ABSTRACT
Whether nutrient availability interacts with induced resistance to alter plant chemistry and, consequently, the preference and performance of herbivores on plants remains unclear. We hypothesized that changing fertilizer inputs modulates responses induced by exogenous application of the defensive phytohormone methyl jasmonate (MeJA) against Tuta absoluta, a devastating pest of tomatoes. We found that tomato plants grown at or 2-fold above optimal fertilizer rates had higher nutrient content and were more preferred by T. absoluta females for oviposition and were better hosts for their offspring. MeJA increased phenolic content and volatile emissions in plants, which correlated with lower T. absoluta oviposition preference and offspring performance. However, we found no significant interactions when fertilizer and MeJA were applied simultaneously, such that induced responses against T. absoluta in tomatoes were similar regardless of fertilizer rate. These results provide novel insights into the bottom-up effects of fertilization and induced resistance on plant-insect herbivore interactions.
Introduction
Most plants must frequently deal with a variety of biotic stresses, such as the attack by insect herbivores, and in response, they can protect themselves through physical and chemical resistance traits to avoid or reduce herbivore damage (Karban and Myers Citation1989; War et al. Citation2012). These resistance traits can be constitutive, i.e. always present, or inducible, i.e. only produced or mobilized in response to damage by herbivores (Karban and Myers Citation1989). Induced plant resistance occurs upon recognition of an herbivore attack and may often increase the levels of physical and chemical defensive traits that, in turn, reduce the preference (i.e. antixenosis) and performance (i.e. antibiosis) of herbivores on plants (Bezemer and Van Dam Citation2005; Eyles et al. Citation2010; Alba et al. Citation2011; Poelman Citation2015). The expression of many of these plant resistance-related traits is regulated through hormones (Stella de Freitas et al. Citation2019). Specifically, the activation of inducible resistance in plants against chewing insects and necrotrophic pathogens is mainly regulated by the jasmonic acid (JA) defensive pathway (Thaler et al. Citation2001; Howe and Jander Citation2008; Erb et al. Citation2012; Acevedo et al. Citation2015; Tortorici et al. Citation2022). For instance, exogenous application of jasmonates, i.e. JA or its volatile derivative methyl jasmonate (MeJA), often induces the production of secondary metabolites in plants that deter herbivore feeding, reduce their survival, or inhibit their digestion, i.e. direct resistance traits, in a manner like those induced by chewing herbivores (Chen et al. Citation2005; Wei et al. Citation2021). In addition, exogenous jasmonate application induces volatile emissions in plants that can modify the herbivore’s behavior (Rodriguez-Saona et al. Citation2001; Cao et al. Citation2014) and attract their natural enemies, i.e. indirect resistance traits (Kessler and Baldwin Citation2001; Yu et al. Citation2018). Thus, these jasmonates are often used to mimic herbivory and induce plant resistance (Thaler et al. Citation2001; Tortorici et al. Citation2022).
In addition to biotic stresses, abiotic stresses can also affect plant chemistry, leading to changes in trophic cascades through bottom-up forces (Hunter and Price Citation1992; Power Citation1992; Amtmann et al. Citation2008; Chen et al. Citation2010; Han, Becker, et al. Citation2019). One important factor that could trigger strong bottom-up effects in plant-insect interactions is plant nutrient availability (Poelman Citation2015; Han et al. Citation2022). However, despite its importance, plant nutrient availability has received little attention within the practical context of integrated pest management (IPM) (Han et al. Citation2022). In agroecosystems, fertilizer application often alters plant quality via changes in the plant’s nutritional status, which in turn may affect the survival, growth, and behavior of insect herbivores (Joern et al. Citation2012; Han et al. Citation2014; Islam et al. Citation2017; Li et al. Citation2021). For instance, nitrogen is an essential nutrient in regulating leaf photosynthesis (Hou et al. Citation2019) that has been widely studied in terms of its impact on insect herbivores (Sétamou et al. Citation1993; Han et al. Citation2022). Low nitrogen levels in plant tissues are known to reduce herbivore survival, performance, and/or fertility (Awmack and Leather Citation2002; Schoonhoven et al. Citation2005; Han et al. Citation2014). In contrast, high nitrogen levels in plants often result in increased performance of herbivores, which may subsequently alter their population dynamics, leading to rapid herbivore outbreaks (Throop and Lerdau Citation2004; Schoonhoven et al. Citation2005).
Despite this knowledge, it is still unclear how plants cope with simultaneous biotic and abiotic stressors, such as protection against herbivores under limited nutrient availability. Because the activation of defense pathways can demand considerable resources, induced resistance in plants is assumed to be energy-intensive (Gershenzon Citation1994; Kessler and Baldwin Citation2002; Hahn and Maron Citation2016). In this way, plants continuously deal with the dilemma of how to allocate their resources to growth or defense, particularly when these resources are limited (Herms and Mattson Citation1992). According to the resource availability hypothesis, fast-growing plants that are adapted to resource-rich environments should have high foliar nutrient concentrations, high tissue turnover rates, and low investment in antiherbivore defenses and, consequently, should experience high levels of herbivore damage (Coley Citation1987). In contrast, plants in resource-poor environments should show slow growth rates and enhanced resistance to herbivores. Thus, according to this hypothesis, we expect trade-offs between nutrient availability and plant resistance to herbivores.
In tomato (Solanum lycopersicum L.) plants, exogenous MeJA application increased defensive proteins, including trypsin proteinase inhibitors and polyphenol oxidases, that reduced the performance of insect herbivores such as the corn earworm, Helicoverpa zea (Boddie) (Paudel et al. Citation2014) and the cotton bollworm, Helicoverpa armigera Hübner (Tan et al. Citation2012). Additionally, low nitrogen inputs applied to tomato plants increase concentrations of soluble phenolics, glycoalkaloids, polyphenol oxidases, and volatile compounds (Stout et al. Citation1998; Larbat et al. Citation2016; Islam et al. Citation2017; Becker et al. Citation2021) and impair the performance (Han et al. Citation2014; Larbat et al. Citation2016), longevity (Blazhevski et al. Citation2018), and fertility (Blazhevski et al. Citation2018) of the South American tomato pinworm Tuta absoluta (Meyrick), as well as the attraction of the whitefly Bemisia tabaci (Gennadius) (Islam et al. Citation2017). However, the interactive effects of jasmonate-induced resistance and nutrient availability on the preference-performance relationship of T. absoluta in tomato plants have not been studied.
In this study, we hypothesized that nutrient availability interacts with jasmonate-induced resistance to affect plant chemical defenses and the preference and performance of T. absoluta on tomato plants. Specifically, we asked the two following questions: (1) What are the bottom-up effects of fertilization and MeJA on tomato plant growth and resistance traits (direct and indirect) and on the preference and performance of T. absoluta? (2) Do fertilization and MeJA interact to affect tomato plant growth and resistance traits and the preference and performance of T. absoluta? Our study aims to better understand how nutrient availability may modulate plant resistance traits against a specialist herbivore of tomato and other solanaceous plants, which can have practical implications within an IPM framework.
Materials and methods
Plants and insects
We tested our hypothesis in a system that consisted of tomato plants and the herbivore T. absoluta (Lepidoptera: Gelechiidae), a serious insect pest of tomatoes in its native range (South America) that has recently invaded new areas around the world (Desneux et al. Citation2010; Mansour et al. Citation2018; Han, Bayram, et al. Citation2019; Desneux et al. Citation2022).
Tomato plants, S. lycopersicum cv. Santa Clara, were grown from seeds in a greenhouse under natural light and temperature conditions (24 ± 5.5°C) in Basaplant soil (Base Agro, São Paulo, Brazil) in plastic cells; the cultivar Santa Clara is highly susceptible to T. absoluta (Proffit et al. Citation2011). Seedlings at 6–7 cm high (15–20 days after sowing) were transferred to 1.9-L round plastic pots (17 × 15 × 12 cm) containing Basaplant and coconut fiber (1:1). Plants were maintained in the greenhouse, irrigated as needed, and used in experiments (see ‘MeJA treatment’ below) when they had six fully expanded leaves (40–45 days after sowing).
Tuta absoluta was obtained from a laboratory colony initiated in 2018 from larvae and pupae collected from commercial tomato farms located in Paulínia (São Paulo, Brazil). The colony was maintained on tomato plants, cv. Santa Cruz, in the Department of Entomology and Acarology at ESALQ/USP (Piracicaba, São Paulo, Brazil). Larvae and adults were kept in separate cages (60 × 60 × 30 cm) covered with a fine mesh under controlled conditions (25 ± 2°C, 70 ± 10% relative humidity [RH], 12:12 light:dark [L:D]). An aqueous honey solution (10%) was provided on a cotton wool as a food source for adults inside the cage. Adults used in oviposition studies were 2‒3 days old, and eggs used in performance studies were 60‒72 h old.
Fertilization regimes
After being transplanted to the round plastic pots, 15–20 days after sowing as described above, plants were grown in soil treated with one of the following four different rates of Basacote® Plus 6M nitrogen, phosphorus, and potassium (NPK) 16:8:12(+2) (Compo, Münster, Germany), a slow-release fertilizer: (1) low = 0.05 g/L (0.025×), (2) medium = 1 g/L (0.5×), (3) optimal = 2 g/L (1×), and (4) high = 4 g/L (2×). These rates were based on recommendations of the manufacturer (https://www.compo-expert.com/sites/default/files/2020-01/Folder_Basacote_EN_2018.pdf). Because tomato plants do not grow well without fertilizer, the lowest fertilizer rate used was 0.025× the optimal rate; in addition, we tested a rate below and a rate above the optimal rate following methods in de Lange et al. (Citation2019). Fertilizer applications were made once. Different sets of plants were used to study the effects of fertilization on the preference and performance of T. absoluta and on plant chemistry (see details below).
