the drug itself and the properties of the matrix (matrix type, pH) and temperature (Polianciuc et al. Citation2020). For example, the concentration range of tetracycline detected in soil fertilized with natural fertilizers was 10–15 µg × kg−1 soil (Minden et al. Citation2017), while Hamscher et al. (Citation2002) indicate a concentration range of 86–172 mg × kg−1 soil, depending on the depth of sampling. References to antibiotic contents in plants and the aqueous environments (surface water, ground water, drinking water, waste water) are given in the Appendices (Tables 1 and 2). Most studies into the effects of antibiotics on living organisms are focused on animals. The effects of antibiotics on physiological and biochemical processes in plants have been documented but are still poorly understood. Most reports suggest that photosynthesis is one of those areas of plant metabolism that are most rapidly and most severely affected by antibiotic residues. Therefore, this study aims to review the literature concerning the effect of antibiotics on the photosynthetic apparatus of higher plants.
2. The effect of antibiotics on morphology and chlorophyll content in higher plants
Antibiotics in the environment can have both a positive and a negative effect on plants. A positive effect (hormesis) occurs at very low concentrations in the environment, whereas high concentrations of antibiotics result in toxic effects. The first visible symptom of antibiotic toxicity to plants is a change in the color of the photosynthetic organs. Changes in the leaves’ color into light green or yellow under the influence of antibiotics were observed in pea (Margas et al. Citation2016), Lemna gibba (Brain et al. Citation2004), and Lemna minor (Sikorski et al. Citation2014; Baciak et al. Citation2016). The overall chlorophyll content is a significant parameter in the assessment of antibiotics impacts on the photosynthetic apparatus. It was demonstrated that plant exposure to tetracycline at a concentration of 12.84 µM (EC 50) results in 50% inhibition of chlorophyll a synthesis in Lemna minor L., while EC50 for chlorophyll b was observed at a concentration of 0.26 µM (Baciak et al. Citation2016). The inhibition of chlorophyll synthesis under the influence of tetracycline was also examined by Rydzyński et al. (Citation2017). It was demonstrated that after 10 days of growth with the addition of tetracycline at a concentration of 90 mg × kg−1 of soil, chlorophyll a content in yellow lupin (Lupinus luteus) seedlings decreased by 80% in young, developing leaves. In older leaves, chlorophyll degradation was estimated at 68% in relation to the control. Similar results were obtained for spinach Spinacia oleracea (Rydzyński et al. Citation2019) and Iberis sempervirens (Di Marco et al. Citation2014). However, low concentrations of antibiotics may even support the chlorophyll biosynthesis process by affecting nucleic acids and chloroplast proteins, thus reducing the activity of chlorophyllase, and preventing the degradation of chlorophyll (Liu et al. Citation2013). The phenomenon of positive effects of a stress factor on organisms is called hormesis (Agathokleous et al. Citation2018). Hormesis in the plant-antibiotic system was first described by Migliore et al. (Citation2000) in Lythrum salicaria L. treated with fluoroquinols. Hormetic effects of antibiotics were also demonstrated in Raphanus sativus L., Cucumis sativus L., Lactuca sativa L. (Migliore et al. Citation2003). Liu et al. (Citation2011) showed an increase in chlorophyll content in Phragmites australis induced by low concentrations of antibiotics (0.1–1 µg × L−1). Although the mechanism of antibiotic-induced hormesis is not yet understood at the molecular level, production of Reactive Oxygen Species (ROS) seems to be the essential element of this biphasic dose–response pathway. Small amounts of ROS accumulated under stress activate cellular signaling pathways leading to adaptive/beneficial responses (Duarte-Sierra et al. Citation2020).
Additionally, in antibiotic-treated plants, disturbances in the chlorophyll a to chlorophyll b ratio were observed, as well as the chlorotic and necrotic spots manifesting chlorophyll degradation. Under physiological conditions, the ratio of chlorophyll a to b contents in cryptogamic plants is approximately 3:1, with chlorophyll b accounting for 25–35% of the overall chlorophyll content. An decrease in chlorophyll b content in plants treated with antibiotics results in disturbances in electron flow between both photosystems (Liu et al. Citation2013). Changes in the chlorophyll a to chlorophyll b ratio were demonstrated in the brittle willow (Salix fragilis) treated with sulfadimethoxine (Michelini et al. Citation2012). An increase in the chlorophyll a/b ratio was also demonstrated in Spirodela polyrhiza under the influence of amoxicillin (Singh et al. Citation2018). Disturbances in the chlorophyll a/b ratio are a good biomarker that enables an assessment of the condition of the photosynthetic apparatus in plants exposed to stress factors (Li et al. Citation2011b).
The changes in chlorophylls content are accompanied by disturbances in chloroplast structure. The effect of bactericidal compounds on plant photosynthetic apparatus is very probable in view of the fact that chloroplasts are considered descendants of cyanobacteria (Cavalier-Smith Citation2000). In lower plants, systems responsible for chloroplast division have been identified that originate from the bacterial peptidoglycan synthesis pathway. This pathway is the target site for β-lactam antibiotics, which act by interfering with bacterial peptidoglycan polymerization. In Physcomitrella patens, after ampicillin treatment, disturbances in chloroplast division and consequent formation of so-called macrochloroplasts were observed. In Euglena gracilis cells treated with chloramphenicol, a disruption of chloroplast ultrastructure was observed, probably resulting from the inhibition of expression of proteins responsible for lamella structure formation.
Although genes of the peptidoglycan synthesis pathway have not been found in plastids of higher plants, murE and murG genes, involved in the peptidoglycan synthesis pathway in bacteria, have been identified in the Arabidopsis thaliana nuclear genome (Katayama et al. Citation2003).
3. The effect of antibiotics on chlorophyll synthesis
In order for the antibiotic to act on chloroplasts, it has to enter them. Aminoglycoside antibiotics get into the chloroplast via an iron transporter (MAR1, multiple antibiotic resistance 1), which is probably involved in cellular iron homeostasis and allows opportunistic entry of many antibiotics into the chloroplast (Conte et al. Citation2009). After penetrating the chloroplast, the antibiotics may disturb its functioning as early as at the chlorophyll biosynthesis stage. Chlorophyll biosynthesis consists of 16 reactions catalyzed by 16 different enzymes that are encoded by more than 20 genes (Beale Citation2005). The process of chlorophyll synthesis starts with the formation of glutamyl-tRNA and subsequent modifications of the glutamyl-derived moiety into six main precursors: δ-aminolevulinic acid, porphobilinogen, uroporphyrinogen III, protoporphyrinogen IX, Mg-protoporphyrin IX and protochlorophyllide. The end products of this process are both chlorophyll a and chlorophyll b (Brusslan and Peterson Citation2002). To date, the effects of antibiotics on the specific enzymes of chlorophyll biosynthesis have not been determined. A general schematic of chlorophyll biosynthesis is shown in . The sites where antibiotic effects have been studied so far are shown. However, determining the effect of antibiotics on specific reactions is very difficult. It is known that the enzymes involved in chlorophyll biosynthesis are encoded by nuclear genes (Beale Citation2005). One could therefore expect that antibiotics should have no effect on chlorophyll biosynthesis. However, many protein complexes of the chloroplasts are co-coded by both chloroplast and nuclear genes (Larkin et al. Citation2003; von Gromoff et al. Citation2006; Koussevitzky et al. Citation2007). Koussevitzky et al. (Citation2007) showed that the expression of a nuclear gene associated with chlorophyll synthesis can be inhibited by some feedback signaling pathways when a chloroplast gene expression is inhibited. It is likely that chlorophyll biosynthesis is first inhibited at the level of the chloroplast gene expression and then there is also an indirect effect on the nuclear genes expression.
