ABSTRACT
Over the last decade, the superior properties of graphene have contributed to intensive studies on the fabrication and applications of graphene nanocomposites. Ex-situ homologous recombination and recombination techniques were listed. Because of their remarkable features, including thermal conductivity and high-specific area, graphene and its derivatives have a significant prospective for medical and biological applications, including drug delivery and bio-imaging. The usage of graphene-based nanomaterials is a hot topic in medicinal research. Many research studies have been performed on graphene-based composites, but only a few reviews have been published regarding their applications in the biomedical field and potential risk factors associated with human well-being and the environment. Hence, this review paper aims to provide in-depth information on ongoing knowledge and results about the properties of graphene-based composites. The discovery, developmental methods, structural properties, and synthesis of graphene nanomaterials have been discussed. After a brief description of the most common methods used for fabricating or extracting graphene derivatives, the main steps of graphene-based composite preparation are introduced. Applications of graphene-based composites in drug delivery, medical, and biomedical fields have been addressed. Finally, the future perspectives and challenges associated with the applications of graphene-based composites have been summarized.
Highlights
Compositions and characteristics of nanocomposite materials reinforced with graphene, graphene oxide, reduced graphene oxide, and modified graphene.
The synthesis method of graphene and the manufacturing of graphene-based composites.
Latest developments in graphene-based composites for biomedical applications.
Consequences of the large-scale production of graphene-based composites.
GRAPHICAL ABSTRACT
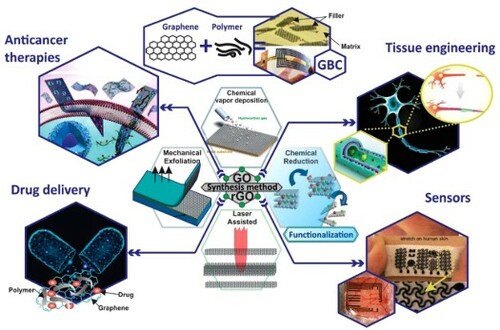
ABBREVIATIONS:
- CHI: chitosan
- CNT: carbon nanotubes
- CPT: camptothecin
- CVD: chemical vapor deposition
- Cy7: cyanines-7
- DOX: doxorubicin
- E. coli: Escherichia coli
- FET: field effect transistor
- FG: functionalized graphene
- FGO: functionalized graphene oxide
- FLG: few-layer graphene
- GO: graphene oxide
- GS: graphene sheets
- GBC: graphene-based composites
- HA: hyaluronic acid
- HRTEM: high resolution transmission electron microscope
- HyG: hydrogenated graphene
- IR: infrared
- LLB: layer by layer assembly
- nGO: nano graphene oxide
- PEG: polyethylene glycol
- PNIPAM: poly(N-isopropylacrylamide)
- rGO: reduced graphene oxide
- PBS: poly(butylene succinate)
- Rh2: ginsenoside
- PTT: photo-thermal therapy
- QD: quantum dots
Introduction
Graphene is a word used to describe a one-atom thick honeycomb of carbon. Graphene is, so far, the strongest known material regardless of diamond hardness and is more elastic than rubber (Citation1). Graphene and its related composites are very attractive fields of research thanks to graphene's unique properties, such as its electron mobility is almost 100 times faster that of silicon, its heat conductivity is two times that of diamond, and its electrical conductivity is 13 times that of copper. Moreover, graphene has an optical absorption of 2.3% leading to high transparency, and its hermetic in such a way that it does not allow even the smallest atom to pass through a sheet of defect-free (single-layer graphene). Graphene has been considered as a nanomaterial with potential biomedical applications for many years due to its well-known properties (e.g. firmness, high mechanical resistance, high electrical and thermal conductivity, etc.). However, graphene-based biomaterials are affected by crucial drawbacks like cytotoxicity, which could limit their applications (Citation2). Researchers have recognized that if one wants to eliminate toxicity and side effects; functionalities modification mechanisms or methods should be developed to fabricate GBCs updated to alter their chemical reactions mostly through biometric environmental friendly and bioinspired methods (Citation3).
In this review, we firstly summarize the graphene synthesis methods and the preparation processes of polymer matrix composites reinforced with graphene or its derivatives (GBCs). This is followed by a description of the main properties of graphene and their contribution to the final GBC. The current developments in GBCs and their applications in biomedical sciences such as medical sensors, tissue engineering, anticancer therapy, and drug delivery systems are all discussed. In fact, the study aims to highlight the relationship between graphene properties and its involvement in biomedical fields as nanocomposite materials.
Discovery of graphene-based composites
Nowadays, polymer composites are globally used in our industries, most specifically carbon fiber reinforced polymers, which are used in automotive and aerospace industry. In light of this, most of the material manufacturing companies are looking for improving this composite material to be more competitive by introducing fillers. In particular, the discovery of graphene in 2004, brought much attention within manufacturing industries, academia, and research to develop new composites (Citation4). Graphene is theoretically the best carbon reinforcement in polymer composites because of its one-thick-atom carbon structure (2D material as shown in ), showing Young's modulus of about 1 TPa, intrinsic strength of about 130 GPa and surface area (single graphene sheet) estimated theoretical of 2630 m2/g (Citation5, Citation6).
In 1947, Wallace formulated the early theory related to graphene and defined graphene as the graphite building block. Subsequently, Geim and Novoselov succeeded in isolating a single graphene sheet in 2004, which was a great discovery recompensed by a Nobel Prize in physics in 2010. From this, they identified individual graphene layers and other two-dimensional crystals (Citation7). Graphene is a two-dimensional, atomically thick sheet comprised of sp2 carbon atoms organized in a crystal lattice. It is the fundamental structural unit for allotropic carbon, such as graphite, fullerenes, and carbon nanotubes. For example, graphite (3-D carbon allotropic) is composed of graphene sheets layered on top and distanced by 3.37 Å. The graphene sheets are wrapped to form fullerenes, which are dimensionless carbon allotropes (Citation8). Moreover, the 1-D carbon nanotubes can be made by rolling the graphene sheets. In contrast to carbon nanotubes (CNT), these two-dimensional carbon films can reduce host membranes’ gas permeability and derive at a relatively lower price from natural graphite. Its mechanical properties in-plan are also similar to the CNT (Young's modulus ∼1 TPa) (Citation9). The possible high aspect ratio of individual graphene sheets indicates that, if effectively exfoliated in a polymer matrix, graphene can significantly improve mechanical and gas barrier features at tiny integration. Further, its stress effectiveness transfer to graphene composite is well known and how its width, thickness, defect application or attentiveness, and chemical ramification will affect the mechanical characteristics of bulk graphene (Citation10).
Structure of graphene
Graphene is a carbon allotropic. It is described as one layer of carbon sheet with a hexagonal structural matrix with hybrid carbon atoms of the sp2 tightened up into a 2D wave grid-like structure, as shown in , thereby offering a wide area on both sides of the linear axis. Graphene placed on the unmodified base band plane has free, hydrophobic electrons on the substrate. The hydrophobicity of the plot is, however, hugely reliant on the thickness, and single-layer graphene is much more hydrophilic than the thicker one (Citation11). The perfect graph is a monolayer, covalent-bonded carbon atom. Graphene is the slimmest existing element that is capable of thermal conductivity at a high rate and has properties such as quantum hall effect, intrinsic strength, and high Young's modulus. A single graphene sheet without a gap possess properties of high electronic transportation, perfect optical transmission, barrier, and flame-retardant. It shows an ambitious electric field effect with gate voltage. A single sheet of graphene consumes 2.3% of radiation, and the material's shattering intensity is 42 N/m, according to a model of non-interrelating massless Dirac fermions. Other carbon allotropes have graphene as their base structure (Citation12). TEM analysis of graphene nanoparticles revealed that Fe3O4 and Mn3O4 could be conveniently adjusted to change their particle diameter and coating densities by using different response times, as shown in (Citation13).
Two-dimensional nanomaterial (Graphene) is composed of one to ten sp2-hybridized carbon atom layers that are combined in a six-membered ring pattern. It comprises various inimitable properties, including high Young's modulus (1 TPa), strength to bear fracture (130 GPa), mobility of charge carriers (about 200,000 cm2/Vs), chemical stability, particular surface area, and thermal conductivity capability of about 5000 W/m K. By synthesizing and modifying graphene with different processes and adding it to other materials, various GBCs can be made (Citation12, Citation14). For example, Qian and Wang developed graphene/Fe3O4 composites using a solvothermal reaction ( and ) (Citation13, Citation15). These products have been developed to satisfy the growing need for high-pitched performance materials for decontamination to prevent the release of waste from factories, which can be toxic to the climate, the marine animals, and all living creatures (Citation16). In general, numerous approaches to synthesizing graphene are appropriate with the basic techniques, such as mechanical exfoliation from graphite, epitaxial growth, chemical reduction process, chemical exfoliation, and chemical vapor deposition (CVD) (Citation12, Citation15).
Figure 2. Hybrid graphene/Fe3O4 composites made by solvothermal reaction at 180°C. (A,B,C) TEM analysis of hybrid graphene/Fe3O4 composites made by solvothermal reaction at 4, 8, and 16 h, respectively; (D) HRTEM vision of boxed region of (C); (E, F) the subsequent SAED pattern and conventional EDS pattern of the hybrid composite graphene/Fe3O4. Reprinted from (Citation13) © 1999, the Royal Society of Chemistry.