MeJA treatment
To determine a MeJA concentration that affects T. absoluta performance on tomatoes, we conducted a preliminary study to test the effects of different MeJA concentrations on T. absoluta. Plants (40–45 days old) were individually sprayed 25 days after the fertilizer treatment with suspensions of 15 mL MeJA (Sigma-Aldrich, St. Louis, MO, USA) at 0 (control), 1, 2, or 3 mM dissolved in 0.1% Tween-20. Control treatments were sprayed with 0.1% Tween-20 only. Treatments were applied to plants using an atomizer until run-off. To avoid contamination, MeJA and control sprays were performed in different greenhouses maintained under the same environmental conditions mentioned above, and plants remained in separate greenhouses until the start of the experiments. Three days after the MeJA and control treatments, four T. absoluta eggs were individually placed on different leaves within plants; 16 plants (replicates) were used for each treatment. After larval development was completed, pupal weights were recorded.
Based on the results from these preliminary studies (see Supplemental Figure 1), we conducted all the experiments on the effects of MeJA application on the preference and performance of T. absoluta and on plant chemistry (see details below) using 15 mL of a 2 mM MeJA solution per plant. This (2 mM) was the lowest MeJA concentration that negatively affected T. absoluta pupal weight. All these experiments were conducted three days after the MeJA treatment, as in Tan et al. (Citation2012), and different sets of plants were used for each experiment.
Oviposition preference
Three choice experiments were carried out to determine T. absoluta oviposition preference in response to fertilizer rates and induction by MeJA on tomato plants. All experiments were conducted in an empty greenhouse under natural light and temperature conditions (24 ± 5.5°C). In all experiments, T. absoluta adults were removed from the plants after 24 h and the number of eggs laid on each plant was counted. Each choice experiment was replicated 8 times.
Two-choice tests. We conducted two-choice tests to compare the oviposition preference of T. absoluta between MeJA-induced plants and non-induced (control) plants, under the same fertilization rate. Plants from each treatment were placed 0.8 m apart on opposite sides of a cage (100 × 70 × 50 cm), covered with fine netting, in a greenhouse. Cages were at least 2 m from each other and 6 T. absoluta adults (3 males and 3 females) were released inside each cage.
Four-choice tests. We conducted four-choice tests to compare the oviposition preference of T. absoluta among plants exposed to the four different fertilization rates (low, medium, optimal, and high) under the same induction status (i.e. plants induced by MeJA or non-induced plants). The four plants were arranged randomly in a square pattern at 1 m from each other inside a greenhouse (4 × 4 × 4 m). Twelve T. absoluta adults (6 males and 6 females) were then released inside the greenhouse. Each replicate was run on a separate day.
Multiple-choice test. We conducted multiple-choice tests to compare the oviposition preference of T. absoluta among all eight treatment combinations (plants under four different fertilization regimes that were induced and not induced by MeJA). Plants were arranged in a (3.5 m diameter) circle inside a greenhouse (4 × 4 × 4 m), such that a plant from each treatment combination was placed randomly at about 1 m from each other. Following, 24 T. absoluta adults (12 males and 12 females) were released inside the greenhouse. Each replicate was run on a separate day.
Offspring performance
To assess T. absoluta offspring performance on plants, four T. absoluta eggs were placed with a fine bush on plants inside of cages (45 × 30 × 30 cm) covered in fine netting and kept in a greenhouse under natural light and temperature conditions (24 ± 5.5°C). The eggs were individually placed in different leaves within the plants and were inspected daily until they hatched. If an egg failed to hatch, a newly emerged larva was placed on the same leaf. Larval survival, developmental time (days) from egg to pupa, and pupal weights were recorded. Thirteen plants (replicates) were used for each of the 8 treatment combinations, i.e. plants under different fertilization regimes treated or not treated with MeJA.
Plant growth
Aboveground plant dry weight and plant height were used as proxy for plant growth. Plants (n = 8) for each of the 8 treatment combinations were cut just above the soil surface from the pots, their lengths were measured, and were then dried in paper bags in a drying oven (Quimis Q317M32, São Paulo, Brazil) at 60°C for 72 h. After drying, samples were weighed to determine differences on dry plant biomass among treatments.
Soil nitrogen levels
To quantify the amounts of nitrogen in the soil, soil samples (∼100 g, n = 5) were taken from pots from each of the 8 treatment combinations. Soil samples were dried at 45°C for 72 h and sieved (∼2-mm mesh), and total nitrogen was quantified by the Kjeldahl method (Nelson and Sommers Citation1980).
Nutrient levels in leaves
Leaf samples (140 g, n = 5) were taken for each of the eight treatment combinations and dried in a stove at 60°C for 72 h. Dried samples were ground and then subjected to sulfuric digestion to determine nitrogen concentrations by the Kjeldahl method (Nelson and Sommers Citation1980) and subjected to nitric-perchloric digestion to determine the concentration of phosphorus, potassium, calcium, magnesium, and sulfur (Zarcinas et al. Citation1987).
Total phenolics
We measured levels of total phenolics as proxy of a direct resistance trait. Total phenolic content was determined by a modification of the Folin-Ciocalteu method (Ainsworth and Gillespie Citation2007). In short, leaf samples (0.3 g) were frozen in liquid nitrogen and placed into 15-mL glass tubes with 4 mL methanol (50%). The extract was incubated in a water bath (Marconi MA156, São Paulo, Brazil) for 1.5 h at 80°C and centrifuged (Sorvall instruments RC5C centrifuge, Dupont, DE, USA) at 20,000 g for 15 min at room temperature, and then the supernatant was collected in a new tube (Kofalvi and Nassuth Citation1995). A total of 150 µL of this extract was mixed with 150 µL Folin reagent (Sigma Aldrich, Merck, Germany), previously diluted with water (1:1), and allowed to stand for 5 min. Then, 3 mL of Na2CO3 (2%) was added. After 5 min, the mixture was measured at 750 nm on a spectrophotometer (Hitachi U-1900, Tokyo, Japan). A standard curve was prepared with chlorogenic acid at concentrations of 0, 10, 20, 30, 40, 50, 100, 150, and 250 µg/mL (Bray and Thorpe Citation1954). Five samples (replicates) of each of the 8 treatment combinations were analyzed.
Collection and analysis of volatile emissions
We measured volatile emissions as a proxy of an indirect resistance trait. Headspace volatile samples were taken from tomato plants exposed to one of the four fertilizer regimes and one of the two induction treatments for 4 h during daytime under laboratory conditions (25 ± 1°C, 70 ± 10% RH). Pots containing the plants were wrapped with aluminum foil to avoid trapping volatiles from plastic and soil. After that, plant shoots were individually enclosed in a 15-L glass chamber and connected to a volatile collection system (Analytical Research Systems Inc., Gainesville, FL, USA). Clean humidified air was pushed at 0.8 L/min into the glass chamber connected to a column containing an adsorbent polymer (30 mg; Hayesep-Q, Alltech Associates, Deerfield, IL, USA) that trapped the volatiles, which was connected to a vacuum pump pulling air at the same flow rate. Following, the polymer column was eluted with 150 µL of distilled hexane, and the samples were stored in glass vials at −30°C until analysis. Dry weight of the plant shoots was determined. Five replicates for each of the 8 treatment combinations were sampled.
Volatile quantification was performed by gas chromatography coupled to a flame ionization detector (GC-FID; GC2010 Shimadzu, Kyoto, Japan) operated at 280°C. Nonyl acetate (Sigma Aldrich, St. Louis, MO, USA) was added (10 µL of a 10-ng/µL solution) in each sample as an internal standard. A 2-µL aliquot of each sample was injected in the column with helium as the carrier gas (24 cm/s). The relative amount of the compounds in each sample was determined based on a comparison of their peak area with that of the internal standard and standardized per unit of fresh shoot biomass (g) for each of the replicates. For volatile identification, the most representative sample of each treatment was further analyzed by gas chromatography coupled to a mass spectrometer (GC-MS; Agilent 6890 Series GC system G1530A), following the same set-up of the GC-FID analysis. Volatile compounds were tentatively identified by comparing the mass spectra obtained in the GC-MS run with the NIST11 library database and by comparison with published Kovats retention indices. Authentic standards were used to confirm the identities of α-pinene, β-myrcene, α-phellandrene, limonene, γ-terpinene, and β-caryophyllene (Sigma-Aldrich, Merck KGA, St. Louis, Missouri, USA).
Data analyses
The experimental design was 4 × 2 factorial, with four fertilizer regimes and two induction treatments for a total of eight treatment combinations. Prior to the analyses, all data were checked for normality using the Shapiro-Wilk test and homogeneity of variances using the Levene’s test. All data analyses were performed in Minitab version 17 (Minitab Citation2013) and R software version 2022.02.3 (R Core Team Citation2022) using the packages ‘hnp,’ ‘emeans,’ and ‘multcomp.’