Figure 1. The chlorophyll biosynthesis stages and sites of antibiotics action: 1 –streptomycin, 2 – erythromycin and 3 – ciprofloxacin.
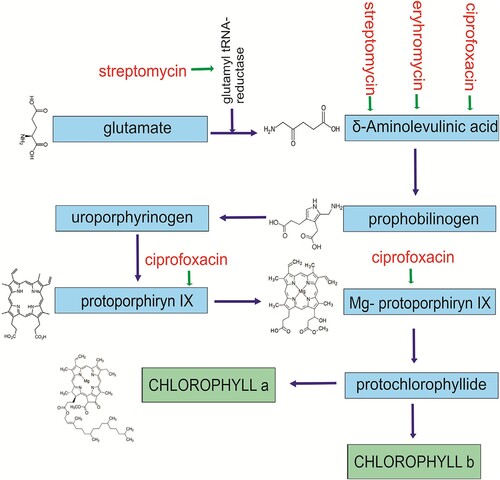
δ-Aminolevulinic acid (ALA) is the first compound of the chlorophyll biosynthesis pathway and thus a chlorophyll precursor (Reinbothe et al. Citation2010). A decrease in ALA content was observed in algae Selenastrum capricornutum treated with erythromycin, ciprofloxacin, and sulfamethoxazole, however, the ciprofloxacin treatment resulted chiefly in reduced contents of protoporphyrin IX and protochlorophyllide (Liu et al. Citation2011). Erythromycin inhibits the production of proteins by reducing the chloroplast gene expression and binding to the small subunit of the ribosome (Liu et al. Citation2011). A decreased ALA content was also demonstrated in soybean (Glycine max) treated with bisphenol at a concentration of 1.5 mg × L−1. On the other hand, increasing the bisphenol concentration to 17.2 and 50 mg × L−1 stimulated the accumulation of δ-aminolevulinic acid (Jiao et al. Citation2015). A similar effect was demonstrated under the influence of thermal stress in the seeds of cucumber and common wheat (Tewari and Tripathy Citation1998).
In contrast, streptomycin (an aminoglycoside targeting the 30S subunit of the ribosome) caused an increase in ALA content as well as a change of chloroplast shape and the disappearance of their ribosomes (Yaronskaya et al. Citation2007). The accumulation of ALA in plant cells results in the generation of free radicals (Noriega et al. Citation2007).
Oxidative stress, and thus the accumulation of free radicals, is a common pathway of plant responses to environmental stress. The accumulation of free radicals under the influence of antibiotics was demonstrated in pea (Margas et al. Citation2019), yellow lupin (Orzoł and Piotrowicz-Cieślak Citation2017), and thale cress (Arabidopsis thaliana) (Gudiño et al. Citation2018; Xu et al. Citation2020), ginger (Lv et al. Citation2020) and many other plants.
4. The effect of antibiotics on the photosynthetic apparatus
The main stages of photosynthesis are light reactions in photosystems I and II (including in the later case the Hill reaction) and CO2 assimilation in the Calvin cycle. Photosystem I (PSI) is a large membrane protein complex and consists of two essential parts: the core complex, also called the reaction center complex (RC), where most of the light capture and charge separation reactions take place, and the light-harvesting complex I (LHCI), which serves as an additional antenna system (Chitnis Citation2001). The plant supercomplex PSI-LHCI consists of 19 known protein subunits and approximately 200 non-covalently bound cofactors (Jensen et al. Citation2007). Photosystem II (PSII) is also a large supramolecular pigment–protein complex embedded in the thylakoid membrane (Barber Citation2003), but it mainly occurs in a dimeric form, and each monomer consists of at least 27–28 subunits organized into a core complex and antenna system (Dekker and Boekema Citation2005). The core contains, among others, proteins D1, D2, CP47, and CP43 that coordinate the molecule of chlorophyll a and form the internal antenna (Shi and Schröder Citation2004). PSII in addition to its bioenergetic and catalytic role in the breakdown of water and oxygen release (Hill reaction) supports the electron transport and is an essential control element in photosynthesis (Geiken et al. Citation1998). Ribulose-1,5-bisphosphate carboxylase-oxygenase (RuBisCO), on the other hand, is a key control in the Calvin cycle (Udenigwe et al. Citation2017). Photosynthesis is one of the main sources of reactive oxygen species (Asada Citation2006), and the presence of antibiotics and their derivatives taken up by plants further enhances this effect (Di Marco et al. Citation2014). PSII and PSI control the mode of photosynthetic electron flow (Iwai et al. Citation2010). The effect of antibiotics on photosynthesis is not fully understood. Studies on the direct effect of antibiotics are shown in . Most of the claims about the effect of antibiotics on photosynthesis are conjectural. What we do know, however, is that antibiotic action leads to H2O2 accumulation in chloroplasts, and this results in damage to the D1 protein in PSII, which is essential for coordinating electron flow between subsequent electron carriers. Hydrogen peroxide in plant cells may result in the inhibition of the D1 protein repair processes, thus leading to irreversible damage to PS II and the inhibition of Hill reaction by 3% and 73% depending on antibiotic concentration (Kummerová et al. Citation2006; Hájková et al. Citation2019). Inhibition of Hill reaction may be due to the inhibition of chloroplast gene expression and, consequently, the synthesis of proteins responsible for electron flow. Wall et al. (Citation2004) demonstrated in Arabidopsis thaliana the presence of DNA gyrase, an enzyme that catalyzes the DNA helix twisting, characteristic of Procaryota. Antibiotics, by affecting the gyrase activity, may cause damage in chloroplasts inhibiting the DNA replication process (Evans-Roberts et al. Citation2016). Treating tobacco (Nicotana tabacum) with antibiotics targeting DNA gyrase (quinolone nalidixic acid and aminocoumarin novobiocin) resulted in the virtually immediate inhibition of plant growth (Heinhorst et al. Citation1985). Inhibition of the inclusion of thymidine in pea chloroplasts was observed (Mills et al. Citation1989). The consequences of nalidixic acid and novobiocin application included also some disturbances that were not directly related to the biosynthesis of plastid DNA. Lam and Chua (Citation1987) demonstrated the inhibition of the transcription process in pea chloroplasts under the influence of novobiocin. Following the treatment of thylakoids with various antibiotics, a disturbance was demonstrated in the chloroplast translation process, caused by a decreased number of Lhcb transcripts (that encode the subunits of the light-harvesting LHC II complex). Moreover, phosphorylation of the proteins contained in the LHC II complex (CP43, D1/D2) was demonstrated in these plants following the application of streptomycin and cyclophosphamide (Mulo et al. Citation2003). The inhibition of the protein translation process in pea chloroplasts under the influence of tetracycline was demonstrated by Kasai et al. (Citation2004). These results show that certain antibiotics may disrupt the transcription and translation processes in plants, thus affecting the proteins contained in the photosynthetic apparatus and leading to multiple damaging effects. Conte et al. (Citation2009) suggest that aminoglycoside antibiotics inhibit chloroplast translation by attacking rRNA of the ribosomal small subunit. Similarly, in prokaryotic organisms aminoglycoside antibiotics also bind to rRNA (16S rRNA) in the small subunit of the ribosome (Recht et al. Citation1999). Kasai et al. (Citation2004) indicates that because chloroplasts possess bacterial transcription and translation systems the inhibitory effect of tetracycline on plant (pea) chloroplast activity in protein synthesis can probably be attributed to a specific interaction of the antibiotic with the 70S ribosome.