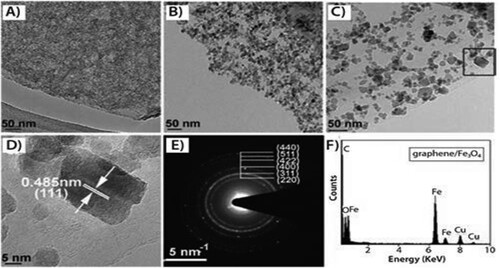
Figure 3. N-doped TiO2/graphene nano composite prepared at 180°C at varying reaction times of 7 h, 14 h, 21 h, respectively; (A, B, C), HRTEM micrograph (D), SAED pattern (E); and typical EDX pattern of N-doped TiO2 (F). Reprinted from (Citation15) © 1999, the Royal Society of Chemistry.
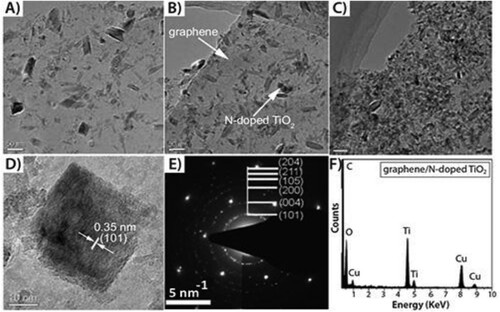
Graphene has unusual chemical features, mainly because of its sp2-linked hybridity and final surface incarnation. The graphite surface may adsorb and desorb numerous molecules and atoms such as NO2, NH3, and K, as well as OH. These connected adsorbates can be donors or recipients and lead to fluctuations in the concentration of the carrier, making graphene extremely conductive (Citation17).
The graphene features which make it perfect for sensing include: (i) it is a two-dimensional material and its entire volume is consequently exposed to adsorbed surfaces; (ii) it is highly conductive; and (iii) it has several crystal deficiencies which lead to reduced intrinsic noise (Citation17). Up to this point, most manufacturing industries claim to be producing graphene material of high quality, yet the material quite differs from one industry to another. For example, commercially available graphene differs in firmness depending on the company producing it. After characterization, commercial graphene monolayers are differentiated from one another by parameters of flake width, thickness, defect, and chemical ramification (Citation18).
Methods of synthesis of graphene and its derivatives
Nowadays, many techniques are used to synthesize graphene and they are divided into two main approaches: (i) bottom-up, in which graphene is synthesized by deposing carbon atoms next to each other on a substrate (epitaxial growth, pyrolysis, and chemical vapor deposition) and (ii) top-down, in which graphene is prepared from a graphite by extracting thin layers (mechanical exfoliation, chemical exfoliation, and chemical synthesis) (Citation19, Citation20). Below are mentioned the main techniques of graphene synthesis () (Citation20).
Figure 4. Different techniques of graphene producing. (a) Epitaxial growth; (b) chemical exfoliation; (c) chemical vapor deposition; (d) mechanical exfoliation; and (e) laser-assisted. Adapted from (Citation20) © 2012, Elsevier.
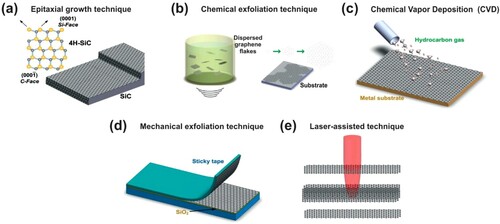
Epitaxial growth technique
The epitaxial development of silicon carbide, thus causing its degradation in the form of graphene sheets, is deemed a popular technique for graphene deposition on a substratum ((a)). Consequently, the silicon with highly pure defect-free graph sheets is desorbed off the surface. This procedure offers some benefits for the finalized component, from the metal substratum to the dielectric substratum (Citation21). The development of graphene material is entirely unclean without transformation or movement, regulated activation, and product development, which can also be accomplished by picking the correct substratum position. The silicon carbide should be tracked under high temperatures, and once non-carbon atoms have evaporated, it should be integrated. The silicon carbide would then be rearranged (Citation20, Citation22). shows a high-temperature production process of graphene by epitaxial growth. The silicon carbide may also be examined at elevated temperatures and rearranged following the evaporation of a non-carbon atom (Citation23).
Figure 5. Schematic diagram showing expatial growth of graphene. Adapted from open access publication (Citation23) © 2018, MDPI.
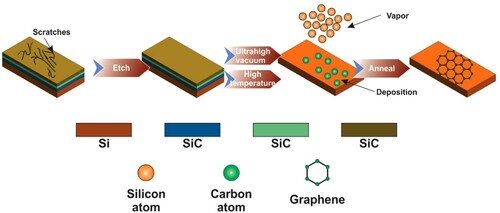
Chemical exfoliation
Chemical exfoliation is a versatile procedure to produce reduced GO (rGO) from GO, which usually supports the formulation between temperatures of 85–100 per day (moisture). GO is a disinfected graphite form that has many oxygen-containing functions and is generally metabolized in deionized water by using ultrasound approaches (see (b)). For the experimental observation stage, a transition in color from light yellow to dark suggests high content of the functional groups that are oxidized, normally identifies the transfer between GO and rGO (Citation24). Several reducing agents, such as thiourea organosulfur compound, alkaline metal borohydrides, hydrazine hydrate, hydrohalic acid solvent, and alkaline metal have been utilized to reduce GO into rGo (Citation25).
Chemical vapor deposition
One of the most widely used techniques for the formation of one-layer graphene is the CVD technique ((c)). It produces a comparatively high quality of bulk graphene. It is an uncomplicated chemical reaction that employs a carbon source at high temperatures. The decomposition results in the deposition of carbon atoms at the surface, forming the graphene layer. Moreover, chemical reaction and homogenous gas-phase reaction are the main processes in graphene deposition (Citation26).
Mechanical exfoliation
The easiest and cheapest way to manufacture graphene or few-layer graphene (FLG) from the graphite is by mechanical exfoliation ((d)), and it is known to be one of the best approaches to produce massive graphite at affordable prices. Some researchers have prepared FLG from two sources: pencil lead and graphite disc, and discovered that graphite discs provide higher outputs, better looks, and are much more homogeneous than graphite lead (Citation27).
Laser-assisted
The laser-assisted technique was recently developed to efficiently produce rGO films ((e)). Its preparation is well ordered for the in situ and non-thermal removal of solvent-based GO by laser beam emission (at room temperature) (Citation28). In this connection, the characteristics of this method continue to be close to those of traditional grids and physics. Moreover, sheet resistance compared with other ∼700 Ω/sq synthesis processes is significantly lower (Citation29).
Graphene surface modification
Graphene material functioning is used to address structural drawbacks, including poor dispersibility, chemical inertness, and a null bandgap. This categorizes change of techniques into two classes, depending on their form of bonding: covalent and noncovalent approaches. The most commonly studied form of functionality is covalently functioning graphene materials. Graphene materials can be categorized through covalence on substrates depending on the kind of reaction, including cycloaddition reactions, monoatomic reactions (oxidation, halogenation, hydrogenation), and radical reactions (Citation30). Additionally, graphene is covalently bound to polymers, and it is the most commonly used method. Graphene is accompanied by changes in electronic conductivity owing to gas molecules attached to the surface of graphene and operating as donors or receivers (Citation30).
Oxidation
Acid graphite treatments with Hummer's process can conveniently be used to produce oxidized graphene (GO). Due to the rugged environment used in this procedure, the consistency of the graphene sheet on the substratum is critical. Graphene oxide (GO) is a derivativative of oxidized graphite. Four major processes are in use, like Staudenmaier, Hofmann, Brodie, and Hummer's. Solid oxidants in acidic environments are used in these processes (Citation31). By using oxidants at 60°C, epitaxial graphene was oxidized without having been exfoliated from its silicon carbide (SiC) substrate (Citation32, Citation33). Moreover, the research suggested a mild drop-casting technique with GO with a horizontal organization and a band distance equal to 0.4 eV, despite the detrimental requirements in Hummer's process (Citation32).
Hydrogenation
As hydrogen proves to be the smallest atom that can easily be bound to the carbon compound, the initial case of covalent functionalization is hydrogenation. After the covalent bonding of hydrogen atom with carbon compound, the hydrogen changes its hybridization properties from sp2 to sp3. The zero-gapped graphene is then converted to hydrogenated graphene (G) with a 3.5 eV bandgap. Graphene materials may be hydrogenated by using plasma or through methods dependent on liquids, which provide a non-conductor or semiconductor 2D film (Citation34). In the field of modular electronics, this property has gained much attraction. Hydrogenation has been partially achieved for perhaps the first time with aerated graphene plasma processed for 2 h, and the graphene properties could quickly be restored after welding at 450°C for 24 h (Citation35). In the latest research, an insulator HyG was achieved by producing electric arcs between two aluminum electrodes using an ion source pulsed arc clusters (25 KV, 15 W, 160 Hz). The findings revealed that the measured electric resistance of HyG could be reduced progressively by increasing the irradiation time with concentrated electron beams from 200 to 2 KΩ. The analysis showed that disconnecting the HyG isolator with resolutions of 18 nm, which can be useful in producing transparent and versatile electronics and wearable electronic systems, isolation of carbon and hydrogen bands (induced by electron beams) patterns of semiconductive graphene (Citation36).