Two-choice tests to determine T. absoluta oviposition preference were analyzed using Student’s t tests. Four-choice and multiple-choice tests were analyzed using two-way analysis of variance (ANOVA), testing for the effects of fertilizer rates, MeJA-induced resistance, and the interaction between them (fixed factors); replicate was included in the model as a random factor.
Larval survival, egg-larva developmental times, pupal weights, plant growth, nitrogen in the soil, and total phenolics in leaves were analyzed using two-way ANOVA with fertilizer rates, MeJA-induced resistance, and the interaction between them as independent factors. Prior to the analysis, data on offspring performance were averaged for each plant (replicate), and data on percent larval survival were arcsine-square-root transformed. Replicate was included in the model as a random factor.
Since the components of the nutrient and volatile profiles are not independent, we used two-way multivariate analysis of variance (MANOVA) to analyze the effects of fertilizer rates, MeJA-induced resistance, and their interaction on the levels of leaf nutrients and volatile emissions. A significant MANOVA effect was then followed by generalized linear model (GLM) with a gamma distribution (log link) to assess which individual component within the nutrient and volatile profiles was affected by treatment.
If needed, data were transformed prior to ANOVA and MANOVA analysis by using ln (x + 1) to meet assumptions of normality. Significant ANOVA effects were subjected to pairwise comparison by Tukey post-hoc tests (P < 0.05).
Results
Oviposition preference
Two-choice tests. Induced resistance by MeJA had a significant effect on T. absoluta oviposition preference under the low (t = 2.50; df = 1, 17; P = 0.041) and optimal (t = 2.63; df = 1, 17; P = 0.034) fertilizer rates; females laid 53% and 46% more eggs, respectively, on non-induced (control) plants than on MeJA-induced plants ((a)). Although the trend in oviposition preference between MeJA-induced and control plants was similar at the medium and high fertilizer rates, the difference was not significant (Medium: t = 1.10; df = 1, 17; P = 0.308; High: t = 2.15; df = 1, 17; P = 0.068) ((a)).
Figure 1. Number of eggs laid by Tuta absoluta females (mean ± SE) on tomato plants exposed to four fertilizer regimes (L = low, M = medium, O = optimal, and H = high) with and without induction by methyl jasmonate (MeJA) in two-choice (a), four-choice (b), and multiple-choice tests (c). An asterisk (*) indicates significant differences between treatments. Different letters indicate significant differences among treatments (two-way analysis of variance, P < 0.05). n = 8; n.s, not significant.
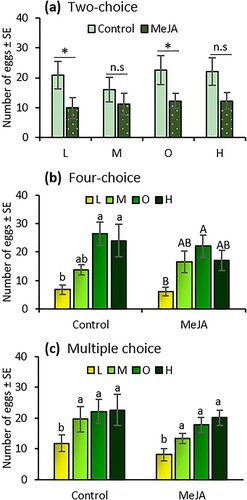
Four-choice tests. Fertilizer regime significantly affected T. absoluta oviposition preference on non-induced (control) plants (F = 9.33; df = 3, 31; P < 0.001) and on plants induced by MeJA (F = 4.81; df = 3, 31; P = 0.011). On non-induced plants, T. absoluta laid 51%, 74%, and 72% more eggs under medium, optimal, and high fertilizer rates, respectively, than at the low rate ((b)). Similarly, on MeJA-induced plants, the numbers of eggs laid were 64%, 72%, and 64% higher under medium, optimal, and high fertilizer rates, respectively, than at the low rate ((b)).
Multiple-choice tests. The amount of fertilizer significantly affected T. absoluta oviposition preference (F = 10.76; df = 3, 63; P < 0.001). Females laid 40%, 50%, and 54% more eggs on plants under the medium, optimal, and high fertilizer rates, respectively, than at the lower rate ((c)). In contrast, MeJA application had no effect on adult oviposition preference (F = 2.88; df = 1, 63; P = 0.096), and there was no interaction effect between fertilizer rates and induced resistance by MeJA (F = 0.54; df = 3, 63; P = 0.657).
Offspring performance
Survival. Fertilizer rates affected larval survival of T. absoluta significantly (F = 2.85; df = 3, 103; P = 0.041). Larval survival was 13%, 21%, and 16% greater on plants under the medium, optimal, and high fertilizer rates, respectively, than at the lower rate ((a)). However, MeJA application had no effect on larval survival (F = 1.92; df = 1, 103; P = 0.169), and there was no interaction effect between fertilizer rates and induced resistance by MeJA (F = 0.40; df = 3, 103; P = 0.755).
Figure 2. Larval survival (percent ± SE) (a), developmental time from egg to pupa (mean days ± SE) (b), and pupal weights (mean mg ± SE) (c) of Tuta absoluta feeding on tomato plants exposed to different fertilizer regimes (L = low, M = medium, O = optimal, and H = high) with and without induction by methyl jasmonate (MeJA). Uppercase letters indicate significant differences between induced and non-induced plants, while lowercase letters indicate significant difference among fertilizer regimes (two-way analysis of variance, P < 0.05). n = 13.
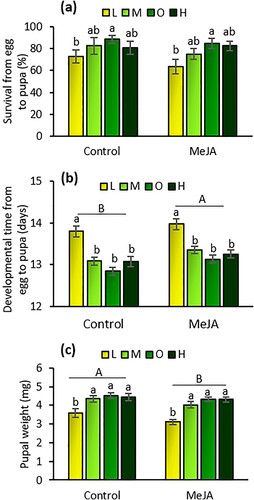
Larval developmental time. The amount of fertilizer (F = 18.83; df = 3, 103; P < 0.001) and induced resistance by MeJA (F = 6.47; df = 1, 103; P < 0.01) had a significant effect on T. absoluta developmental time. Larval developmental time was 5‒6.5% longer when plants received low fertilizer rates than under the medium, optimal, and high rates ((b)). Induced resistance by MeJA also significantly extended larval developmental times by 1.7% compared to control plants. There was no interaction effect between fertilizer rates and induction (F = 0.11; df = 3, 103; P = 0.952).
Pupal weight. Both fertilizer rates (F = 20.73; df = 3, 103; P < 0.001) and induced resistance by MeJA (F = 6.62; df = 1, 103; P = 0.012), but not their interaction (F = 0.44; df = 3, 103; P = 0.723), had a significant effect on T. absoluta pupal weight. Pupal weights were 20%, 24%, and 24% higher in plants under the medium, optimal, and high fertilizer rates, respectively, than at the lower rate ((c)). In contrast, pupal weights were 7% lower in plants induced by MeJA than in non-induced plants ((c)).
Plant growth
Plant dry weight was significantly affected by fertilizer rates (F = 16.34; df = 3, 63; P < 0.001). Plant dry weight under the optimal fertilizer input was 53%, 20%, and 23% higher than at the low, medium, and high rates ((a)). Plants under the low fertilizer rate had the lowest weight of all the treatments. There were no effects of induced resistance by MeJA (F = 0.01; df = 1, 63; P = 0.919) or an interaction effect between fertilizer and induction on the dry weight of tomato plants (F = 0.18; df = 3, 63; P = 0.906).
Figure 3. Dry weight (mean g ± SE) (a) and height (mean cm ± SE) (b) of tomato plants exposed to four fertilizer regimens (L = low, M = medium, O = optimal, and H = high) with and without induction by methyl jasmonate (MeJA). Different letters indicate significant differences among treatments (two-way analysis of variance, P < 0.05). n = 8.
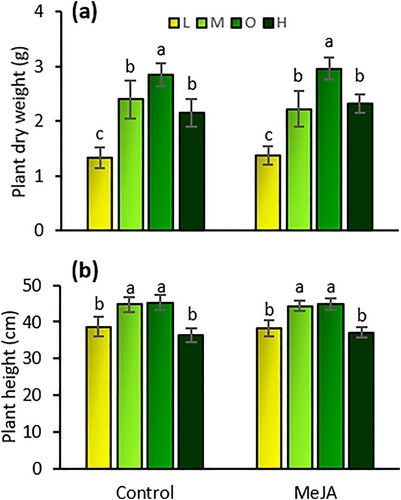
Plant height was also significantly affected by fertilizer rates (F = 35.0; df = 3,63; P < 0.001). Plant height under the optimal and medium fertilizer inputs was higher than at the low and high rates ((b)). There were no effects of MeJA induction (F = 0.02; df = 3,63; P = 0.896) or an interaction effect between fertilizer and induction on the height of tomato plants (F = 0.15; df = 3,63; P = 0.926)
Soil nitrogen levels
As expected, higher fertilizer rates led to significantly higher nitrogen levels in the soil (F = 42.64; df = 1, 40; P < 0.001) (). Also, as expected, there were no effects of MeJA induction (F = 0.22; df = 1, 40; P = 0.805) or an interaction effect between fertilizer regime and induction on the level of nitrogen in the soil (F = 0.65; df = 3, 40; P = 0.937).
Figure 4. Percentage of nitrogen in the soil (mean percent ± SE) exposed to four fertilizer regimens (L = low, M = medium, O = optimal, and H = high) with and without induction by methyl jasmonate (MeJA). Different letters indicate significant differences between treatments (two-way analysis of variance, P < 0.05). n = 5.