Figure 2. Sites of action of antibiotics the deteriorative effect of which in photosynthesis was shown. 1 −□azithromycin; 2 −□streptomycin; 3 −□cyclophosphamide; 4 – fluoroquinolone; 5 – quinolones; 6 −□tetracycline.
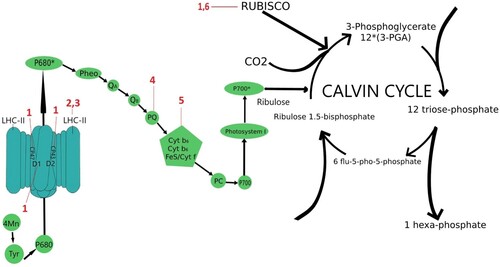
The effect of antibiotics on chloroplast proteins was demonstrated by Margas et al. (Citation2019). In pea seedlings, following the application of tetracycline, an increase was noted in the content of protective proteins involved in the photosynthesis process, inter alia the HCF136 factor involved in the process of stabilizing the PS II. A reduction in the transcription rate of photosynthesis-related nuclear genes, such as Lhcb (chlorophyll-binding protein) and RbcS (ribulose diphosphatocarboxylase small chains) was also demonstrated (Mulo et al. Citation2003). The chlorophyll-binding protein Lhcb builds the main antenna complex and is present as trimer built from homologous binding proteins Lhcb1, Lhcb2 and Lhcb3. LHCII trimers occur in several homo- and heterotrimeric configurations, depending on their location and function. In addition to the trimeric LHCII with PSII (Wei et al. Citation2016), there are also smaller monomeric antenna complexes: Lhcb4 (CP29), Lhcb5 (CP26) and Lhcb6 (CP24) (Caffarri et al. Citation2009; Sacharz et al. Citation2017). However, a detailed elucidation of the antibiotic-induced changes within the antenna complex is still lacking. The emergence of thylakoid protective proteins was also noted. Yoon et al. (Citation2020) demonstrated the degradation of most of the chloroplast structural proteins in Brassica campestris treated with erythromycin (5 mg × L−1). Neither PS II proteins CP47 or CP43 nor PS II protein D1 were identified.
Most studies focus on the examination of damage to photosystem II under the influence of antibiotics. A disturbance in the FV/FM (variable fluorescence to maximum fluorescence) ratio is a good measure of the PS II damage. This coefficient reflects quickly and accurately the degree of damage to PS II. A decrease in this coefficient was noted in the common duckweed under the influence of oxytetracycline. A decrease in this parameter value below 0.85 indicates the PS II photoinhibition caused by the action of antibiotics. A decrease in the FV/FM coefficient value was also demonstrated following the treatment of ginseng with tetracycline (Liu et al. Citation2018). A significant reduction in the FV/FM value was also demonstrated in the leaves of lettuce (Lactuca sativa L. var Ramosa Hort) (Yang et al. Citation2020a) and Lemna minor L. (Kummerová et al. Citation2006). Damage to PS II under the influence of ibuprofen was demonstrated by González-Naranjo et al. (Citation2015). The authors examined the PS II quantum efficiency (ΦPSII) and photochemical quenching in sorghum (Sorghum bicolor). A reduction in these parameters under the influence of ibuprofen was demonstrated. A reduction in the quantum efficiency of photosynthesis was also demonstrated in Microcystis aeruginosa exposed to erythromycin. The disturbance in this parameter value is related to the increase in the proportion of oxidized P700 deprived of donors from the PS II (Deng et al. Citation2014).
Apart from structural damage to PSII and PSI photosystems, antibiotics also cause disturbances in electron flow between the photosystems. Based on measurements of the ETR (electron transport rate) coefficient, a disturbance in electron flow between photosystems was demonstrated in cyanobacterium Microcystis aeruginosa treated with erythromycin. At the same time, it was indicated that PS II was more sensitive to damage. On the other hand, however, increased activity of the cyclic electron transport was noted (Deng et al. Citation2014). A disturbance in the ETR coefficient was also demonstrated in the leaves of Salix fragilis L. treated with sulphonamides (Michelini et al. Citation2012). At the same time, no disturbances in the FV/FM coefficient were noted, which suggests the normal activity of the photosynthetic apparatus and the probable activation of alternative photosynthetic mechanisms. Similar results were obtained for isolated spinach chloroplasts following the application of quinolone antibiotics (although DNA gyrase is generally considered the main target of this group of inhibitors). The authors demonstrated a disturbance in electron flow via PS II to the cytochrome b6/f complex (Reil et al. Citation2001). A disturbance in the electron flow was also demonstrated in Synechocystis sp. following the application of amoxicillin – a member of the β-lactam group of antibiotics, normally targetting the bacterial cell wall biosynthesis system (Pan et al. Citation2008). Measurements of fluorescence demonstrated the inhibition of electron transport on the donor site of PS II, which resulted in the accumulation of P680+, an oxidized form of P680. What is more, the application of amoxycillin at a concentration of 150 mg × L−1 resulted in complete degradation of PS II. Moreover, the effect of amoxycillin on the acceptor site of PS II was demonstrated as well.