Radical reactions
Additional reactions on the surface of the graphene structure will occur in aryl radicals formed by reducing diazonium salts. The substratum of graphene gives electrons to aryl diazonium cations, while by releasing nitrogen gas, an aryl radical is obtained, which attacks graphene aromatic rings. This adjustment technique achieves a high degree of functionality, and under particular circumstances, a balance is reached (Citation36).
Preparation of graphene-based composites
Regarding outstanding properties of graphene, using this material to reinforce polymers allow to have a huge number of combination of composites for various applications. Also, by adjusting the loading of fillers the target property could be controlled (Citation37). Graphene-based composite could be classified according to their shape: fibers, yarns or ribbons (one-dimensional), films and papers (two-dimensional), other composites (three-dimensional). Also, according to the graphene loading GBC is divided into two categories: (i) composites with graphene as the minor component, which represent most composites and (ii) composites with graphene as the major component (e.g. 3D-printed graphene scaffolds) (Citation38).
In a recent review paper, Adhikari et al. (Citation39) have provided relevant information about the different method of preparation of GBC and the principals polymers that were used as matrix with graphene to form GBC such as Polyaniline (PANI), Polypyrrole (PPy), Chitosan, Polystyrene (PS), Poly 3, 4-ethyldioxythiophene (PEDOT), Polyurethane (PU), Polyvinylidene fluoride (PVDF), Epoxy (EP), Polylactic acid (PLA) Polytetrafluoroethylene (PTFE), and Polyvinyl alcohol (PVA) (Citation39). On the other hand, graphene is used as reinforcement or filler to boost the target property. Even though the GO and rGO possess relatively low physical properties compared to perfect graphene, this two derivatives of graphene represent almost majority of the fillers to prepare GBC. This is due to their low cost and high yield production combined with a good dispersion within the polymer matrix (functional groups can alter the van der Waals interactions with the matrix) (Citation4, Citation40).
The ideal GBC must meet certain requirements (i) have good dispersion of graphene filler within the matrix, (ii) contain continuous graphene sheets with a large area, (ii) have good interface binding between the graphene and matrix, and (iv) for some application the graphene sheets should be connected (Citation39, Citation40). Several methods have been developed to prepare polymer matrix composites reinforced with graphene and its derivatives. The mainly important techniques utilized are:
Intercalation of the polymer involves the dispersion fillers into the polymer matrix by using solvent-like acetone or water. Melt blending, in which polymer is mixed mechanically with graphene at high temperatures, with intercalation or exfoliation of the polymer molecules to form the composite. It is versatile and commonly used by the industry to prepare polymers (especially for thermoplastic polymers). In situ polymerization, in which the fillers are dispersed with monomers or pre-polymers and the polymerization leads to have the composites. The sol–gel method is a bottom-up approach to prepare GBC when gel is formed between phases leading to the dispersion of fillers. This method is less adopted, may be due to the low purity of the final GBC that requires a post-treatment for the purification (Citation39, Citation41).
Beside these techniques, other emerging methods could be highlighted, such as additive manufacturing fabrication, in which graphene-based polymeric nanocomposites are 3D printing. 3D geometry of the final part or device is built by successive layers deposed horizontally (2D) by a 3D printer (Citation42). The layer-by-layer approach (LBL) also is an emerging technique to prepare multilayers of GBC (thin films). It consists of alternating polymer-graphene or all graphene layers. The LBL has been widely used for self-assembly operations (Citation43, Citation44). Assembly consists of using electrostatic interactions for deposing alternating layer of opposite charge of graphene and polymer (Citation45, Citation46). The resultant composite material is to manufacture well-matched parts (Citation47). For instance, Vlassiouk et al. (Citation48) were able to prepare an ideal composite architecture (a film of CVD graphene-PMMA). The graphene layers were perfectly oriented without rolling or crumpling. In another study, Lee et al. have developed a thin film supercapacitor made of multilayers GBC (GO-polyaniline) LBL assembly. The resulting film was controlled in terms of thickness, electronic conductivity, flexibility, and chemical stability (Citation44).
The developed GBCs have three main advantages: (i) just small amount of graphene filler can provide the required reinforcement; (ii) a wide variety of properties could be improved (optical, magnetic, electrical, thermal, chemical, and mechanical properties); and (iii) these composites are even lighter compared to classic composites (Citation12, Citation39, Citation49).
Properties of graphene-based composites
This section discusses major characteristics of graphene-based materials from several aspects, including mechanical, thermal, electrical, impermeability, and biological characteristics.
Mechanical properties
Pristine graphene is made up of two-dimensional planar sheets of sp2 atoms with almost unbreakable covalent bonds organized hexagonally. In addition to being nonlinear, graphene has a highly slippery elastic behavior. Damage occurs when its inherent strength is determined by critical stress (about 130 GPa) (Citation14, Citation50). Graphene is considered to be an extremely strong material owing to its structural features leading to high mechanical properties. In addition, the ability to bend is another graphene characteristic that makes it suitable for use in electronics and efficient sensors. For example, GO paper has average break strengths and elastic modulus of about 80 MPa and 32 GPa, respectively (Citation51).
From this, it could be expected that a small loading of graphene in a polymer matrix allows the production of GBC with outstanding mechanical properties (significant increases in tensile strength and elastic modulus). The improvement highly depends on the graphene derivative (intrinsic properties of the graphene), the loading value, the polymer nature, and finally the process (Citation12, Citation41, Citation49). Many studies have found that there is a critical volume of graphene filler when the mechanical property of the GBC is at its peak. In fact, the mechanical properties increase with increasing the graphene loading in the polymer until reaching the critical value. Above this value, a decrease in the mechanical properties is observed due to the aggregation of the filler (stacked together) (Citation41, Citation52). To highlight the effect of the graphene derivative in composites, Bao et al. (Citation53) prepared two composites consisting of a PVA matrix reinforced with 0.8 wt% GO for the first one (GO/PVA) and 0.8 wt% rGO for the second (rGO/PVA). The results have indicated that rGO/PVA exhibited the highest increase in mechanical properties compared to GO/PVA. Tensile strength and elastic modulus improvement were 66% and 70% for rGO/PVA and 54% and 52% for GO/PVA, respectively. This was attributed to the high mechanical strength of rGO (Citation53). Nevertheless, the GO is also a good candidate to reinforce polymers to: (i) good mechanical properties; (ii) load transfer and interfacial interaction due to the presence of functional groups; and (iii) showing good dispersion on the polymer due to functional groups (Citation41). On the other hand, the process also influences the final properties of the composite. In fact, 1 wt% rGO/PMMA composites prepared by in situ polymerization (Citation54) and solution mixing process (Citation55) have shown different increases in tensile strength, 60.7% and 20, respectively. Globally, it has been shown that the GBC could find application in materials requiring high mechanical properties combined with light weight and flexibility (Citation39, Citation41).
Thermal properties
Due to the relatively low density of the non-doped graphene, thermal conductivity is dominated by phonon transport, and the contribution of electronic conductivity is negligible (Citation56). Graphene offers exceptional thermal performance (long-range graphene pattern). A high value (3000–5300 W/m K) of the thermal conductivity of graphene was reported by applying theoretical as well as experimental approaches. This value was considerably superior to that of diamond and graphite; but comparable to that of CNT. The sp2 hybridization of strong C–C connections may be verified to provide efficient heat conduction using gate vibration or phonic models (Citation57).
Therefore, it is expected that adding a small amount of graphene to polymers will allow the fabrication of a composite with high thermal properties (Citation39, Citation41). For this reason, many works are devoted to study the thermal stability and thermal conductivity of GBC such as: 25 vol% rGO-Epoxy (Citation58), 0.3 wt% rGO-Epoxy (Citation59), 2 wt% rGO-PS (Citation60), 0.7 wt% GO-PVA (Citation61), and 3 wt% rGO-PLA (Citation62). In fact, it has been shown that an increase of about 3000% in thermal conductivity can be achieved by using a 25 vol% loading of rGO as a filler for epoxy. The composites prepared by Yu et al. (Citation58) showed a thermal conductivity of k = 6.44 W/m K, which was reported to be superior to other carbon material fillers (Citation58). However, it is also been reported that the filler-matrix interface could limit the thermal conductivity of the composite. To overcome this obstacle, Hu et al. (Citation63) functionalized GO in order to improve the interfacial bonding of the composite. With 0.5 vol% of GO, the thermal conductivity of the epoxy was increased by 800%. Also, the thermal conductivity of the composite was 30% higher than that of composites filled with CNT (Citation63). In other work, Bui et al. (Citation64) have indicated that the 2D geometry of the graphene gives more thermal conductivity efficiency to GBC compared to the 1D shape of CNT.