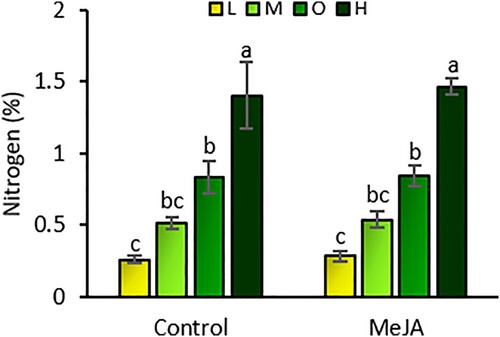
Nutrient levels in leaves
The amount of fertilizer affected the levels of nutrients in tomato leaves (Wilks’ Lambda = 0.058; F = 7.416; df = 18, 76; P < 0.001). However, neither MeJA induction (Wilks’ Lambda = 0.726; F = 1.698; df = 6, 27; P = 0.160) nor the interaction between fertilizer and induction (Wilks’ Lambda = 0.844; F = 0.263; df = 18, 76; P = 0.999) affected nutrient levels. Levels of nitrogen, phosphorus, potassium, calcium, and magnesium in leaf tissue were significantly altered by fertilizer rates (). Plants under high fertilizer rates had 34%, 15%, and 12% higher levels of nitrogen than under low, medium, and optimal rates, respectively ((a)). In the same way, phosphorus levels in high fertilizer plants were 47%, 34%, and 25% higher than at the low, medium, and optimal rates, respectively ((b)). Plants under the optimal fertilizer rate had 20%, 10%, and 10% higher concentration of potassium than the low, medium, and high rates, respectively ((c)). Plants under the low fertilizer rate had the lowest levels of nitrogen, phosphorus, and potassium of all fertilizer treatments ((c)). Conversely, plants under the low fertilizer rate had the highest concentration of calcium and magnesium in leaf tissue of all fertilizer treatments ((d,e)). In both cases, plants under the high fertilizer rate had the lowest concentration of calcium and magnesium. The amount of fertilizer did not affect sulfur concentration in leaves (, (f)).
Figure 5. Concentrations of nitrogen (a), phosphorus (b), potassium (c), calcium (d), magnesium (e), and sulfur (f) in the leaves of tomato plants (mean g/kg ± SE) exposed to four fertilizer regimens (L = low, M = medium, O = optimal, and H = high) with and without induction by methyl jasmonate (MeJA). Different letters indicate significant differences among treatments (two-way multivariate analysis of variance, P < 0.05). n = 5.
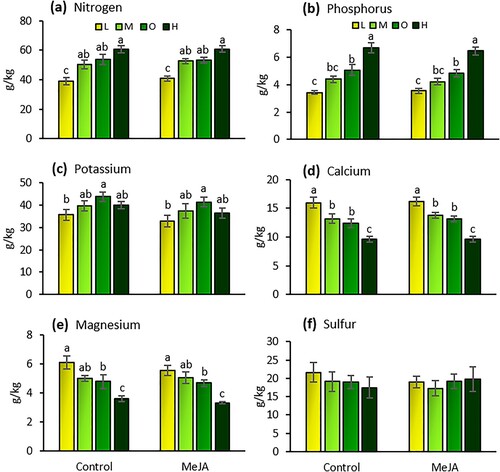
Table 1. Results of two-way analysis of variance (ANOVA) for the effects of fertilizer rates (‘Fertilizer’) and induction by methyl jasmonate (‘MeJA’), and the interaction between these two factors on the levels of nutrients in tomato leaves.
Total phenolics
Total phenolic levels in leaf tissue were not affected by fertilizer rates (F = 1.79; df = 3, 39; P = 0.169). However, MeJA increased total phenolic levels by 14% compared to non-induced plants (F = 5.67; df = 1, 39; P = 0.023) (). There was no interaction effect between fertilizer rates and MeJA induction on the levels of total phenolics in tomato plants (F = 0.05; df = 3, 39; P = 0.985).
Figure 6. Total phenolic content (mean mg de chlorogenic acid/g fresh tissue ± SE) in tomato leaves exposed to four fertilizer regimes (L = low, M = medium, O = optimal, and H = high) with and without induction by methyl jasmonate (MeJA). Different letters indicate significant differences between treatments (two-way analysis of variance, P < 0.05). n = 5.
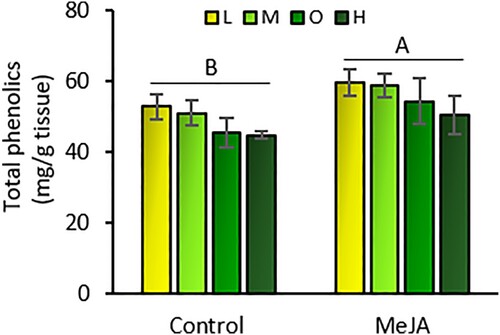
Volatile emissions
Volatile analyses identified 14 compounds among all treatments. Volatile emissions were affected by MeJA induction (Wilks’ Lambda = 0.371; F = 2.305; df = 14, 19; P = 0.046) but not by fertilizer regime (Wilks’ Lambda = 0.15; F = 1.217; df = 42, 57; P = 0.243) or the interaction between fertilizer regime and MeJA induction (Wilks’ Lambda = 0.146; F = 1.244; df = 42, 57; P = 0.220). MeJA induction increased total volatile emissions and the emissions of 12 terpene compounds (α-pinene, β-pinene, β-myrcene, 2-carene, α-phellandrene, α-terpinene, o-cymene, β-phellandrene, limonene, β-ocimene, γ-terpinene, and β-caryophyllene) in tomato plants compared to non-induced plants (; ).
Figure 7. Amounts (mean ng/g fresh tissue ± SE) of (Z)-3-hexenol (a), α-pinene (b), β-pinene (c), β-myrcene (d), 2-carene (e), α-phellandrene (f), α-terpinene (g), o-cymene (h), β-phellandrene (i), limonene (j), β-ocimene (k), γ-terpinene (l), β-caryophyllene (m), humulene (n), and total volatiles (o) emitted from tomato plants under four fertilizer regimes (L = low, M = medium, O = optimal, and H = high) with and without induction by methyl jasmonate (MeJA). Means followed by different letters indicate significant differences among treatments (GLM, P < 0.05). n = 5.
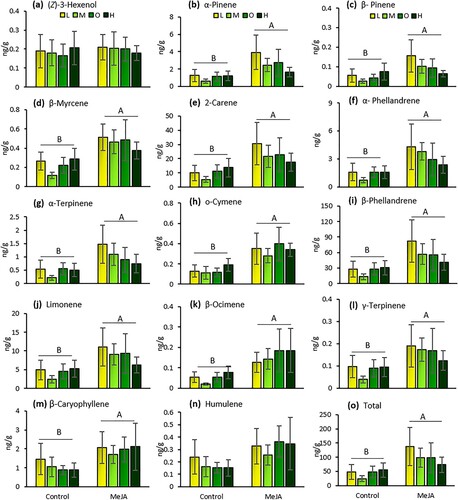
Table 2. Results of two-way analysis of variance (ANOVA) for the effects of fertilizer rates (‘Fertilizer’) and induction by methyl jasmonate (‘MeJA’), and the interaction between these two factors on the amounts of volatiles emitted from tomato plants.
Discussion
Although plants often deal with multiple stresses that can affect their growth and defensive traits and subsequently affect their interactions with herbivores (Chapin et al. Citation1987), most research has focused on understanding these interactions under a single stress condition. Here, we explored how nutrient availability, a potential abiotic stress, modules induced resistance triggered by the elicitor MeJA against T. absoluta in tomato plants. Our study demonstrated that (1) fertilization increased leaf nutrient content of nitrogen, phosphorus, and potassium (NPK), which resulted in greater preference and performance of T. absoluta, but had no effect on defensive traits (total phenolic and volatile emissions); (2) MeJA increased levels of defensive traits, which correlated with decreased preference and performance of T. absoluta; and (3) fertilization did not interact with MeJA to affect plant growth and nutrient content, defensive traits, or the preference and performance of T. absoluta.
Fertilization triggered bottom-up effects that influenced tomato-T. absoluta interactions. In our study, tomato plants under an optimal fertilizer rate had higher growth rates than plants under insufficient (i.e. below optimal) and excessive (i.e. above optimal) fertilizer rates. Nutrient deficiencies can negatively affect plant growth, while an excess of nutrients can be associated with high soluble salt concentrations that can interfere with plant growth (Marschner Citation1983). These bottom-up effects positively affected T. absoluta. In accordance with the preference-performance (or ‘mother knows best’) hypothesis (Jaenike Citation1978), we observed a strong correlation between T. absoluta oviposition preference and larval performance. Females preferred to oviposit and larvae performed better, i.e. had increased survival rates, reduced developmental times, and higher pupal weights, on plants under optimal and high nutrient content. By contrast, plants with low fertilization rates showed low oviposition preference by females and reduced larval performance. These findings were expected since the development success of insect herbivores is often positively correlated with the nitrogen content in their host plants (Sétamou et al. Citation1993; Awmack and Leather Citation2002; Shah Citation2017). In fact, recent studies have also shown that T. absoluta females prefer to oviposit on host plants that enhance their offspring performance (Sylla et al. Citation2019; Silva et al. Citation2021). Tuta absoluta females likely respond behaviorally to visual and chemical changes in host plants due to fertilization, which is an example of antixenosis triggered by bottom-up effects. Interestingly, fertilization rates above optimal reduced plant growth even when nitrogen levels in the soil and leaves were the highest; however, despite this increase in nitrogen levels, T. absoluta preference and performance were comparable between optimal and high fertilizer rates, indicating that too much fertilizer did not benefit either the plant or the pest.