Aristilde et al. (Citation2010) demonstrated the effect of fluoroquinolone antibiotics on the thylakoids isolated from spinach chloroplasts by indicating the exact locations where nalidixic acid and ciprofloxacin bond in PS II. It was demonstrated that fluoroquinolone antibiotics had a chemical structure typical of PS II reaction center inhibitors on the plastoquinone side. Nalidixic acid forms two hydrogen bonds on the side of subunit L of plastoquinone QB molecule, thus resulting in disturbances in electron flow. It was also demonstrated that ciprofloxacin indirectly interfered with the photosynthetic apparatus structures by reducing the rate of energy flow from the chlorophyll-harvesting antennas to reaction centers. The increasing ciprofloxacin concentration led to an increase in the Fo parameter which reflects the amount of excited chlorophyll. Under these conditions, energy is not transferred to the PS II reaction center. The authors indicate that the indirect interference of ciprofloxacin with the photosynthetic apparatus is not due to the damages in the chloroplast structures but to the disturbances in the course of photosynthesis. Tests on spinach chloroplasts were also conducted using an antibacterial substance (trachylobane-19-oic acid). This substance interferes with the spinach chloroplast PS II at the level of plastoquinone QA and QB molecules as well as at the LHC II level, thus resulting in the inhibition of photosystem II (Hernández-Terrones et al. Citation2003).
Although responses of the light phase of photosynthesis to contamination with antibiotics are relatively well documented, there are only a few studies concerning the impacts of antibiotics on the Calvin phase of photosynthesis. Significant parameters in the assessment of the efficiency of photosynthesis dark phase include the photosynthesis rate (Pn), transpiration rate (Tr), stomatal conductance (Gs), and the intercellular CO2 concentration. These parameters were examined by Li et al. (Citation2011a) in cereal (Triticum aestivum L.) seedlings treated with oxytetracycline. Significant disturbances of these parameters at each oxytetracycline concentration were demonstrated compared to the control. These disturbances may be due to the decreased degree of carbon assimilation in the plant or disturbances in electron flow. Opriş et al. (Citation2013) also demonstrated a disturbance in the Gs parameter value under the influence of tetracycline in Triticum aestivum L. Photosynthetic parameters were also examined in Pontederia cordata during heavy metal stress. The authors note that the disturbances in photosynthetic parameters (particularly Tr) may result from the accumulation of heavy metals in the leaves (Xin et al. Citation2020). On the other hand, however, Hájková et al. (Citation2019) demonstrated that the disturbances to photosynthetic parameters in Lemna minor L. treated with diclofenac do not result from the direct damage to PS I but from disturbances in electron flow in PS II.
The efficiency of the photosynthetic apparatus, measured by chlorophyll a fluorescence transient, is a very sensitive indicator for the assessment of the degree of plant damage due to abiotic stress. It was shown that the electron transfer in the intersystem chain to the terminal electron acceptors on the PSI acceptor side decreases (Strasser et al. Citation2004). At the same time, it is concluded that chlorophyll a fluorescence measurement is a sensitive and reliable method to detect and quantify changes in PSII and PSI (Chen et al. Citation2017). Siedlewicz et al. (Citation2020) demonstrated that concentrations of 1, 4 and 8 µg/ml oxytetracycline cause a large increase in the absorption of the light harvesting complex in the active center of the reaction and efficient dissipation of energy in the form of heat. Although the authors did not observe an effective utilization of the energy absorbed in the reaction center and transported for use in photosynthesis, there was always an increase in the effective antenna size, which is directly correlated with the chlorophyll concentration.
The enzyme ribulose-1,5-bisphosphate carboxylase-oxygenase (RuBisCo) which catalyzes the binding of CO2 to ribulose-1,5-diphosphate is a key component of the Calvin cycle, the crucial reaction of photosynthesis dark phase. A decrease in the RuBisCo protein content in pea seedlings under the influence of tetracycline was demonstrated by Margas et al. (Citation2016). Decline of RuBisCo enzyme activity under the influence of antibiotics results from the inhibition of the expression of rbcL genes which encode the large RuBisCo subunit (Liu et al. Citation2014). Complete degradation of the large RuBisCo subunit was demonstrated in Brassica campestris under the influence of erythromycin. Interestingly, at the same concentration, a small RuBisCo subunit was not completely degraded, yet its content decreased.
5. The direct effect of antibiotics on chlorophyll
The direct effect of antibiotics on chlorophyll is also very poorly understood. In contrast, the effect of heavy metals (Kondzior and Butarewicz Citation2018; Houri et al. Citation2020; Yang et al. Citation2020b) or acids (Koca et al. Citation2007; Du et al. Citation2017; Hu et al. Citation2021) on chlorophyll degradation has been thoroughly investigated. The chlorophyll degradation pathway called the PAO consists of four main steps. The first step in chlorophyll degradation is the detachment of phytol from the chlorophyll molecule by chlorophyllase (Hörtensteiner Citation2006); the product of the reaction is chlorophyllide. The next step is the removal of the magnesium ion by chelating molecules, leading to the formation of pheophorbide (Hörtensteiner and Kräutler Citation2011). The third step of chlorophyll degradation is the opening of the porphyrin ring of pheophorbide. This reaction results in the formation of fluorescent chlorophyll catabolites (FCCs). The enzyme involved in the third step is pheophorbide oxygenase a (PAO). The final step of the pathway is the conversion of fluorescent products to non-fluorescent chlorophyll catabolites (NCCs) (Hörtensteiner and Kräutler Citation2011). Chlorophyll degradation pathway and possible tetracycline site of action is presented in . Pheophytin, a chlorophyll molecule lacking magnesium but containing phytol, can also be the product of chlorophyll degradation (Schelbert et al. Citation2009).
This type of chlorophyll degradation proceeds particularly easily in an acidic environment. In vitro studies by Rydzyński et al. (Citation2019) demonstrated that the product of chlorophyll degradation by tetracycline is pheophytin () and this is regardless of the ambient pH. The reaction, however, takes place much faster in an acidic environment than in a neutral one. It was confirmed by the analyses of absorption and fluorescence spectra of the ‘pure’ chlorophyll degradation product (pheophytin) – the emergence of new bands at wavelengths of 506 and 536 mm, the hypsochromically shifted fluorescence spectrum, the overlap of the absorption and fluorescence spectra of the chlorophyll degradation product with the spectra of commercially purchased pheophytin, and the disappearance of fluorescence. Moreover, the obtained fluorescence lifetime for the product is identical to that for the commercially purchased pheophytin.