On the other hand, the thermal stability and flame retardancy of polymer matrix composites could also be improved through graphene and its derivatives. This is by considering the high thermal stability of graphene as a barrier hindering the transfer of combustion gases. Compared to a neat polymer matrix, adding a small amount of graphene can significantly improve the thermal stability of GBC (Citation49). For example, Guo et al. (Citation59) have investigated the flammability of a rGO-epoxy composite. The results showed that the total heat release rate decreased when increasing the volume content of rGO in the composite (decreased for 43.9% at 5 wt%) (Citation59). These above-mentioned properties (conductivity and stability) make the GBC a promising material for thermal applications such as electronic components (Citation49).
Electrical conductivity
The electrical capabilities of graphene have attracted greater attention as compared with its other outstanding characteristics. Graphene may be categorized as an intrinsic semiconductor, having a distinctive Fermi energy level configuration with a linear Dirac-like spectrum (Citation65). The double-layer graphene band overlap has a 1.6 meV, extremely tiny and insignificant, parabola spectrum. It may thus be seen as a metal-like substance with a single sort of exciton. Concerning graphene belt constructions with more than three layers, they should be indicated with several load carriers that straddle the line and valence band (Citation66, Citation67).
Several studies have been conducted to improve the electrical properties of polymers by using graphene as a conductive filler. For this, various GBC were investigated, such as 0.19 vol% rGO-PS (Citation68), 2 wt% rGO-PU (Citation69), 1 vol% rGO-HDPE (Citation70), and 3 ≈5 wt% rGO-PLA (Citation62), and 1 vol% rGO-PS (Citation71). For instance, it was reported that a GBC (rGO-PS) with only 1 vol% of rGO has 10 orders of magnitude in electrical conductivity compared to neat PS (Citation71).
In a review paper, Du et al. (Citation41) have concluded that the perfect electrically conductive GBC should has a good conductive network of filler, which provides higher conductivity at a lower graphene filler loading (i.e. lower point at which an insulator-to-conductor transition occurs). In fact, the low volume fraction of graphene in the GBC is a key factor in preserving the processability of the composite as well as decreasing the cost of the final materials. This is because it was observed that electrical properties of graphene/polymer composites are mainly influenced by dispersion state, aspect ratio, intrinsic electrical conductivity, contact resistance, and quality of the interconnected 3D network. Also, it has been shown that GBC is more efficient in terms of electrical conductivity compared to CNT-based composite; this is thanks to some graphene properties such as (i) the easiest to aggregate due to a larger surface area; (ii) lower aspect ratio due to wrinkle and crimp behavior; and (ii) multitudes of contact possibilities due to the 2D shape. These properties make the GBC an attractive candidate in many applications when electric conductivity is important (Citation41).
Impermeability
Graphene is impermeable to various gases and chemicals due to the following reasons: (i) strong resistance; (ii) high electron density; (iii) densely packed carbon atoms; and (iv) tight overlaying of sheets. Graphene is a highly waterproof substance (Citation72). In 2008, it was claimed that the impermeability of graphene-based materials could be used in novel applications such as selective gas permeation, ultra-thin composite corrosion coating, wet electron microscopy confinement, nanoporous bio-scale, and water molecular layers of retention. The selected synthesis process (which influences the flaws) for graphene is important for permeability (Citation72). In another study, Wang et al. (Citation73) indicated an obvious improvement in the impermeability of PVA to water when adding graphene. In fact, by using 1 wt% of rGO to prepare an rGO/PVA composite, the water absorption ratio of PVA decreased from 105.2% (pure PVA) to 48.8% (rGO/PVA composites). Also, GBC has a good impermeability (barrier properties) to some gases, such as O2 (Citation74), N2, (Citation75), and He (Citation76). These gas and water barrier properties are very important in packaging materials as well as in biomedical devices (Citation41, Citation77).
Biological characteristics
The majority of GO and its derivatives are cytocompatible both in vitro and in vivo. 2D materials, on the other hand, have physicochemical features that affect cellular activity. Surface concentration and aggregation states are two aspects of graphene surface functioning. When administered across cell membranes, graphene's sharp edges make it potentially hazardous to cells. In some cases, its aggregation form might be cytotoxic. Cytotoxicity, inflammation, and even genotoxicity are all side effects of nanoscale graphene (due to facing less steric hindrance). Moreover, functionalized graphene (GO, FGO, and rGO) is readily absorbed by cells (particularly at nanoscales) and causes more irregular cell membrane disruption. GO and its derivatives have been shown to have antibacterial characteristics and are employed in tissue engineering applications. Membrane tension, oxidative stress, entrapment, basal plane, and photo-thermal are the mechanisms underlying GO-induced antibacterial effects were widely investigated (Citation78). The membrane stress response system can be triggered by GO because it contains sharp edges that can disrupt the cell membrane. In the presence of bacteria, the structure of GO allows for electron abstraction within the membrane, compromising membrane integrity and ultimately killing bacteria (particularly Pseudomonas aeruginosa and Staphylococcus aureus). Because GO and rGO include functionalized groups, they can change the partial pressure of intracellular oxygen, causing oxidative damage and bacteria death, particularly in Escherichia coli (E. coli). When combined with laser light, GO has synergistic advantages; therefore, it is employed in photo-thermal therapy to boost antibacterial activity. Since sp2 carbons are required for radical adducts formation and electron transfer between them, the antioxidant activity of GO-based materials is especially intriguing (Citation79, Citation80). Because of this property, these biomaterials can effectively scavenge free radicals and protect cells from oxidative stress. Non-biodegradable graphene (except FGO) is associated with major concerns concerning toxicity, immunological reactions, and environmental toxicity. GO is sensitive to oxidative degradation by hydrogen peroxide and horseradish peroxidase in the presence of oxygen. Because the disintegration rate of biomaterials (e.g. scaffolds) must match that of tissues and organs, many attempts have been made to speed up GBC biodegradation (Citation39, Citation79, Citation81). For instance, Wan and Chen (Citation82) have revealed the importance of functional groups on the GO surface in promoting bioactivity for polymers. Hence, the formation of calcium phosphate on the surface of the GBC was attributed to the good bioactivity of GO.
Recent advances in applications of graphene-based composites
Electrical applications
Storage of energy and solar cells
Graphene is thin in nature; it occupies a large surface area to volume ratio. This feature makes graphene very attractive for energy storage applications, when it is used in manufacturing batteries and supercapacitors. Graphene can provide several advantages to energy conversion and storage devices, such as high capacity, fast charging, lightweight, changeable, and high-temperature range (Citation83). Graphene-based material has hopeful used up to time energy associated sites. Some of the recent advances in our today's life are improving the energy capacity and the charge rates to rechargeable batteries; updated graphene creates supercapacitors for energy storing (Citation40). Furthermore, graphene is highly regarded in advanced solar panel cells. In fact, researchers have recognized the very association of graphene's chemical nature or material that acts as a host to either half of the positive and the negative electrode, which are used in energy generation at a low rate. For instance, Sookhakian et al. (Citation84) have developed a GBC to fabricate hybrid electrodes for high performance energy storage. The nanocomposite (Prussian blue-N-graphene composites) has been recognized as high performance hybrid battery-supercapacitor electrode due to the presence of graphene (Citation84).
Sensors
Functioning graphene material has a key promising word in exclusion of either biological or chemical functions. A group of researchers has noticed that the emerging 2D structures of GO, with its waterproof nature can sense all devices with unknown speed, for instance, the Ultrafast graphene sensor, which monitors breath speed when speaking or communicating with colleagues (Citation85). Researchers have noticed that chemical vapor alters graphene transistor noise spectra, hence making the graphene structure distinguish many devices with graphene-based components-pristine graphene. It is also indulged in using 3D structure material which has high electric affinity or conductivity; these gases have high sensing nature (Citation86).
Transistors and memory
The most known use of graphene and its oxides are in electronics, detectors, and thermal administration. The first graphene with both top and bottom gate structure called field effects transistors (FETs) have been shown up to time. Therefore, for any transistor to be used for both analog and digital communication, the level of electronic low-frequency noise has to be reduced to a certain favorable point (Citation87). Transistor based on graphene is considered the replacement of silicon because electrons move faster in graphene than in silicon. Graphene, at this point, shows the capability to enhance terahertz computing. Graphene has the high potential power to un-align and takes overused silicon material, and graphene has pristine graphene that acts as a barrier in transistors made of this latest. Practically, graphene has been reviewed as the most promising factor material in memory, unlike current memory materials, because of its intrinsic nature (Citation88).
Flexible, stretchable, and fordable electronics
The presence of flexible, stretchable, and fordable electronics is the key feature of advances brought by graphene and its components worldwide. In fact, the flexibility of GBC shows great potential application of flexible, portable, and wearable devices; the resulting GBC properties provide a bridge between nanoscale graphene real macro-scale applications (Citation89). Researchers have come to recognition that graphene can be introduced as filler into either flexible or stretchable products. These composites are used as substrates in conducive conditions for manufacturing of FETs and logic circuits, which is known as flexible graphene and builds a top-gate structure, whereby ion-gel is sandwiched within graphene channel, by use of conventional printing method, acts as a gate dielectric (Citation90). As another example, the graphene-based freestanding paper-like materials find many applications in electrical engineering (electrochemical actuators, electronic, optoelectronic, and flexible supercapacitors) and biomedical field (miniaturized lab devices), the advantage is to have excellent flexibility without compromising the sensitivity (Citation89, Citation91). It was shown that the highly flexible graphene-based film has also a potential application in energy harvesting. In fact, Jiang et al. (Citation92) have developed a flexible triboelectric nanogenerator based on GBC film which can convert harvest writing energy, promoting a potential application in writing or tactile sensing for pad (Citation92).