The positive effects of fertilization on T. absoluta preference and performance can be attributed to changes in plant nutrients and secondary metabolites (Herms and Mattson Citation1992; Herms Citation2002). As expected, our study showed that plants under higher fertilizer rates had increased levels of NPK in their leaves. These three elements are essential for the proper growth and development of tomato plants (Adams Citation1986) and of insects (Dale Citation1988; Schoonhoven et al. Citation2005; Amtmann et al. Citation2008; Chan et al. Citation2021). For instance, nitrogen is positively correlated with amino acid and protein content and its deficiency may limit insect growth (Schoonhoven et al. Citation2005). Indeed, nitrogen deficiency to tomato plants has been shown to limit T. absoluta growth via bottom-up effects (Han et al. Citation2014). Potassium deficiency may disrupt protein synthesis, which can result in an increase in amino acids and a decrease in sugar content (Dale Citation1988), and phosphorus can also play an important role in plant resistance against herbivores (Chan et al. Citation2021). Higher fertilizer rates also decreased levels of calcium and magnesium, and these imbalances in macro-elements could trigger bottom-up effects that affect the performance of T. absoluta on tomato plants (Queiroz et al. Citation2022); however, compared to NPK, these two elements appear to be less critical to tomato-T. absoluta interactions because their levels were not correlated with T. absoluta preference and performance. As predicted by the resource availability hypothesis, we expected tomato plants under high fertilizer regimes to have low investment in anti-herbivore defenses (Coley Citation1987). However, our study did not show changes in levels of total phenolics (direct resistance) or volatile emissions (indirect resistance) in response to fertilization. It is possible that other resistance traits not measured here, such as specific phenolic compounds, glycoalkaloids, and defensive enzymes, could have increased under low nutrition since nitrogen content may affect the levels of these compounds in tomato plants (Han et al. Citation2016; Larbat et al. Citation2016; Becker et al. Citation2021).
Jasmonates (i.e. JA and MeJA) are elicitors of plant resistance against chewing herbivores such as caterpillars (Chen et al. Citation2005; Tortorici et al. Citation2022). Here, we demonstrated that T. absoluta females lay fewer eggs on tomato plants treated with MeJA. Previous studies showed that T. absoluta females prefer to oviposit on undamaged plants than on plants damaged by conspecific larvae (Anastasaki et al. Citation2018), which could be mediated by plant volatiles. Indeed, plant volatiles are cues used by females to locate suitable oviposition sites (Webster and Cardé Citation2017) and to avoid competition by conspecific and heterospecific herbivores (De Moraes et al. Citation2001; Knolhoff and Heckel Citation2014). In fact, tomato plants infested by herbivores altered the emission of volatiles, which modified T. absoluta oviposition (Anastasaki et al. Citation2018; Silva et al. Citation2022). In our study, MeJA-induced plants increased the emission of 12 terpene volatiles and many of them are also increased after T. absoluta larval feeding (Anastasaki et al. Citation2018; Silva et al. Citation2022). Likewise, tomato seeds treated with JA increased the emission of methyl salicylate and 4,8,12-trimethyltrideca-1,3,7,11-tetraene (Smart et al. Citation2013). By contrast, a decrease in volatile emission was found when tomato seeds were applied with a low concentration of MeJA (0.8 mM) (Strapasson et al. Citation2014). Our MeJA treatment increased volatile emissions during the daytime, which could attract the natural enemies of T. absoluta and thus increase indirect resistance in tomatoes. For instance, tomato plants damaged by T. absoluta emitted higher amounts of α-pinene, α -phellandrene, 3-carene, β-ocimene, and β-phellandrene that increased attraction of the predator Nesidiocoris tenuis (Reuter) (Ayelo et al. Citation2021). Additional studies are needed to determine if MeJA also induces volatile emissions in tomatoes at nighttime.
Previous studies showed that T. absoluta larval performance is negatively affected by MeJA application to tomato seeds (Strapasson et al. Citation2014). In accordance with the preference-performance hypothesis, our study showed that lower oviposition on MeJA-treated tomato plants is correlated with reduced pupal weights and increased larval developmental times. Our MeJA treatment increased total phenolic content in leaves, which could partially explain the reduced T. absoluta performance on these plants. Phenolic compounds play an important role in plant resistance against insect herbivores (Summers and Felton Citation1994; Wallis and Galarneau Citation2020). Other studies also report increases of defensive enzymes after MeJA treatment in tomato plants (Tan et al. Citation2012; Paudel et al. Citation2014). In our study, we used a MeJA concentration that induces a relatively low, but significant, defensive response in tomato plants against T. absoluta; however, whether this concentration induces a defensive response similar to that caused by herbivore damage remains unknown. It is also unclear whether stronger bottom-up effects of tomato plants on T. absoluta could be induced by higher concentrations of MeJA (> 2 mM), and if these effects could persist over a long period.
We expected potential trade-offs between resistance responses of plants when simultaneously exposed to abiotic and biotic stresses. However, our study showed no interactions between nutrient availability and jasmonate-mediated induced resistance such that each plant stressor acted independently to affect antixenotic and antibiotic resistance against T. absoluta in tomato plants, i.e. there were no fertilizer regime × MeJA induction interaction effects. This finding suggests that in our bottom-up tomato-T. absoluta system, resources allocated for jasmonate-mediated induced resistance did not compete with resources allocated for plant growth. Other studies have also attempted to identify trade-offs between nutrient availability and induced plant resistance and found variable evidence (i.e. negative, neutral, and positive) for these trade-offs (Stout et al. Citation1998; Cipollini and Bergelson Citation2001; Schmelz et al. Citation2003; Lou and Baldwin Citation2004; Mason et al. Citation2022; Wang et al. Citation2022). For example, limited nutrient availability reduced induced resistance in rapeseed plants, but not under high nutrient availability (Cipollini and Bergelson Citation2001). By contrast, low nutrient availability resulted in increased induction of defense-related plant terpenes and phytohormones in maize (Schmelz et al. Citation2003) and increased herbivore-induced defenses in cotton (Chen et al. Citation2008). Recent studies in maize showed that high nitrogen supply improves induced resistance (Wang et al. Citation2022), while low fertilization reduces induced defenses but only in a resistant genotype (Mason et al. Citation2022). These studies, together with ours, highlight that predicting the outcomes of bottom-up effects on induced resistance against herbivores can be difficult because these effects depend on many factors, such as the type and strength of the fertilizer treatment (i.e. amount, composition, and rate) and the inducer of plant resistance (i.e. mechanical, herbivory, or elicitor), as well as on the plant’s genotype. Future studies using standardized methodologies across multiple crops may help in better predicting the outcomes of these bottom-up effects.
In summary, we demonstrated that, under our testing conditions, the combined bottom-up effects of fertilization and MeJA independently affect the interactions between tomato plants and T. absoluta. Managing fertilizer inputs and the level of induced resistance in plants can be strategies to control insect pests in agroecosystems (Han et al. Citation2022). For instance, we found a lower preference and performance of T. absoluta on tomato plants under low fertilizer inputs and on plants treated with MeJA, which was correlated with lower nutrient content and higher levels of induced phenolics and terpene emissions, respectively. These two treatments could, however, negatively affect plant growth and yield. Although low fertilizer regimes are expected to reduce plant growth and yield, the effects of jasmonates on tomato growth and yield are not clear (Thaler Citation1999; Redman et al. Citation2001). It is even less clear how simultaneous stressors, such as limited nutrient availability and the activation of induced resistance, affect crop yield. In our study, T. absoluta performed well even at half the optimal fertilizer rate, which suggests that lower rates would be needed for its control but to the detriment of plant growth. In contrast, MeJA-induced resistance reduced T. absoluta preference and performance without any negative (short term) effects on plant growth, which suggests that manipulating induced resistance in tomatoes could serve as a potential control strategy against this pest. Moreover, it is important to examine how bottom-up effects on induced resistance influence plant interactions with multiple herbivores and their natural enemies (Strapasson et al. Citation2014; Becker et al. Citation2021). Our study was conducted in the greenhouse under controlled conditions; therefore, further studies are needed to determine if the bottom-up effects we observed here are consistent under field conditions. Improving our understanding on methods to manipulate plant nutrient and defense levels can help develop novel IPM strategies against herbivores, such as T. absoluta, in agroecosystems.
Supplemental Material
Download MS Word (33.5 KB)Acknowledgements
We thank Drs. José R. P. Parra and Flávio C. Montes for assistance with rearing T. absoluta in the laboratory. We also thank two anonymous reviewers for helpful comments on an earlier draft of the manuscript.