There are two mechanisms of chlorophyll degradation to pheophytin by tetracycline hydrochloride: (1) the loss of Mg2+ ions from the chlorophyll molecule due to the presence of H+ ions in the solution (a result of acidifying the environment by tetracycline hydrochloride), (2) the removal of Mg2+ ions directly from chlorophyll by tetracycline which bonds Mg2+ from chlorophyll. Tetracycline without HCl ions directly removes magnesium from the chlorophyll molecule, yet the reaction proceeds over a longer period. The removed magnesium bonds to the tetracycline molecule in two locations: one Mg2+ ion binds to the tetracycline BCD ring (at low Mg2+ concentrations in the solution) and a second Mg2+ ion bonds to the A ring (at higher Mg2+ concentrations in the solution). Chlorophyll degradation leads to changes in the energy of absorbed and emitted light quanta by the formed Cd-Chl, a decrease in the amount of absorbed light quanta, and a reduction in the chlorophyll fluorescence intensity. The considerable reduction in chlorophyll concentration in plants due to its degradation by tetracycline and the changes in its spectroscopic properties (e.g. a change in energy levels) impair the energy transfer in chlorophyll antennas, and thus contribute to a reduction in photosynthesis efficiency.
6. Conclusion
The presence of antibiotics in the environment is undoubtedly one of the factors causing concerns. The worldwide consumption of antibiotics is steadily increasing, and the COVID-19 pandemic is even enhancing this problem. So far, the effects of antibiotics on plants have rather been documented than thoroughly understood. Antibiotic-caused disturbances arise in plants at various levels – damages to chlorophylls a and b, shifts in the quantitative ratio of these pigments, impairment of chlorophyll biosynthesis pathway, damages to other components of photosystems (PSII in particular), generation of ROS, impairment of electron transfer between both photosystems, inhibition and breakdown of RuBisCo, changes in numbers, sizes and structure of chloroplasts (inhibition of chloroplast divisions, formation of macrochloroplasts, reduced internal membrane system, disappearance of chloroplast ribosomes), in some cases inhibition of chloroplast DNA replication, transcription and translation, down regulation of chloroplast-related nuclear genes. Some of these effects cannot be easily predicted based on the data on antibiotic effects on microorganisms (e.g. gyrase inhibitors in some studies affected not only the plastid DNA replication but also the electron flow to the cytochrome b6/f complex). Plant responses to antibiotic pollution have undoubtedly become a vital area of plant and environmental research.
Conflicts of interest
Conceptualization, AP-C; writing – original draft preparation, MK; writing – review and editing, MK, DJM and AP-C. All authors have read and agreed to the published version of the manuscript.
Supplemental Material
Download MS Word (398.5 KB)Disclosure statement
No potential conflict of interest was reported by the author(s).
Additional information
Funding
Notes on contributors
Magdalena Krupka
Magdalena Krupka is PhD student at Department of Plant Physiology, Genetics and Biotechnology, Faculty Biology and Biotechnology. She focuses on biology and physiology of plants under environmental stress.
Agnieszka I. Piotrowicz-Cieślak
Agnieszka I. Piotrowicz-Cieślak is a Professor at Department of Plant Physiology, Genetics and Biotechnology, Faculty Biology and Biotechnology. She scientific interest is focused on ecotoxicological responses of plants to drugs used in human and veterinary medicine.
Dariusz J. Michalczyk
Dariusz J. Michalczyk is Senior Researcher at Department of Plant Physiology, Genetics and Biotechnology, Faculty Biology and Biotechnology. He focuses on plant molecular biology.
References
- Agathokleous E, Kitao M, Calabrese EJ. 2018. Human and veterinary antibiotics induce hormesis in plants: scientific and regulatory issues and an environmental perspective. Environ Int. 120:489–495. doi:10.1016/j.envint.2018.08.035.
- Aristilde L, Melis A, Sposito G. 2010. Inhibition of photosynthesis by a fluoroquinolone antibiotic. Environ Sci Technol. 44(4):1444–1450. doi:10.1021/es902665n.
- Asada K. 2006. Production and scavenging of reactive oxygen species in chloroplasts and their functions. Plant Physiol. 141(2):391–396. doi:10.1104/pp.106.082040.
- Baciak M, Sikorski Ł, Piotrowicz-Cieślak AI, Adomas B. 2016. Content of biogenic amines in Lemna minor (common duckweed) growing in medium contaminated with tetracycline. Aquat Toxicol. 180:95–102. doi:10.1016/j.aquatox.2016.09.007.
- Barber J. 2003. Photosystem II: the engine of life. Q Rev Biophys. 36(1):71–89. doi:10.1017/s0033583502003839.
- Beale SI. 2005. Green genes gleaned. Trends Plant Sci. 10(7):309–312. doi:10.1016/j.tplants.2005.05.005.
- Brain RA, Johnson DJ, Richards SM, Sanderson H, Sibley PK, Solomon KR. 2004. Effects of 25 pharmaceutical compounds to Lemna gibba using a seven-day static-renewal test. Environ Toxicol Chem. 23(2):371–382. doi:10.1897/02-576.
- Brusslan JA, Peterson MP. 2002. Tetrapyrrole regulation of nuclear gene expression. Photosynth Res. 71(3):185–194. doi:10.1023/A:1015539109209.
- Caffarri S, Kouřil R, Kereïche S, Boekema EJ, Croce R. 2009. Functional architecture of higher plant photosystem II supercomplexes. EMBO J. 28(19):3052–3063. doi:10.1038/emboj.2009.232.
- Cavalier-Smith T. 2000. Membrane heredity and early chloroplast evolution. Trends Plant Sci. 5(4):174–182. doi:10.1016/S1360-1385(00)01598-3.
- Chen Z, Wang Z, Shen Z, Ou D, Xu Z, Yuan S. 2017. Effects of oxytetracycline on growth and chlorophyll fluorescence in rape (Brassica campestris L.). Pol J Environ Stud. 26(3):995–1001. doi:10.15244/pjoes/67575.
- Chitnis R. 2001. Photosystem I: function and physiology. Annu Rev Plant Physiol Plant Mol Biol. 52:593–626. doi:10.1146/annurev.arplant.52.1.593.
- Conte S, Stevenson D, Furner I, Lloyd A. 2009. Multiple antibiotic resistance in Arabidopsis is conferred by mutations in a chloroplast-localized transport protein. Plant Physiol. 151(2):559–573. doi:10.1104/pp.109.143487.
- Dekker JP, Boekema EJ. 2005. Supramolecular organization of thylakoid membrane proteins in Green plants. Biochim Biophys Acta Bioenerg. 1706(1–2):12–39. doi:10.1016/j.bbabio.2004.09.009.
- Deng CN, Zhang DY, Pan XL. 2014. Toxic effects of erythromycin on photosystem I and II in Microcystis aeruginosa. Photosynthetica. 52(4):574–580. doi:10.1007/s11099-014-0063-4.
- Di Marco G, Gismondi A, Canuti L, Scimeca M, Volpe A, Canini A. 2014. Tetracycline accumulates in Iberis sempervirens L. through apoplastic transport inducing oxidative stress and growth inhibition. Plant Biol. 16(4):792–800. doi:10.1111/plb.12102.