The ion-gel dielectric gate has crucial characteristics useful in flexible devices such as low-temperature process act, which are present inside substrate made of plastic and high capacitances for use in reduced voltage operations and excellent mechanical features. Low temperature is vital when producing substrates outputs like a rubber balloon. On the other hand, stretchable or foldable displays items made of plastic substrates face serious degradation caused by moisture. For this reason, adding barrier layers (Al2O3 and SiO2) is highly recommended. However, under mechanical deformations, these barrier layers showed fracture. (Citation93). In this context, by introducing a double-layer graphene between alumina and a polymeric substrate, Won et al. (Citation93) have successfully prepared a stretchable and transparent moisture barrier. The crack density of the alumina layer was reduced to sliding between the graphene layers, which enhanced stretchability of the composite materials.
Mechanical applications
Graphene is world-widely recognized as the strongest material, as it impacts strength to other materials. Reinforcing materials like plastic or metals with graphene makes them much stronger for different uses. The composite material reinforced by graphene is known to have immense use in telephone, building materials, mobile devices, among other applications (Citation94).
Thermal applications
Graphene possesses high heat conductibility features which boost its popularity up to time. Graphene's popular features enable its functionality in biomedical applications due to its capability of breaking in the penetration rate. Since graphene is strong and light, most companies prefer it to other products since it is mostly used in dispassion solutions like heat sinks and dissipation films (Citation95). This product is in turn used in micro-electric or immense applications like thermal foils made for mobile devices. For example, Huawei's latest phone has greatly emulated graphene-associated thermal films (Citation96).
Graphene-based composites as biomaterials
GBC materials have played a significant role in the biomedical field (). Acrylic-based nanoconjuctant systems have piqued attention as a platform for cancer theranostics efficiency in polymer- and protein-based nanotechnologies. Zhang et al. (Citation97) have investigated a wide range of pH-sensitive polymers, including poly(N,N-dialkyl aminoethyl methacrylate), poly(acrylic acid), and poly(methacrylic acid), as well as drug delivery systems, stimuli-responsive hydrogels, and even dental restorative substances. In the past 30 years, the resin-based filler composites technique has been modified for clinical research (Citation77). In dentistry, for example, amalgams compounds based on graphene nanocomposites had higher aesthetic features, increased safety guard matter, thereby providing relatively satisfying clinical outcomes. Enhancements in their formulation, such as new green/organic monomers and fillers techniques, have progressively enhanced the characteristics and functions of these materials over time (Citation98). The graphene use in medical applications is wide. Current investigations majorly focus on utilizing the characteristics of graphene and its 2D material for novel medical instruments or devices that could be used to improve the healthcare community. This includes testing kits and smart implants such as medical sensors and 3D scaffolds (Citation99).
Finally, from , the GBC applications for medical devices demonstrate a significant potential to improve human health. The use of graphene not only keeps the end product light in weight but also makes the processing more accessible for various multipurpose medical applications (Citation47). The outstanding properties of graphene, cited above, bring advantages for the GBC in biomedical applications such as bio-imaging, medical sensors, drug delivery, tissue engineering, and anticancer therapy (). More details will be presented in the following paragraphs (Citation16).
Figure 7. Schematic diagram showing graphene properties and its applications in biomedical. Adapted from open access publication (Citation16) © 2020, Taylor & Francis.
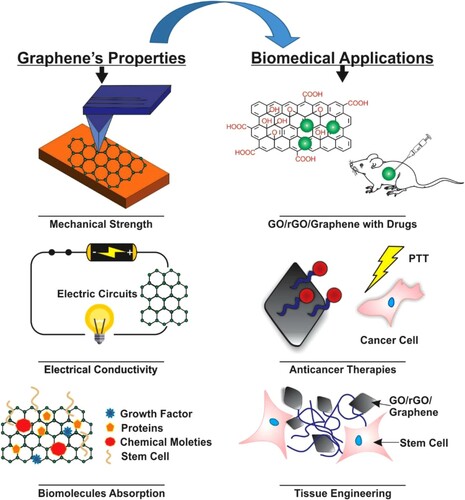
Biomedical applications
Bio-imaging
Bio-imaging is a technique that allows observing and studying biological entities in cellular and subcellular levels, which plays crucial roles in regenerative medicine. GBCs in general showed a potential in various imaging techniques applications, such as ultrasonography (US), photoacoustic imaging (PAI), magnetic resonance imaging (MRI), computed tomography (CT), fluorescence/confocal imaging, surface-enhanced Raman scattering (SERS), coherent anti-Stokes Raman scattering imaging (CARS), and electron paramagnetic resonance imaging (EPRI). Using bio-imaging help to monitor processes in living cells, tissues, or the whole body. Thus, abnormal processes, such as necrosis and cancer development, could be tracked (Citation77, Citation100).
In this regard, Sun et al. (Citation101) have found that the photoluminescence of nGO–PEG could be exploited for cell imaging in the near-infrared (NIR). In fact, GO and nGO showed fluorescence broadly from the visible to the IR range. Subsequently, Ma et al. (Citation102) have revealed for the first time the possibility of in vivo magnetic resonance (MR) imaging of tumor-bearing mice by using a GBC contrast agent (Citation102). On the other hand, labeled nGO–PEG was found to be adapted for in vivo positron emission tomography (PET) imaging, which facilitates active tumor targeting (Citation103). Likewise, besides the nano GO (nGO), graphene quantum dots (GQD) with ultra-small sizes show high photostability combined with cytotoxicity, indicating a great potential in bio-imaging (Citation100, Citation103).
When taking into account that each imaging method has its own advantages and limitations, using several methods at the same time (Multimodal Imaging) could provide better diagnostics (Citation100, Citation103). In order to make the bio-imaging by GBC more versatile, Yang et al. (Citation104) have combined rGo with iron oxide nanoparticles (IONP). The resulting GBC (RGO-IONP-PEG) demonstrated strong magnetic properties, high NIR absorbance, and fluorescence labeling, allowing for triple modal in vivo tumor imaging. Furthermore, the authors demonstrate that GBC could be used for real-time monitoring of photo-thermal therapeutic responses of tumors. In fact, it was possible to detect the complete ablation of the tumors after treatment (Citation104). In another report, Chen et al. (Citation105) presented an imaging agent that can combine fluorescence and photoacoustic dual-modality. This was achieved by combining GBC (nano rGO-PEG) with indocyamine green (ICG). Also, Yue et al. (Citation106) have reported the possibility of target tumor cells. In addition to showing good biocompatibility, multimodal imaging through GBC may have a high potential for imaging-guided cancer diagnosis and therapy (Citation100, Citation106).
To summarize, the most commonly used GBCs for bio-imaging are prepared by combining GO, nGO, or GQDs with biopolymers such as polyethylene glycol (PEG), polyethyleneimine, and polystyrene. Also, by integrating other materials such as IONP with specific properties onto GOs, a multimodal imaging platform could be obtained. Hence, GBC has the potential to provide powerful detection monitoring tools for biomedicine due to the several roles that could be played by graphene and its derivatives within GBC, such as carriers, effective contrast agents, fluorescence quenchers, or wrapping materials (Citation77, Citation100, Citation103, Citation107).
Medical sensors
Sensors made from graphene are highly sensitive, which can sense any hazard particle or atom passing by, and hence protecting the certain environment from environmental hazards (Citation37). Graphene is a very recommended material for making sensors. This is because every atom of graphene is always exposed to their surrounding areas where they sense danger ought to rise in such a situation. Chemical sensors are mostly meant to sense chemical information in an environment, such as concentration, pressure, moisture, or particle activity (Citation108). For instance, Ping et al. (Citation109) have developed a graphene-based disposable bismuth film electrode that can be used as a sensing platform for heavy metals. They have found that this new generation of electrochemical transducer is a reliable, sensitive, and stable device, which could widen the scope of application of graphene in this field (Citation109). Up to the time, graphene has been used to sense particular danger by creating micrometer-size sensors which could sense each event in a particular molecular range. By definition, sensors are devices that can detect substances, pressure, or deformation and convert their concentration into an electrical signal (Citation110, Citation111).
In the biomedical field, due to the widely proliferative of cardiovascular diseases, neurodegenerative disorders, cancer rates, and autoimmune diseases, combined with increasing personnel heath care cost, have enabled the emergence biosensors as health monitoring devices (Citation108, Citation112). This technology is capable of specifically and rapidly detect ultralow concentrations of relevant pathogens, active molecules, biomarkers, pharmaceuticals, and toxins elements in environmental and biological environment (Citation108, Citation113). Besides detecting the precedingly cited elements, the device can be used to monitor critical health parameters such as motion detection blood pressure, blood glucose electrocardiogram signal, electromyogram signal, respiration rate, pulse oxygenation, body temperature, heart rate, etc. (Citation111–113).