Additional information
Funding
Notes on contributors
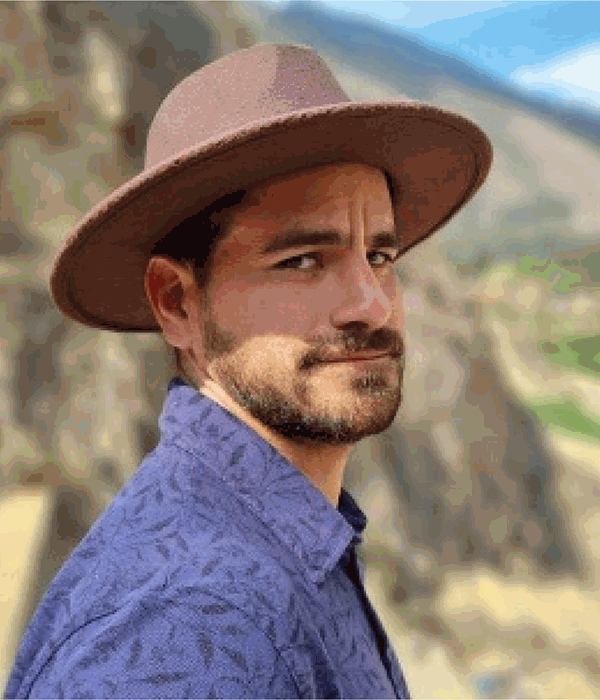
Paolo Salazar-Mendoza
Paolo Salazar-Mendoza is a PhD student in the Department of Entomology and Acarology, ‘Luiz de Queiroz’ College of Agriculture, at the University of São Paulo, Brazil. He is studying the effects of domestication, nutrient availability, and induced resistance on tomato plant defenses against the South American tomato pinworm Tuta absoluta.
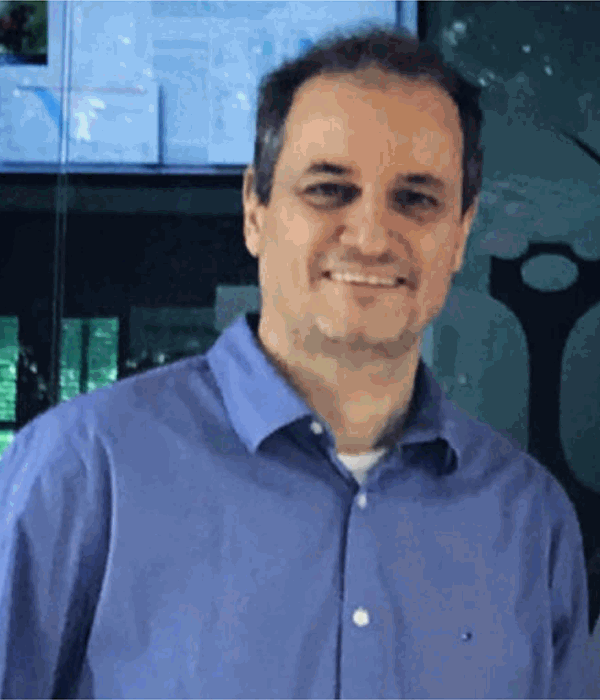
José Maurício S. Bento
José Maurício S. Bento is a Full Professor in the Department of Entomology and Acarology, ‘Luiz de Queiroz’ College of Agriculture, at the University of São Paulo, Brazil. His research comprises chemical communication in insects and plant-insect-natural enemy interactions.
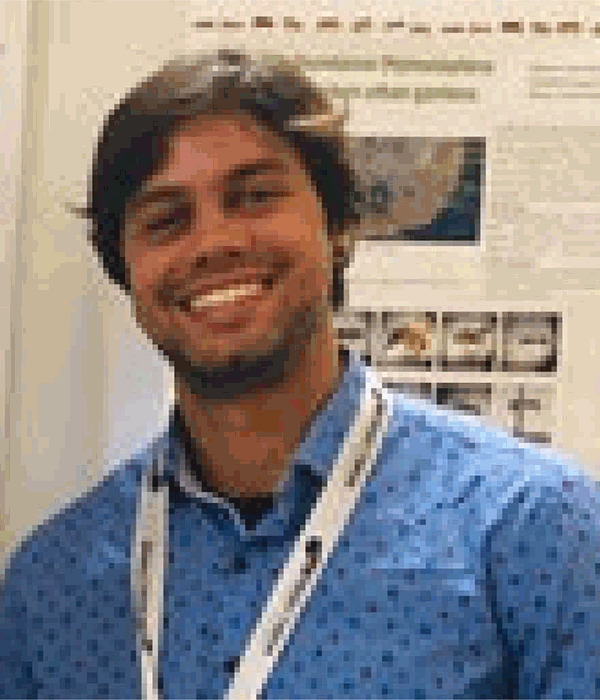
Diego B. Silva
Diego B. Silva is an Associate Scientist at Provivi, Campinas, Brazil. He was a Post-doctoral Researcher in the Department of Entomology at Rutgers University and in the Department of Entomology and Acarology, ‘Luiz de Queiroz’ College of Agriculture, at University of São Paulo, Brazil. His research interests include chemical ecology, biological control, and integrated pest management.
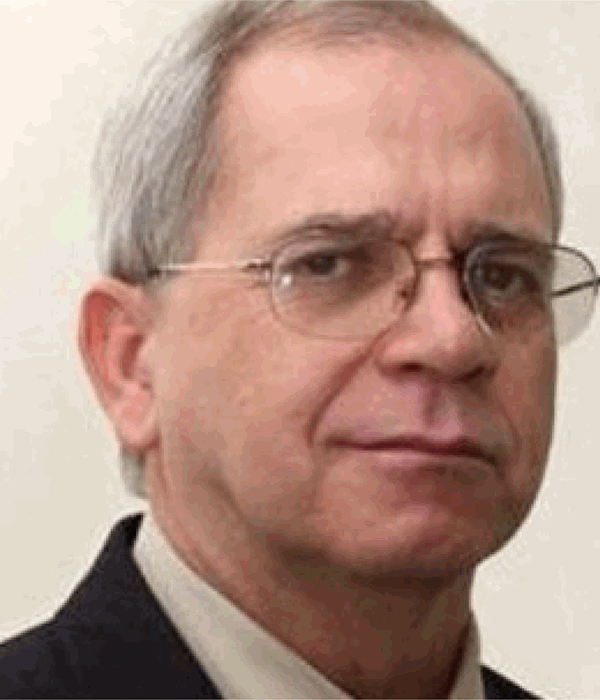
Sérgio F. Pascholati
Sérgio F. Pascholati is a Senior Professor in the Plant Pathology and Nematology Program, ‘Luiz de Queiroz’ College of Agriculture, at University of São Paulo, Brazil. His research interests include plant disease physiology and biochemistry, biological control of plant pathogens, and induced plant resistance to pathogens.
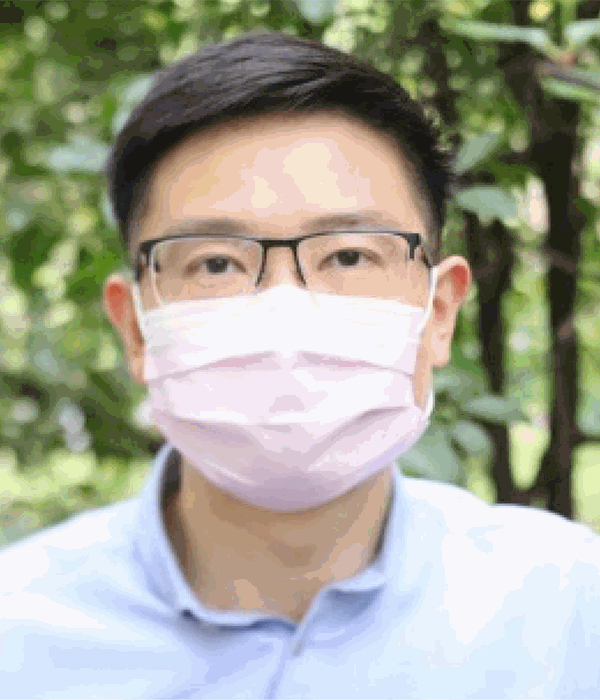
Peng Han
Peng Han is a Full professor in the School of Ecology and Environmental Sciences at Yunnan University, China. His research interests include: (i) revealing the bottom-up effects of environmental variables on plant-insect herbivore-natural enemy tri-trophic interactions, and (ii) monitoring, risk assessment and integrated management of invasive alien species.
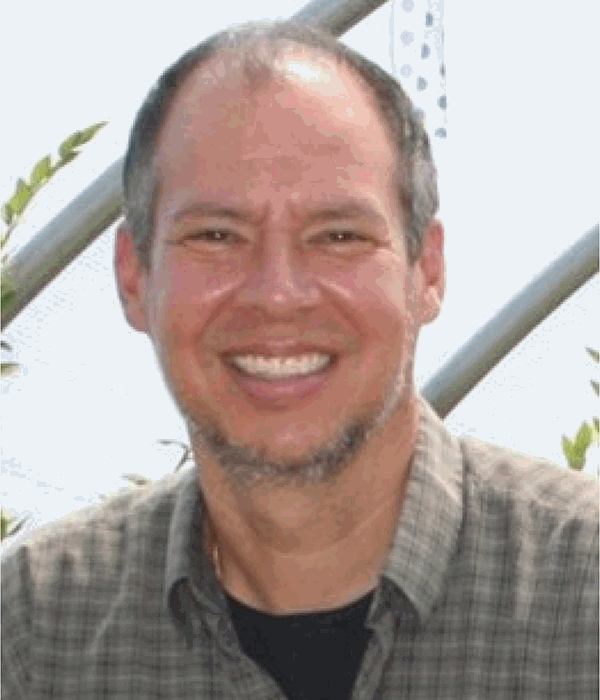
Cesar Rodriguez-Saona
Cesar Rodriguez-Saona is a Full Professor and Extension Specialist in the Department of Entomology at Rutgers University. He obtained his Masters from Oregon State University and his PhD from the University of California, Riverside. His areas of research include chemical ecology, insect-plant interactions, tri-trophic interactions, and host-plant resistance.