- Du E, Dong D, Zeng X, Sun Z, Jiang X, de Vries W. 2017. Direct effect of acid rain on leaf chlorophyll content of terrestrial plants in China. Sci Total Environ. 605–606. doi:10.1016/j.scitotenv.2017.06.044.:764–769.
- Duarte-Sierra A, Tiznado-Hernandez ME, Jha DK, Janmeja N, Arul J. 2020. Abiotic stress hormesis: an approach to maintain quality, extend storability, and enhance phytochemicals on fresh produce during postharvest. Compr Rev Food Sci Food Saf. 19(6):3659–3682. doi:10.1111/1541-4337.12628.
- EMA. 2016. European Medicines Agency Sales of veterinary antimicrobial agents in 30 European countries in 2016- Trends from 2010 to 2016 - Eight ESVAC report. Eur. Med. Agency 2018. https://www.ema.europa.eu/en/documents/report/ sales-veterinary-antimicrobial-agents-30-european-countries-2016-trends-2010-2016-eighth-esvac_en.pdf.
- Evans-Roberts KM, Mitchenall LA, Wall MK, Leroux J, Mylne JS, Maxwell A. 2016. DNA gyrase is the target for the quinolone drug ciprofloxacin in Arabidopsis thaliana. J Biol Chem. 291(7):3136–3144. doi:10.1074/jbc.M115.689554.
- Geiken B, Masojidek J, Rizzuto M, Pompili ML, Giardi MT. 1998. Incorporation of [35S] methionine in higher plants reveals that stimulation of the D1 reaction centre II protein turnover accompanies tolerance to heavy metal stress. Plant Cell Environ. 21(12):1265–1273. doi:10.1046/j.1365-3040.1998.00361.x.
- González-Naranjo V, Boltes K, de Bustamante I, Palacios-Diaz P. 2015. Environmental risk of combined emerging pollutants in terrestrial environments: chlorophyll a fluorescence analysis. Environ Sci Pollut Res. 22(9):6920–6931. doi:10.1007/s11356-014-3899-z.
- Gudiño ME, Blanco-Touriñán N, Arbona V, Gómez-Cadenas A, Blázquez MA, Navarro-García F. 2018. β-Lactam antibiotics modify root architecture and indole glucosinolate metabolism in Arabidopsis thaliana. Plant Cell Physiol. 59(10):2086–2098. doi:10.1093/pcp/pcy128.
- Hájková M, Kummerová M, Zezulka Š, Babula P, Váczi P. 2019. Diclofenac as an environmental threat: impact on the photosynthetic processes of Lemna minor chloroplasts. Chemosphere. 224:892–899. doi:10.1016/j.chemosphere.2019.02.197.
- Hamscher G, Sczesny S, Hoper H, Nau H. 2002. Determination of persistent tetracycline residues in soil fertilized with liquid manure by high-performance liquid chromatography with electronspray ionization tandem mass spectrometry. Anal Chem. 74(7):1509–1518. doi:10.1021/ac015588m.
- Heinhorst S, Cannon G, Weissbach A. 1985. Plastid and nuclear DNA synthesis are not coupled in suspension cells of nicotiana tabacum. Plant Mol Biol. 4(1):3–12. doi:10.1007/BF02498710.
- Hernández-Terrones MG, Aguilar MI, King-Diaz B, Lotina-Hennsen B. 2003. Inhibition of photosystem II in spinach chloroplasts by trachyloban-19-oic acid. Pestic Biochem Physiol. 77(1):12–17. doi:10.1016/S0048-3575(03)00066-X.
- Hörtensteiner S. 2006. Chlorophyll degradation during senescence. Annu Rev Plant Biol. 57(1):55–77. doi:10.1146/annurev.arplant.57.032905.105212.
- Hörtensteiner S, Kräutler B. 2011. Chlorophyll breakdown in higher plants. Biochim Biophys Acta – Bioenerg. 1807(8):977–988. doi:10.1016/j.bbabio.2010.12.007.
- Houri T, Khairallah Y, Zahab AA, Osta B, Romanos D, Haddad G. 2020. Heavy metals accumulation effects on the photosynthetic performance of geophytes in Mediterranean reserve. J King Saud Univ Sci. 32(1):874e880. doi:10.1016/j.jksus.2019.04.005.
- Hu H, Hua W, Shen A, Zhou H, Sheng L, Lou W, Zhang G. 2021, Apr 6. Photosynthetic rate and chlorophyll fluorescence of barley exposed to simulated acid rain. Environ Sci Pollut Res Int. doi:10.1007/s11356-021-13807-8.
- Iwai M, Takizawa K, Tokutsu R, Okamuro A, Takahashi Y, Minagawa J. 2010. Isolation of the elusive supercomplex that drives cyclic electron flow in photosynthesis. Nature. 464(7292):1210–1213. doi:10.1038/nature08885.
- Jensen PE, Bassi R, Boekema EJ, Dekker JP, Jansson S, Leister D, Robinson C, Scheller HV. 2007. Structure, function and regulation of plant photosystem I. Biochim Biophys Acta. 1767:335–352. doi:10.1016/j.bbabio.2007.03.004.
- Jiao L, Wang L, Qiu Z, Wang Q, Zhou Q, Huang X. 2015. Effects of bisphenol A on chlorophyll synthesis in soybean seedlings. Environ Sci Pollut Res. 22(8):5877–5886. doi:10.1007/s11356-014-3764-0.
- Kasai K, Kanno T, Endo Y, Wakasa K, Tozawa Y. 2004. Guanosine tetra- and pentaphosphate synthase activity in chloroplasts of a higher plant: association with 70S ribosomes and inhibition by tetracycline. Nucleic Acids Res. 32(19):5732–5741. doi:10.1093/nar/gkh916.
- Katayama N, Takano H, Sugiyama M, Takio S, Sakai A, Tanaka K, Ono K. 2003. Effects of antibiotics that inhibit the bacterial peptidoglycan synthesis pathway on moss chloroplast division. Plant Cell Physiol. 44(7):776–781. doi:10.1093/pcp/pcg096.
- Koca N, Karadeniz F, Burdurlu HS. 2007. Effect of pH on chlorophyll degradation and colour loss in blanched Green peas. Food Chem. 100(2):609–615. doi:10.1016/j.foodchem.2005.09.079.
- Kondzior P, Butarewicz A. 2018. Effect of heavy metals (Cu and Zn) on the content of photosynthetic pigments in the cells of algae Chlorella vulgaris. J Ecol Eng. 19(3):18–28. doi:10.12911/22998993/85375.
- Koussevitzky S, Nott A, Mockler TC, Hong F, Sachetto-Martins G, Surpin M, Lim J, Mittler R, Chory J. 2007. Signals from chloroplasts converge to regulate nuclear gene expression. Science. 316(5825):715–719. doi:10.1126/science.1140516.
- Kümmerer K. 2009. The presence of pharmaceuticals in the environment due to human use – present knowledge and future challenges. J Environ Manage. 90(8):2354–2366. doi:10.1016/j.jenvman.2009.01.023.