In a review paper, Singh et al. (Citation113) have indicated that GO-based materials could be used in biomedical as sensors for several applications such as DNA/RNA detection, Aptamer-based DNA detection, glucose detection, and cancer bio-sensing (Citation113). In another review paper, Huang et al. (Citation111) have thoroughly investigated graphene-based materials as sensors for human health monitoring. The authors give an overview of both non-invasive and invasive sensors by exploring their potential application (). The non-invasive sensors are materials that do not infiltrate and break in the tissue or skin while in operation, which include detecting vital signals, such as biophysical signals (electrophysiological measurement, kinematic detection, thermometer), biochemical signals (metabolites, electrolyte, and volatile biomarker gases), and environmental signals (gases, light, heavy metals). On the other hand, the invasive sensors are more close to the target tissues or organs within human body significantly improve the sensing accuracy. Thereby, this kind of sensors exhibits huge interest in medical applications, such as implants for digestive system, nervous system ((a, e)), cardiovascular system, and locomotor system (Citation111).
Figure 8. Potential application of GBCs as sensors for the medical field. (a) GB sensors for nervous system: schematic of graphene transistor (left) and their correspondent implant; (b) GB metabolic sensor; (c) GB bioelectrical electrode; (d) GB strain sensor; (e) GB sensor for nervous system: comparison between neural response to electrical stimulation with platinum and graphene electrode; (f) invasive sensor: The difference between tumor image captured with camera of the endoscope through metalelectronic devices (right) and transparent bioelectronic devices based on graphene (left). Adapted from open access publication (Citation111) © 2019, Frontiers.
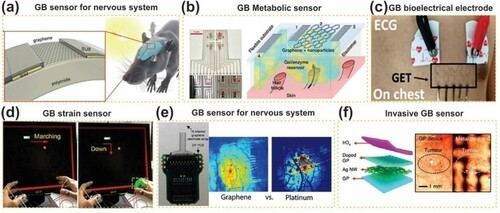
In this regards, the GBC sensor is an outstanding candidate for both structural and wearable health monitoring, this is due to: (i) high flexibility and durability of the polymer matrix. (ii) Conductive and sensitivity of graphene and its derivatives that greatly improve the electrochemical detection. (iii) The GBC-printed circuits are easily integrated into complex structures. Finally, (iv) GBC-based composites can be combined with biological receptors (Citation42, Citation111, Citation112).
Drug delivery
Frequently used for drug delivery systems, GO usually has multi-layer compounds of ∼1–3 layers, ∼1–2 nm in thickness and a particulate size ranging from a few nanometers up to hundreds of nanometers. In addition, it has various unique features that have appropriate biocompatibility and physiological solubility, notably in drug/gene loading through chemical conjugation or physisorption response (). Further, the volatile carboxy and hydroxy function groups (–COOH and –OH) on the surface of GOs are interesting for a wide range of biomedical applications. In fact, including graphene in polymers gives organic compatibility and helps reduce their hazardous effects. For instance, in doxorubicin (DOX) deposited graphene nanosheets, gelatin functions as a functional agent. Given the enormous surface area of gelatine graphene and its high P interactions, this phenomenon increases the drug loading capacity. Gelatin–graphene–DOX combination had disclosed a few years ago that the high toxicity to MCF-7 might activate the responsive nanocarrier system for the selective delivery of the drug DOX to the cytosol (Citation42).
In addition, an efficient approach was to employ the well-established poly(N-isopropylacrylamide) (PNIPAM) functionalized graphene sheets (GS). PNIPAM–GS is generally used over the course of the investigation to manufacture camptothecin (CPT) and the discharge of PNIPAM–GS–CPT in water conditions and poly(butylene succinate) (PBS) at 37°C as a poor water-soluble anticancer drug. The transition phase between hydrophilic and hydrophobic was changing at 33°C, which shows that it is lower than 37.8°C for PNIPAM homopolymer (Citation114). However, an effective CPT load of 18.5% was reached; this was attributed to the hydrophobic and p–p stacking behavior that reacted against the graphene plate and showed significantly higher activity than the CPT specimen. This demonstrated that PNIPAM–GS sheets were virtually not hazardous to the health of humans and had excellent CPT association capabilities (Citation115).
A new revolutionary GO-based nanocomposite containing chitosan (CHI) powder that was introduced to increase biocompatibility and to construct a GO–CHI–HA complex under CD44 target ligands in conjunction with hyaluronic acid (HA). Moreover, this combination can determine the exact identification of tumor cells and greatly improve their efficacy in terms of antitumor medication delivery (Citation116). The resulting GO–CHI–HA final product was then combined as an anticancer medicine (Hsp90 inhibitor) with the SNX-2112 (anticancer agent). Apart from the general release, acidification was considerably active compared to radiologic conditions in the total released quantity of SNX-2112. In addition, the low-level GO–CHI–HA did not influence red blood cell (RBC) lysis and coagulation. Moreover, GO–CHI–HA/SNX-2112 had proved its cytotoxicity towards healthy bronchial NHBE human cells in the death of A549 cells (Epithelial). In conclusion, the GO–CHI–HA nanocomposite exhibited high drug loading little adverse side effects (no severe long-term injury). In addition, various existing nanostructures that possess good biocompatibilities with GO are accessible as anticancer drug agents. For instance, lysine-treated highly porous graphene (Gr-Lys), Ginsenoside Rh2-treated GO (GO-Rh2), Gr-arg-treated arginine (Gr-ARg), Gr-Lys-recorded Rh2 (Gr-Lys-Rh2), and Gr-Arg-treated Rh2 (Gr-Arg-Rh2) were able to enhance the anticancer activities. Moreover, the functionalized graphene with Arg and Lys have low side effects compared to the non-functionalized (Citation117). Therefore, it can be concluded that using GBC for the drug delivery system could be a promising strategy for anticancer (Citation16, Citation103). Finally, these cited works well demonstrate the benefit of graphene and GBCs in the drug delivery field. However, it is worth to note that GBC drug delivery should possess some extra properties related to safety of the patient, such as easy to administer, biocompatibility, reliability, and most be non-hazardous (Citation38, Citation39, Citation118).
Tissue engineering
Using technologies in medicine, biology, and engineering has contributed to developing biomimetic tissue (constructions for organ transplantation and diagnostic or therapeutic devices). Tissue engineering is a multi-disciplinary area, which includes investigations on particular cell functions, direct cell differentiation, and modifying cell–cell interactions in tissue engineering (Citation119). Tissues differ mechanically, electrically, and physically from one another. Research into tissue engineering and regenerative medicine has been spurred by the remarkable mechanical and electrical characteristics of graphene. Engineered materials with specified properties may be developed by combining graphene with a range of bioactive ingredients (). As a gene/drug delivery vehicle, graphene's unique atomic structures provide it with a high adsorption capacity. Graphene and its derivatives offer a new option for improving the mechanical and surface characteristics of biomaterials (Citation120). Another benefit of graphene is that it has a high electrical conductivity, which is predicted to boost cardiac myocyte and neuron development and activity, while also improving the recording of cellular impulses. To produce more complicated graphene structures, 3D printing technology must be combined with adjustable surface chemical and machinable characteristics (Citation47). Solanki et al. (Citation121) have indicated that nanoparticle monolayers coated with GO (hybrid nanostructures) have a huge potential in the field of neurobiology as an ECM component (). In fact, the hybrid structures (positively charged silica nanoparticles and graphene) result in nanomaterial containing oxygen functional groups attached to the graphene basal plane, which allows nanosheets to attach readily to molecules ((b)) (Citation121).
Figure 9. Graphene hybrid nanostructures for neuro-regenerative medicine. (a) Experimental substrates and (b) enhanced growth and alignment on GO-nanoparticle structure. Reprinted from (Citation121) © 2013, Wiley.
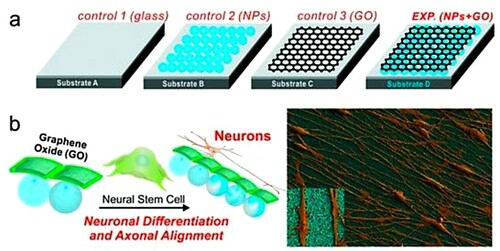
To produce multifunctional smart materials, graphene and its derivate can be combined with other materials having characteristics such as wettability or flexibility. Carbon nanotube composites can become environmentally sensitive or have shape memory or self-folding characteristics that could broaden their biological uses (Citation119). When GO was combined with a phase-changing substance, a thermos-responsive drug delivery system was developed. For photodynamic treatments, this method enhances drug loading efficiency as well as the photo-thermal effects. Merging self-folding graphene-based materials with 3D printing technology can assist create the next generation of printing techniques for tissue engineering. Because of their cytotoxic or genotoxic properties, graphene and derivatives have been widely employed in a variety of biological fields. When it comes to graphene products in biological systems, there is no gold standard. The graphene-derived simulations on cell activity in vitro should be developed (Citation65).
Anticancer therapy
Graphene may be used to load a variety of biological compounds, including polymers, proteins, carbohydrates, and anticancer drugs (). To enhance tumor cell transport, graphene derivatives are modified to avoid or increase their hydrophilicity in the biological fluid, among other things. Graphene is also employed as a cancer detection tool (Citation122). GO inhibits the development of tumor spheres in breast, ovarian, lung, pancreatic, and glioblastoma cell lines. GO is more harmful to cancer cells than normal fibroblastic cells. In cancer cells, GO has been shown to activate toll-like receptors, which can lead to autophagy and antitumor actions, according to research (Citation123). The PEG–GO conjugate was found to cause tumors in xenograft animals, and hence has a high therapeutic potential. Now, it is believed that the phototherapeutic effect of nGO subordinates is caused by oxidative stress generation and depolarization of mitochondria, caspase activation, which causes necrosis and apoptotic cell death (Citation17).