References
- Acevedo FE, Rivera-Vega LJ, Chung SH, Ray S, Felton G. 2015. Cues from chewing insects – the intersection of DAMPs, HAMPs, MAMPs and effectors. Curr Opin Plant Biol. 26:80–86.
- Adams P. 1986. Mineral nutrition. In: Atherton JG, Rudich J, editors. The tomato crop. Dordrecht: Springer; p. 281–334.
- Ainsworth EA, Gillespie KM. 2007. Estimation of total phenolic content and other oxidation substrates in plant tissues using Folin–Ciocalteu reagent. Nat Protoc. 2(4):875–877.
- Alba JM, Glas JJ, Schimmel CJ, Kant MR. 2011. Avoidance and suppression of plant defenses by herbivores and pathogens. J Plant Interact. 6(4):221–227.
- Amtmann A, Troufflard S, Armengaud P. 2008. The effect of potassium nutrition on pest and disease resistance in plants. Physiol Plant. 133(4):682–691.
- Anastasaki E, Drizou F, Milonas PG. 2018. Electrophysiological and oviposition responses of Tuta absoluta females to herbivore-induced volatiles in tomato plants. J Chem Ecol. 44(3):288–298.
- Awmack CS, Leather SR. 2002. Host plant quality and fecundity in herbivorous insects. Annu Rev Entomol. 47(1):817–844.
- Ayelo PM, Yusuf AA, Pirk CW, Chailleux A, Mohamed SA, Deletre E. 2021. Terpenes from herbivore-induced tomato plant volatiles attract Nesidiocoris tenuis (Hemiptera: Miridae), a predator of major tomato pests. Pest Manag Sci. 77(11):5255–5267.
- Becker C, Han P, deCampos MR, Béarez P, Thomine E, L Bot, Adamowicz J, Brun S, Fernandez R, Desneux X, et al. 2021. Feeding guild determines strength of top-down forces in multitrophic system experiencing bottom-up constraints. Sci Total Environ. 793:148544.
- Bezemer TM, Van Dam NM. 2005. Linking aboveground and belowground interactions via induced plant defenses. Trends Ecol Evol. 20(11):617–624.
- Blazhevski S, Kalaitzaki AP, Tsagkarakis AE. 2018. Impact of nitrogen and potassium fertilization regimes on the biology of the tomato leaf miner Tuta absoluta. Entomol Gen. 37(2):157–174.
- Bray HG, Thorpe WV. 1954. Analysis of phenolic compounds of interest in metabolism. Methods Biochem Anal. 1:27–52.
- Cao HH, Wang SH, Liu TX. 2014. Jasmonate-and salicylate-induced defenses in wheat affect host preference and probing behavior but not performance of the grain aphid, Sitobion avenae. Insect Sci. 21(1):47–55.
- Chan C, Liao YY, Chiou TJ. 2021. The impact of phosphorus on plant immunity. Plant Cell Physiol. 62(4):582–589.
- Chapin FS, Bloom AJ, Field CB, Waring RH. 1987. Plant responses to multiple environmental factors. Bioscience. 37(1):49–57.
- Chen H, Wilkerson CG, Kuchar JA, Phinney BS, Howe GA. 2005. Jasmonate-inducible plant enzymes degrade essential amino acids in the herbivore midgut. Proc Nat Acad Sci. 102(52):19237–19242.
- Chen Y, Olson DM, Ruberson JR. 2010. Effects of nitrogen fertilization on tritrophic interactions. Arthropod Plant Interact. 4(2):81–94.
- Chen Y, Schmelz EA, Wäckers F, Ruberson JR. 2008. Cotton plant, Gossypium hirsutum L., defense in response to nitrogen fertilization. J Chem Ecol. 34:1553–1564.
- Cipollini D, Bergelson J. 2001. Plant density and nutrient availability constrain constitutive and wound-induced expression of trypsin inhibitors in Brassica napus. J Chem Ecol. 27(3):593–610.
- Coley PD. 1987. Interspecific variation in plant anti-herbivore properties: the role of habitat quality and rate of disturbance. New Phytol. 106:251–263.
- Dale JE. 1988. The control of leaf expansion. Annu Rev Plant Physiol. 39(1):267–295.
- de Lange ES, Kyryczenko-Roth V, Johnson-Cicalese J, Davenport J, Vorsa N, Rodriguez-Saona C. 2019. Increased nutrient availability decreases insect resistance in cranberry. Agric Forest Entomol. 21(3):326–335.
- De Moraes CM, Mescher MC, Tumlinson JH. 2001. Caterpillar-induced nocturnal plant volatiles repel conspecific females. Nature. 410(6828):577–580.
- Desneux N, Wajnberg E, Wyckhuys KA, Burgio G, Arpaia S, Narváez-Vasquez CA, Gonzáles-Cabrera J, Ruescas DC, Tabone E, Frandon J, et al. 2010. Biological invasion of European tomato crops by Tuta absoluta: ecology, geographic expansion and prospects for biological control. J Pest Sci. 83(3):197–215.
- Desneux N, Han P, Mansur R, Arnó J, Brévault T, Campos MR, Chailleux A, Guedes RNC, Karimi J, Lavoir AV, et al. 2022. Integrated pest management of Tuta absoluta: practical implementations across different world regions. J Pest Sci. 95:17–39.
- Erb M, Meldau S, Howe GA. 2012. Role of phytohormones in insect-specific plant reactions. Trends Plant Sci. 17(5):250–259.
- Eyles A, Bonello P, Ganley R, Mohammed C. 2010. Induced resistance to pests and pathogens in trees. New Phytol. 185(4):893–908.
- Gershenzon J. 1994. Metabolic costs of terpenoid accumulation in higher plants. J Chem Ecol. 20(6):1281–1328.
- Hahn PG, Maron JL. 2016. A framework for predicting intraspecific variation in plant defense. Trends Ecol Evol. 31(8):646–656.
- Han P, Bayram Y, Shaltiel-Harpaz L, Sohrabi F, Saji A, Esenali UT, Jalilov A, Ali A, Shashank PR, Ismoilov K, et al. 2019. Tuta absoluta continues to disperse in Asia: damage, ongoing management and future challenges. J Pest Sci. 92(4):1317–1327.
- Han P, Becker C, Sentis A, Rostás M, Desneux N, Lavoir AV. 2019. Global change-driven modulation of bottom–up forces and cascading effects on biocontrol services. Curr Opin Insect Sci. 35:27–33.
- Han P, Desneux N, Amiens-Desneux E, Le Bot J, Bearez P, Lavoir AV. 2016. Does plant cultivar difference modify the bottom-up effects of resource limitation on plant-insect herbivore interactions? J Chem Ecol. 42:1293–1303.
- Han P, Lavoir AV, Le Bot J, Amiens-Desneux E, Desneux N. 2014. Nitrogen and water availability to tomato plants triggers bottom-up effects on the leafminer Tuta absoluta. Sci Rep. 4(1):1–8.
- Han P, Lavoir AV, Rodriguez-Saona C, Desneux N. 2022. Bottom-up forces in agroecosystems and their potential impact on arthropod pest management. Annu Rev Entomol. 67:239–259.
- Herms D, Mattson W. 1992. The dilemma of plants: to grow or defend. Q Rev Biol. 67:283–335.
- Herms DA. 2002. Effects of fertilization on insect resistance of woody ornamental plants: reassessing an entrenched paradigm. Environ Entomol. 31(6):923–933.
- Hou W, Tränkner M, Lu J, Yan J, Huang S, Ren T, Cong R, Li X. 2019. Interactive effects of nitrogen and potassium on photosynthesis and photosynthetic nitrogen allocation of rice leaves. BMC Plant Biol. 19(1):1–13.
- Howe GA, Jander G. 2008. Plant immunity to insect herbivores. Annu Rev Plant Biol. 59(1):41–66.
- Hunter MD, Price PW. 1992. Playing chutes and ladders: heterogeneity and the relative roles of bottom-up and top-down forces in natural communities. Ecology. 73:724–732.
- Islam MN, Hasanuzzaman ATM, Zhang ZF, Zhang Y, Liu TX. 2017. High level of nitrogen makes tomato plants releasing less volatiles and attracting more Bemisia tabaci (Hemiptera: Aleyrodidae). Front Plant Sci. 8:466.
- Jaenike J. 1978. On optimal oviposition behavior in phytophagous insects. Theor Popul Biol. 14:350–356.
- Joern A, Provin T, Behmer ST. 2012. Not just the usual suspects: insect herbivore populations and communities are associated with multiple plant nutrients. Ecology. 93(5):1002–1015.
- Karban R, Myers JH. 1989. Induced plant responses to herbivory. Annu Rev Ecol Evol Syst. 20:331–348.
- Kessler A, Baldwin IT. 2001. Defensive function of herbivore-induced plant volatile emissions in nature. Science. 291(5511):2141–2144.