- Kummerová M, Krulová J, Zezulka Š, Tříska J. 2006. Evaluation of fluoranthene phytotoxicity in pea plants by Hill reaction and chlorophyll fluorescence. Chemosphere. 65(3):489–496. doi:10.1016/j.chemosphere.2006.01.052.
- Lam E, Chua NH. 1987. Chloroplast DNA gyrase and in vitro regulation of transcription by template topology and novobiocin. Plant Mol Biol. 8(5):415–424. doi:10.1007/BF00015819.
- Larkin RM, Alonso JM, Ecker JR, Chory J. 2003. GUN4, a regulator of chlorophyll synthesis and intracellular signaling. Science. 299(5608):902–906. doi:10.1126/science.1079978.
- Li ZJ, Xie XY, Zhang SQ, Liang YC. 2011a. Negative effects of oxytetracycline on wheat (Triticum aestivum L.) growth, root activity, photosynthesis, and chlorophyll contents. Agric Sci China. 10(10):1545–1553. doi:10.1016/S1671-2927(11)60150-8.
- Li ZJ, Xie XY, Zhang SQ, Liang YC. 2011b. Wheat growth and photosynthesis as affected by oxytetracycline as a soil contaminant. Pedosphere. 21(2):244–250. doi:10.1016/S1002-0160(11)60124-0.
- Liu B, Liu W, Nie X, Guan C, Yang Y, Wang Z, Liao W. 2011. Growth response and toxic effects of three antibiotics on Selenastrum capricornutum evaluated by photosynthetic rate and chlorophyll biosynthesis. J Environ Sci. 23(9):1558–1563. doi:10.1016/S1001-0742(10)60608-0.
- Liu L, Liu YH, Liu CX, Wang Z, Dong J, Zhu GF, Huang X. 2013. Potential effect and accumulation of veterinary antibiotics in Phragmites australis under hydroponic conditions. Ecol Eng. 53:138–143. doi:10.1016/j.ecoleng.2012.12.033.
- Liu X, Lv Y, Xu K, Xiao X, Xi B, Lu S. 2018. Response of ginger growth to a tetracycline-contaminated environment and residues of antibiotic and antibiotic resistance genes. Chemosphere. 201:137–143. doi:10.1016/j.chemosphere.2018.02.178.
- Liu Y, Zhang J, Gao B, Feng S. 2014. Combined effects of two antibiotic contaminants on Microcystis aeruginosa. J Hazard Mater. 279:148–155. doi:10.1016/j.jhazmat.2014.07.002.
- Lv Y, Xu J, Xu K, Liu X, Guo X, Lu S, Xi B. 2020. Accumulation characteristics and biological response of ginger to sulfamethoxazole and ofloxacin. Environ Pollut. 262:114203. doi:10.1016/j.envpol.2020.114203.
- Margas M, Piotrowicz-Cieślak AI, Michalczyk DJ, Głowacka K. 2019. A strong impact of soil tetracycline on physiology and biochemistry of pea seedlings. Scientifica (Cairo). e3164706. doi:10.1155/2019/3164706.
- Margas M, Piotrowicz-Cieślak AI, Ziółkowska A, Adomas B. 2016. Tetracycline accumulation in pea seedlings and its effects on proteome and enzyme activities. Int J Agric Biol. 18(4):789–796. doi:10.17957/IJAB/15.0166.
- Michelini L, Reichel R, Werner W, Ghisi R, Thiele-Bruhn S. 2012. Sulfadiazine uptake and effects on Salix fragilis L. and Zea mays L. plants. Water Air Soil Pollut. 223(8):5243–5257. doi:10.1007/s11270-012-1275.
- Migliore L, Cozzolino S, Fiori M. 2000. Phytotoxicity to and uptake of flumequine used in intensive aquaculture on the aquatic weed, Lythrum salicaria L. Chemosphere. 40(7):741–750. doi:10.1016/S0045-6535(99)00448-8.
- Migliore L, Cozzolino S, Fiori M. 2003. Phytotoxicity to and uptake of enrofloxacin in crop plants. Chemosphere. 52(7):1233–1244. doi:10.1016/S0045-6535(03)00272-8.
- Mills WR, Reeves M, Fowler DL, Capo SF. 1989. DNA synthesis in chloroplasts: III. The DNA gyrase inhibitors nalidixic acid and novobiocin inhibit both thymidine incorporation into DNA and photosynthetic oxygen evolution by isolated chloroplasts. J Exp Bot. 40(4):425–429. doi:10.1093/jxb/40.4.425.
- Minden V, Deloy A, Volkert AM, Leonhardt SD, Pufal G. 2017. Antibiotics impact plant traits, even at small concentrations. AoB Plants. 9(2):plx010. doi:10.1093/aobpla/plx010.
- Mulo P, Pursiheimo S, Hou CX, Tyystjärvi T, Aro EM. 2003. Multiple effects of antibiotics on chloroplast and nuclear gene expression. Funct Plant Biol. 30(11):1097–1103. doi:10.1071/FP03149.
- Noriega GO, Balestrasse KB, Batlle A, Tomaro ML. 2007. Cadmium induced oxidative stress in soybean plants also by the accumulation of δ-aminolevulinic acid. BioMetals. 20(6):841–851. doi:10.1007/s10534-006-9077-0.
- Opriş O, Copaciu F, Loredana Soran M, Ristoiu D, Niinemets U, Copolovici L. 2013. Influence of nine antibiotics on key secondary metabolites and physiological characteristics in Triticum aestivum: leaf volatiles as a promising new tool to assess toxicity. Ecotoxicol Environ Saf. 87:70–79. doi:10.1016/j.ecoenv.2012.09.019.
- Orzoł A, Piotrowicz-Cieślak AI. 2017. Levofloxacin is phytotoxic and modifies the protein profile of lupin seedlings. Environ Sci Pollut Res. 24(28):22226–22240. doi:10.1007/s11356-017-9845-0.
- Pan X, Deng C, Zhang D, Wang J, Mu G, Chen Y. 2008. Toxic effects of amoxicillin on the photosystem II of Synechocystis sp. characterized by a variety of in vivo chlorophyll fluorescence tests. Aquat Toxicol. 89(4):207–213. doi:10.1016/j.aquatox.2008.06.018.
- Polianciuc SI, Gurzău AC, Kiss B, Ștefan AG, Loghin F. 2020. Antibiotics in the environment: causes and consequences. Med Pharm Rep. 93(3):231–240. doi:10.15386/mpr-1742.
- Recht M, Douthwaite S, Puglisi D. 1999. Basis for prokaryotic specificity of action of aminoglycoside antibiotics. EMBO J. 18(11):3133–3138. doi:10.1093/emboj/18.11.3133.