The use of graphene in anticancer treatment is still in its infancy, but there are a lot of obstacles to overcome. Understanding the mechanisms underlying the interaction of graphene to not only cancer cells, but also healthy cells is crucial to the future application of graphene. Graphene's impact on human cells must be understood in terms of its method of activity. Graphene's anticancer properties will be improved as a result of this understanding. The toxicology of graphene and its compounds in vitro may be substantially different from that in vivo (Citation123).
Antibacterial activities
The benefits of using GBC for antibacterial applications were exhibited by several researches works (Citation124–127). In a dedicated review paper, Xia et al. (Citation79) have listed the most target microbial specie by the antibacterial activities of GBC. such as Escherichia coli, Staphylococcus aureus, Pseudomonas aeruginosa, Streptococcus mutans, Porphyromonas gingivalis, Klebsiella pneumoniae, Salmonella typhimurium, and Fungus/Candida albicans (Citation79). The interaction of GBC with pathogens agents is greatly affected by the physicochemical characteristics of graphene, such as surface-related properties, shape, size, dispersibility, functionalization, and electronic structure (Citation79, Citation81, Citation127).
Recently, Fatima et al. (Citation81) have summarized the factors affecting the antimicrobial activity of graphene used in GBC: (i) shape and type of bacteria: it was found that GBC has a higher efficiency on Gram-negative type bacteria than on Gram-positive type. (ii) Size of the graphene sheet, when smaller sheets, the GBC shows excellent antimicrobial activity due to the greater defect density and interaction area, however, smaller sheets are more cytotoxic. (iii) Number of layers of graphene: it was revealed that monolayer graphene sheets have more interaction with bacteria compared to multi-layer ones. (iv) Concentration of GBCs: it was demonstrated that increasing the concentration of graphene provides more efficiency to eradicate the bacteria. (v) Dispersibility and aggregation of GBC: the dispersing graphene exhibited the highest antimicrobial activity. Finally, (vi) the functionalization of graphene with polymers: it was shown that the formation of stable graphene–polymer improves the dispersion by integrating the graphene into the polymeric matrix, hence, enhancing their antimicrobial activity (Citation81).
3D printed scaffolds
GBCs of diverse characteristics show great potential to be used in the next materials generation. Hence, efforts were made to find simple and effective methods of GBC preparation, which are based on 3D additive manufacturing ((a, c)). It has been shown that using an ink-jet printable graphene results in cheap and scalable ways of introducing GBCs into today's real-life technology (Citation119, Citation128, Citation129). For this purpose, Jakus et al. (Citation129) have developed a graphene ink, which can be 3D printed to form GBC with high graphene content (60 vol% of graphene and 40% polylactide-co-glycolide (PLG)). They have demonstrated the possibility of 3D printing GBC which is electrical conductivities, mechanically robust, and flexible at the same time ((c)). Furthermore, in vivo experiments indicate that the developed GBC has a good biocompatibility and can be manipulated in fine surgical procedures ((d)). In vitro experiments reveal that the GBC supports human mesenchymal stem cells, suggesting a possible application in nerve tissue engineering. Thus, the graphene-based bio-ink is a promising GBC to prepare scaffolds with high graphene content for tissue regenerative engineering applications (Citation129).
Figure 10. 3D graphene ink printed nerve conduits: (a) 3D graphene ink printed process; (b) SEM and optical (inset) images of graphene-PGA 3D printed; (c) uniaxial multichannel nerve guide of different size; and (d) nerve conduit of 3D graphene ink implanted in human cadaver. Reproduced with permission from (Citation129) © 2015, American Chemical Society.
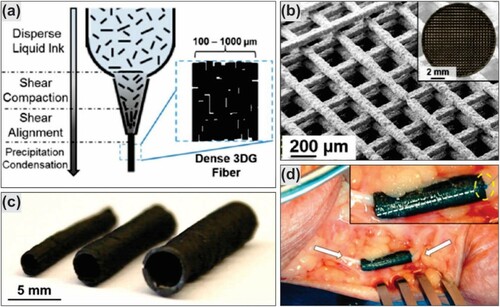
Challenges facing the GBCs
Impact of using GBCs on human health
GBCs have a wide range of applications in the biomedical area like drug delivery, antibacterial materials, and tissue engineering. Therefore, it is vital to take account of all the hazardous effects of graphene on human health before its utilization as a therapeutic agent. Its wide production can lead to huge human and environmental acquaintances. It is essential to measure the toxicity level of GBCs so that their risks to human health and the environment can be addressed (Citation79). Determination of the interactions of GBCs at molecular and cellular levels is essential to understand the toxicity effects. The alteration of GBCs in biological systems leads to future breakthroughs in biotechnological applications by addressing the hurdles in therapeutic delivery (Citation130). The physicochemical features of GBCs play a critical role in the assessment of toxicity from a toxicological point of view. For example, the toxicity of GBCs depends on dosage, surface chemistry, exposure route, and purity. The toxicity also includes the surface area, layer count, lateral size, surface chemistry, and pure GBCs (Citation131).
For every bio-functionalization, the surface chemical composition of GBCs is of paramount relevance. The GBCs surface gradually decreases with an increase in the layer number. An essential aspect is the number of layers of GBCs, which defines the particular surface and bending rigidity (Citation132). The dimension of the material, which is relevant to biological behaviors (cells intake, kidney clearing, and transpassing the blood–brain barrier), influences the specific surface area but instead determines the dimension of the material impacted by particle diameter. For estimating the toxicity effect, it is necessary to keep in mind the aforementioned properties of graphene-based nanocomposites (Citation133). Different organs might suffer when graphene-based particles are administered to the organ by blood circulation or bio-barriers. Because of their nano-size, round shape, functioning, contaminants, aggregation, charges, and physical destruction, the graphene-based nanoparticles may penetrate organs through the blood-testis barrier, blood-air barrier, blood placental barrier, and blood–brain barrier (Citation134). The toxicity of graphene-based nanocomposites to cells can be attributed to multiple cellular processes such as oxidative stress, damage to DNA, inflammatory response, autophagy, apoptosis, and necrotization play a critical part. Although graphene-based nanocomposites have projected different biomedical uses, the major challenges in their biological uses are toxicity and biosecurity (Citation135).
The toxicity of graphene-based nanocomposites is based on many characteristics such as lateral dimensions, hydrophobicity, rigidity, surface functionality, layer count, and dosage. Inhalation, ingestion, skin penetration, and placement for biomedical applications comprise four paths to the entry of any nanoparticles to the human body. How nanoparticles enter the inside body, the dose and duration have a substantial influence on the amount and degree of toxicity. Other major factors, such as dosage and exposure length also play a significant impact on toxicity levels (Citation136).
Impact of GBCs on the environment
Large-scale manufacturing, straining from enriched products, accidental spills during manufacturing and inadequate discharge of derivative waste might lead to large environmental releases and buildup of GBCs (Citation97). It is thus of essential significance to examine the ecotoxicity of GBCs. A huge variety of species, particularly bacteria, algae, plants, vertebrates, and invertebrates, have been examined in several habitats for this purpose, especially under the Graphene Flagship (Citation137).
Bacteria are at the base of trophic chains in the environment, participating in many phases of the nutrient cycle, and have complicated relationships with other creatures, therefore studying their impact on bacteria is critical. Compared to other biological systems, the effects of GBCs on bacteria have been extensively researched. Escherichia coli is the most often used laboratory model. The reported toxicity toward E. coli and other bacteria has been linked to direct interaction between GBCs and bacteria in several investigations. The antibacterial activity of four different GBCs (graphite, GO, and rGO) against E. coli was studied (Citation138). GO dispersions, followed by rGO, graphite oxide, and graphite showed the best antibacterial action. The physical contact between microbes and carbon-based nanomaterials (carbon nanotubes, GO, and fullerene) influenced cell membrane, metabolic activities, and microbe shape (Citation139). Alayande et al. (Citation140) suggested that graphene and GO nanosheets can cause the inner and outer cell membranes of E. coli to degrade using both experimental and theoretical (modeling) techniques. The strength of E. coli is thereby diminished. Reduction and hydration techniques are used to create a GO library with diverse amounts of hydroxyl, oxidation, and carbon radicals to estimate the influence on antibacterial activity in a more recent work (Citation141).
Furthermore, Guoet et al. (Citation137) discovered that GO promoted E. coli and S. aureus cell proliferation, biofilm formation, and biofilm development, but rGO hindered cell proliferation and biofilm formation. Lu et al. (Citation142) oriented GO nanoparticles in a magnetism, fixed them by cross-linking the underlying matrix, and exposed them on the substrate by oxidative etching to explore the orientation-dependent interaction of GBCs with bacteria. When it comes to GO orientations, the vertically oriented GO nanosheets had better antibacterial action against E. coli (Citation143). The scientists hypothesized that the antibacterial process needs cell membrane invasion and that the improved antimicrobial activity of the film with vertically oriented GO is owing to a higher density of edges with a more suitable orientation for membrane rupture. GBCs ecotoxicity was tested on a variety of framework and non-model photoautotrophs, ranging from cyanobacteria to angiosperms (Citation144). All of these species have a cell wall with a distinctive component and ultrastructure (peptidoglycans in cyanobacteria, cellulose in algae, and embryophytes), which is sometimes complemented by other exterior components.