- Kessler A, Baldwin IT. 2002. Plant responses to insect herbivory. Annu Rev Plant Biol. 53:299–328.
- Knolhoff LM, Heckel DG. 2014. Behavioral assays for studies of host plant choice and adaptation in herbivorous insects. Ann Rev Entomol. 59(1):263–278.
- Kofalvi SA, Nassuth A. 1995. Influence of wheat streak mosaic virus infection on phenylpropanoid metabolism and the accumulation of phenolics and lignin in wheat. Physiol Mol. 47(6):365–377.
- Larbat R, Adamowicz S, Robin C, Han P, Desneux N, Le Bot J. 2016. Interrelated responses of tomato plants and the leaf miner Tuta absoluta to nitrogen supply. Plant Biol. 18(3):495–504.
- Li Z, Xu B, Du T, Ma Y, Tian X, Wang F, Wang W. 2021. Excessive nitrogen fertilization favors the colonization, survival, and development of Sogatella furcifera via bottom-up effects. Plants. 10(5):875.
- Lou Y, Baldwin IT. 2004. Nitrogen supply influences herbivore-induced direct and indirect defenses and transcriptional responses in Nicotiana attenuata. Plant Physiol. 135(1):496–506.
- Mansour R, Brévault T, Chailleux A, Cherif A, Grissa-Lebdi K, Haddi K, Sa M, Nofemela RS, Oke A, Sylla S. 2018. Occurrence, biology, natural enemies and management of Tuta absoluta in Africa. Entomol Gen. 38(2):83–112.
- Marschner H. 1983. General introduction to the mineral nutrition of plants. In: Läuchli A, Bieleski RL, editors. Inorganic plant nutrition. Berlin: Springer; p. 5–60.
- Mason CJ, Ray S, Davidson-Lowe E, Ali JG, Luthe DS, Felton G. 2022. Plant Nutrition influences resistant maize defense responses to the fall armyworm (Spodoptera frugiperda). Front Ecol Evol. 10:844274.
- Minitab. 2013. Minitab computer software. State College (PA): Minitab Inc.
- Nelson DW, Sommers LE. 1980. Total nitrogen analysis of soil and plant tissues. J Assoc Off Anal Chem. 63:770–778.
- Paudel S, Rajotte EG, Felton GW. 2014. Benefits and costs of tomato seed treatment with plant defense elicitors for insect resistance. Arthropod Plant Interact. 8(6):539–545.
- Poelman EH. 2015. From induced resistance to defence in plant-insect interactions. Entomol Exp Appl. 157(1):11–17.
- Power ME. 1992. Top-down and bottom-up forces in food webs: do plants have primacy. Ecology. 73(3):733–746.
- Proffit M, Birgersson G, Bengtsson M, Reis R Jr, Witzgall P, Lima E. 2011. Attraction and oviposition of Tuta absoluta females in response to tomato leaf volatiles. J Chem Ecol. 37:565–574.
- Queiroz RB, Lopes MC, Costa TL, da Silva RS, Galdino TV, Gontijo PDC, Martinez HEP, Picanço MC. 2022. Influence of tomato plants nutritional status on the fitness and damage of Tuta absoluta (Lepidoptera: Gelechiidae). Agric Forest Entomol. 24(2):260–266.
- R Core Team. 2022. R: a language and environment for statistical computing. Vienna: R Foundation for Statistical Computing. [accessed 2022 August]. https://www.r-project.org/.
- Redman AM, Cipollini DF, Schultz JC. 2001. Fitness costs of jasmonic acid-induced defense in tomato, Lycopersicon esculentum. Oecologia. 126(3):380–385.
- Rodriguez-Saona C, Crafts-Brandner SJ, Paré PW, Henneberry TJ. 2001. Exogenous methyl jasmonate induces volatile emissions in cotton plants. J Chem Ecol. 27(4):679–695.
- Schmelz EA, Alborn HT, Engelberth J, Tumlinson JH. 2003. Nitrogen deficiency increases volicitin-induced volatile emission, jasmonic acid accumulation, and ethylene sensitivity in maize. Plant Physiol. 133(1):295–306.
- Schoonhoven LM, Van Loon B, van Loon JJ, Dicke M. 2005. Insect-plant biology. New York (NY): Oxford University Press Inc.
- Sétamou M, Schulthess F, Bosque-Pérez NA, Thomas-Odjo A. 1993. Effect of plant nitrogen and silica on the bionomics of Sesamia calamistis (Lepidoptera: Noctuidae). Bull Entomol Res. 83(3):405–411.
- Shah TH. 2017. Plant nutrients and insects development. Int J Entomol Res. 2(6):54–57.
- Silva DB, Hanel A, Franco FP, de Castro Silva-Filho M, Bento JMS. 2022. Two in one: the neotropical mirid predator Macrolophus basicornis increases pest control by feeding on plants. Pest Manag Sci. 78(8):3314–3323.
- Silva GA, Queiroz EA, Arcanjo LP, Lopes MC, Araújo TA, Galdino TS, Samuels RI, Rodrigues-Silva N, Picanço MC. 2021. Biological performance and oviposition preference of tomato pinworm Tuta absoluta when offered a range of Solanaceous host plants. Sci Rep. 11(1):1–10.
- Smart LE, Martin JL, Limpalaër M, Bruce TJ, Pickett JA. 2013. Responses of herbivore and predatory mites to tomato plants exposed to jasmonic acid seed treatment. J Chem Ecol. 39(10):1297–1300.
- Stella de Freitas TF, Stout MJ, Sant'Ana J. 2019. Effects of exogenous methyl jasmonate and salicylic acid on rice resistance to Oebalus pugnax. Pest Manag Sci. 75(3):744–752.
- Stout MJ, Brovont RA, Duffey SS. 1998. Effect of nitrogen availability on expression of constitutive and inducible chemical defenses in tomato, Lycopersicon esculentum. J Chem Ecol. 24(6):945–963.
- Strapasson P, Pinto-Zevallos DM, Paudel S, Rajotte EG, Felton GW, Zarbin PH. 2014. Enhancing plant resistance at the seed stage: low concentrations of methyl jasmonate reduce the performance of the leaf miner Tuta absoluta but do not alter the behavior of its predator Chrysoperla externa. J Chem Ecol. 40(10):1090–1098.
- Summers CB, Felton GW. 1994. Prooxidant effects of phenolic acids on the generalist herbivore Helicoverpa zea (Lepidoptera: Noctuidae): potential mode of action for phenolic compounds in plant anti-herbivore chemistry. Insect Biochem Mol Biol. 24(9):943–953.
- Sylla S, Brévault T, Monticelli LS, Diarra K, Desneux N. 2019. Geographic variation of host preference by the invasive tomato leaf miner Tuta absoluta: implications for host range expansion. J Pest Sci. 92(4):1387–1396.
- Tan CW, Chiang SY, Ravuiwasa KT, Yadav J, Hwang SY. 2012. Jasmonate-induced defenses in tomato against Helicoverpa armigera depend in part on nutrient availability, but artificial induction via methyl jasmonate does not. Arthropod-Plant Interact. 6(4):531–541.
- Thaler JS. 1999. Induced resistance in agricultural crops: effects of jasmonic acid on herbivory and yield in tomato plants. Environ Entomol. 28(1):30–37.
- Thaler JS, Stout MJ, Karban R, Duffey SS. 2001. Jasmonate-mediated induced plant resistance affects a community of herbivores. Ecol Entomol. 26(3):312–324.
- Throop HL, Lerdau MT. 2004. Effects of nitrogen deposition on insect herbivory: implications for community and ecosystem processes. Ecosystems. 7:109–133.
- Tortorici S, Biondi A, Pérez-Hedo M, Larbat R, Zappalà L. 2022. Plant defences for enhanced integrated pest management in tomato. Ann Appl Biol. 180(3):328–337.
- Wallis CM, Galarneau ERA. 2020. Phenolic compound induction in plant-microbe and plant-insect interactions: a meta-analysis. Front Plant Sci. 11:580753.
- Wang W, Wang X, Liao H, Feng Y, Guo Y, Shu Y, Wang J. 2022. Effects of nitrogen supply on induced defense in maize (Zea mays) against fall armyworm (Spodoptera frugiperda). Int J Mol Sci. 23:10457.
- War AR, Paulraj MG, Ahmad T, Buhroo AA, Hussain B, Ignacimuthu S, Sharma HC. 2012. Mechanisms of plant defense against insect herbivores. Plant Signal Behav. 7(10):1306–1320.
- Webster B, Cardé RT. 2017. Use of habitat odour by host-seeking insects. Biol Rev. 92(2):1241–1249.
- Wei X, Vrieling K, Kim HK, Mulder PP, Klinkhamer PG. 2021. Application of methyl jasmonate and salicylic acid lead to contrasting effects on the plant’s metabolome and herbivory. Plant Sci. 303:110784.
- Yu H, Zhang Y, Li Y, Lu Z, Li X. 2018. Herbivore-and MeJA-induced volatile emissions from the redroot pigweed Amaranthus retroflexus Linnaeus: their roles in attracting Microplitis mediator (Haliday) parasitoids. Arthropod Plant Interact. 12(4):575–589.
- Zarcinas BA, Cartwright B, Spouncer LR. 1987. Nitric acid digestion and multi-element analysis of plant material by inductively coupled plasma spectrometry. Commun Soil Sci Plant Anal. 18:131–146.