- Reil E, Höfle G, Draber W, Oettmeier W. 2001. Quinolones and their N-oxides as inhibitors of photosystem II and the cytochrome b6/f-complex. Biochim Biophys Acta – Bioenerg. 1506(2):127–132. doi:10.1016/S0005-2728(01)00189-X.
- Reinbothe C, El Bakkouri M, Buhr F, Muraki N, Nomata J, Kurisu G, Fujita Y, Reinbothe S. 2010. Chlorophyll biosynthesis: spotlight on protochlorophyllide reduction. Trends Plant Sci. 15(11):614–624. doi:10.1016/j.tplants.2010.07.002.
- Rydzyński D, Piotrowicz-Cieślak AI, Grajek H, Michalczyk DJ. 2017. Instability of chlorophyll in yellow lupin seedlings grown in soil contaminated with ciprofloxacin and tetracycline. Chemosphere. 184:62–73. doi:10.1016/j.chemosphere.2017.05.147.
- Rydzyński D, Piotrowicz-Cieślak AI, Grajek H, Wasilewski J. 2019. Investigation of chlorophyll degradation by tetracycline. Chemosphere. 229:409–417. doi:10.1016/j.chemosphere.2019.05.035.
- Sacharz J, Giovagnetti V, Ungerer P, Mastroianni G, Ruban AV. 2017. The xanthophyll cycle affects reversible interactions between PsbS and light-harvesting complex II to control non-photochemical quenching. Nat Plants. 3:16225. doi:10.1038/nplants.2016.225.
- Schelbert S, Aubry S, Burla B, Agne B, Kessler F, Krupinska K, Hörtensteiner S. 2009. Pheophytin pheophorbide hydrolase (pheophytinase) is involved in chlorophyll breakdown during leaf senescence in Arabidopssis. Plant Cell. 21(3):767–785. doi:10.1105/tpc.108.064089.
- Shi LX, Schröder WP. 2004. The low molecular mass subunits of the photosynthetic supracomplex, photosystem II. Biochim Biophys Acta. 1608(2-3):75–96. doi:10.1016/j.bbabio.2003.12.004.
- Siedlewicz G, Żak A, Sharma L, Kosakowska A, Pazdro K. 2020. Effects of oxytetracycline on growth and chlorophyll a fluorescence in Green algae (Chlorella vulgaris), diatom (Phaeodactylum tricornutum) and cyanobacteria (Microcystis aeruginosa and Nodularia spumigena). Oceanologia. 62(2):214–225. doi:10.1016/j.oceano.2019.12.002.
- Sikorski Ł, Adomas B, Dobiesz M, Baciak M, Piotrowicz-Cieślak AI. 2014. Morphological and biochemical responses of Lemna minor L. (common duckweed) to ciprofloxacin. Fresen Environ Bull. 23:363–371.
- Singh V, Pandey B, Suthar S. 2018. Phytotoxicity of amoxicillin to the duckweed Spirodela polyrhiza: growth, oxidative stress, biochemical traits and antibiotic degradation. Chemosphere. 201:492–502. doi:10.1016/j.chemosphere.2018.03.010.
- Sriram A., Kalanxhi E., Kapoor G., Craig J., Balasubramanian R., Brar S., Criscuolo N., HamiltonA K. E., Tseng K., Van Boeckel T., Laxminarayan R. 2021. State of the world's antibiotics 2021: a global analysis of antimicrobial resistance and its drivers. Washington D.C: Center for Disease Dynamics, Economics & Policy.
- Strasser M., Tsimilli M., Srivastava A. 2004. Analysis of the chlorophyll a fluorescence transient. In Papadogeorgiou GC, H Govindjee, editors. Chlorophyll a fluorescence: a signature of photosynthesis. Dordrecht: Springer; pp. 321–362.
- Tewari AK, Tripathy BC. 1998. Temperature-stress-induced impairment of chlorophyll biosynthetic reactions in cucumber and wheat. Plant Physiol. 117(3):851–858. doi:10.1104/pp.117.3.851.
- Udenigwe CC, Okolie CL, Qian HF, Ohanenye IC, Agyei D, Aluko RE. 2017. Ribulose-1,5-bisphosphate carboxylase as a sustainable and promising plant source of bioactive peptides for food applications. Trends Food Sci Technol. 69:74–82. doi:10.1016/j.tifs.2017.09.001.
- von Gromoff ED, Schroda M, Oster U, Beck CF. 2006. Identification of a plastid response element that acts as an enhancer within the Chlamydomonas HSP70A promoter. Nucleic Acids Res. 34(17):4767–4779. doi:10.1093/nar/gkl602.
- Wall MK, Mitchena LA, Maxwell A. 2004. Arabidopsis thaliana DNA gyrase is targeted to chloroplasts and mitochondria. Proc Natl Acad Sci USA. 101(20):7821–7826. doi:10.1073/pnas.0400836101.
- Wei X, Su X, Cao P, Liu X, Chang W, Li M, Zhang X, Liu Z. 2016. Structure of spinach photosystem II-LHCII supercomplex at 3.2 Å resolution. Nature. 534(7605):69–74. doi:10.1038/nature18020.
- Xin J, Ma S, Li Y, Zhao C, Tian R. 2020. Pontederia cordata, an ornamental aquatic macrophyte with great potential in phytoremediation of heavy-metal-contaminated wetlands. Ecotoxicol Environ Saf. 203:111024. doi:10.1016/j.ecoenv.2020.111024.
- Xu D, Pan H, Yao J, Feng Y, Wu P, Shao K. 2020. Stress responses and biological residues of sulfanilamide antibiotics in Arabidopsis thaliana. Ecotoxicol Environ Saf. 199:110727. doi:10.1016/j.ecoenv.2020.110727.
- Yang T, Jiang Z, Lin D, Guo L, Wu T, Li Z, He Z, Zhou X. 2020a. Effects of tetracycline and oxytetracycline on growth and chlorophyll fluorescence in lettuce seedlings. In Proceedings of the IOP Conference Series: Earth Environ. Sci. 474:022013.
- Yang Y, Zhang L, Huang X, Zhou Y, Quan Q, Li Y, Zhu X. 2020b. Response of photosynthesis to different concentrations of heavy metals in Davidia involucrata. PLoS ONE. 15(3):e0228563. doi:10.1371/journal.pone.0228563.
- Yaronskaya EB, Gritskevich ER, Trukhanovet NL, Averina NG. 2007. Effect of kinetin on early stages of chlorophyll biosynthesis in streptomycin-treated barley seedlings. Russ J Plant Physiol. 54(3):388–395. doi:10.1134/S1021443707030144.
- Yoon YE, Cho HM, Bae D, Lee SJ, Choe H, Kim MC, Cheong MS, Lee YB. 2020. Erythromycin treatment of Brassica campestris seedlings impacts the photosynthetic and protein synthesis pathways. Life. 10(12):311. doi:10.3390/life1012031.