Renewable energy fluctuates from time to weather and therefore needs to be balanced to provide a steady electricity supply. There are several balancing solutions, with microgrids deployed locally that store and manage power distribution and balancing impacts. Other solutions involve huge storage and supplementary (cross-country) grid connections with improved grid capacity (Citation145). These options might accommodate more sources of variable generation without affecting local resilience. The thickness and complexity of the cell wall in seed plants, for example, change dramatically from the pectic-rich cell walls, which are ordinarily a fine, flexible, and adaptable layer formed when the cell is growing, to the dense secondary wall, composed of cellulose, xylan, and lignin, which enhances and waterproofs the wall just after a cell has fully developed (Citation146).
Being the bottom participant of the trophic food chain in aquatic habitats, microalgae are of key importance ecologically as primary producers. In algae, the toxicity of carbon-based nanomaterials is mostly due to cell surface interactions, but it can also be owing to other causes such as shadowing (which reduces photosynthetic activity), oxidative stress, or nutrient sequestration. Scenedesmus obliquus growth was decreased and chlorophyll a and chlorophyll b levels were inhibited after 72 h of exposure to rGO, presumably owing to increased oxidative stress (Citation147).
Summary
In this paper, we summarize the characteristics, production processes, and functionalisms of the graphene and its derivatives as central element for composites in general and biomaterials in particular. Graphene may be concluded as altering nanomaterials in a number of disciplines of science, medicine, and engineering with intriguing possibilities. As described above, it has other important characteristics such as its chemical stability, biocompatibility, broad surface area, ability to operate with different functionalities, excellent mechanical properties (both adaptability and steadiness), and external characteristics, which is nearly 200% times stronger than steel and exhibit an extremely high electric conductivity. Thanks to these features, GBCs increase their attention and give additional opportunities for study and development in translational medicine applications. The biomedical application is based on their mechanical, thermal, and electrochemical characteristics, concerning GBCs specific physiological characteristics. Many publications have utilized GBCs in cancer treatment as well as photo-thermal therapy and photodynamic therapy, gene/drug administration, and anti-infection.
The vital research concerns are at cell-level toxicity instead of genetic interactions of GBCs. In environmental applications, other GBCs have potential applications and have been considered highly influential in this case. The GBCs (including porous 3D graphene materials) are used as pollutant removals, which facilitate the removal of radionuclide, organic, and inorganic pollutants from the environment. The interest in graphene has increased over the last few years, referring to a new approach for biomedical uses. Several findings indicate that graphene is better when transformed into nanocomposite graphene in terms of biodegradability, biocompatibility, and cytotoxicity. In therapeutic approaches such as the transport of medications and bio-imaging applications, usually, the bio-functionality of the graphene is extensive. Graphene, among other things, was examined for a wide range of applications in oncology therapies, which can effectively deliver drugs, including doxorubicin, and some other pharmaceuticals for the effective targeted administration of medications. Given the medication concentration, the absorption and efficacy of graphene as well as its derived products would be further explored in the biomedical domains.
On assessing toxicity, there remain confusing data on physicochemical information, such as shape, volume, agglomerative rate, layer thickness, and lateral dimensions based on a variety of graphene types. Graphene derivatives must be validated for safe usage before utilization in biological applications.
Future perspectives
Applications of GBCs have rapidly grown to include numerous sectors; however, the biological connections of GBCs are still uncertain. The experimental toxicity findings are more confined to in vitro than in vivo. To further develop their uses in the field of biomedicine, human safety should be considered. For this reason, the long-term toxic effects of GBCs need to be further explored. Before the toxicological assessment is conducted, the available literature does not give thorough information about the different synthesis and characterization methods. In particular, the detailed knowledge of adsorption, scattering, and toxicity-centered GBCs products should be emphasized and the transition is advocated. The lungs and liver are currently mainly targeted by GBCs. Studies regarding other organs, especially the central nervous system, are relatively limited. GBCs may especially disturb the neuroendocrine/reproductive organs due to their good physicochemical features. To our knowledge, the GBCs reports are quite restricted as endocrine disrupters. Further research is also needed in these fields. GBCs may also interact with DNA and impact the human population's genetics in this way. Studies to understand transgenerational effects or impacts of GBCs on the epigenome are thus necessary. Reina et al. (Citation38) proposed guidelines for GBCs biocompatibility assessment and implementation. These recommendations include extensive definition and regulation of GBCs. It is helpful to identify the structural/property connections and, hence, the appropriate connections to established biological assays of graphene-based materials.
Acknowledgements
Authors’ contributions: Selsabil Rokia Laraba: Investigation, Conceptualization, Writing – Original Draft; Wei Luo: Validation, Writing – Review; Amine Rezzoug: Visualization, Writing – Review & Editing; Qurat ul ain Zahra: Writing – Review & Editing; Shihao Zhang: Validation; Bozhen Wu: Validation; Wen Chen: Validation; Lan Xiao: Writing – Review & Editing; Yuhao Yang: Writing – Review & Editing, Jie Wei: Project administration, Conceptualization, Methodology, Supervision; Yulin li: Project administration, Conceptualization, Methodology, Supervision.
Disclosure statement
No potential conflict of interest was reported by the author(s).
Additional information
Funding
References
- Xu, Y.; Bai, H.; Lu, G.; Li, C.; Shi, G. Flexible Graphene Films via the Filtration of Water-Soluble Noncovalent Functionalized Graphene Sheets. J. Am. Chem. Soc. 2008, 130 (18), 5856–5857.
- Choi, W.; Lahiri, I.; Seelaboyina, R.; Kang, Y.S. Synthesis of Graphene and its Applications: A Review. Crit. Rev. Solid State Mater. Sci. 2010, 35 (1), 52–71.
- Young, R.J.; Kinloch, I.A.; Gong, L.; Novoselov, K.S. The Mechanics of Graphene Nanocomposites: A Review. Compos. Sci. Technol. 2012, 72 (12), 1459–1476.
- Kim, H.; Abdala, A.A.; Macosko, C.W. Graphene/Polymer Nanocomposites. Macromolecules 2010, 43 (16), 6515–6530.
- Novoselov, K.S.; Geim, A.K.; Morozov, S.V.; Jiang, D.; Zhang, Y.; Dubonos, S.V.; Grigorieva, I.V.; Firsov, A.A. Electric Field Effect in Atomically Thin Carbon Films. Science 2004, 306 (5696), 666–669.
- Stoller, M.D.; Park, S.; Zhu, Y.; An, J.; Ruoff, R.S. Graphene-based Ultracapacitors. Nano Lett. 2008, 8 (10), 3498–3502.
- Geim, A. Graphene Prehistory. Phys. Scr. 2012, T146, 014003.
- Zhang, L.; Xia, J.; Zhao, Q.; Liu, L. Functional Graphene Oxide as a Nanocarrier for Controlled Loading and Targeted Delivery of Mixed Anticancer Drugs. Small 2010, 6 (4), 537–544.
- Kim, H.; Macosko, C.W. Morphology and Properties of Polyester/Exfoliated Graphite Nanocomposites. Macromolecules. 2008, 41 (9), 3317–3327.
- Kelly, B.T., Physics of Graphite, Applied Science: United Kingdom, 1981.
- Solís-Fernández, P.; Bissett, M.; Ago, H. Synthesis, Structure and Applications of Graphene-Based 2D Heterostructures. Chem. Soc. Rev. 2017, 46 (15), 4572–4613.
- Shi, G.; Araby, S.; Gibson, C.T.; Meng, Q.; Zhu, S.; Ma, J. Graphene Platelets and Their Polymer Composites: Fabrication, Structure, Properties, and Applications. Adv. Funct. Mater. 2018, 28 (19), 1706705.
- Qian, W.; Chen, Z.; Cottingham, S.; Merrill, W.A.; Swartz, N.A.; Goforth, A.M.; Clare, T.L.; Jiao, J. Surfactant-free Hybridization of Transition Metal Oxidenanoparticles with Conductive Graphene for High-Performance Supercapacitor. Green Chem. 2012, 14 (2), 371–377.
- Novoselov, K.S.; Fal′ko, V.I.; Colombo, L.; Gellert, P.R.; Schwab, M.G.; Kim, K. A Roadmap for Graphene. Nature 2012, 490 (7419), 192–200.
- Qian. W.; Greaney, P.A.; Fowler, S.; Chiu, S.K.; Goforth, A.M.; Jiao, J. Low-Temperature Nitrogen Doping in Ammonia Solution for Production of N-doped TiO2-Hybridized Graphene as a Highly Efficient Photocatalyst for Water Treatment. ACS Sustain. Chem. Eng. 2014, 2 (7), 1802–1810.
- Mousavi, S.M.; Low, F.W.; Hashemi, S.A.; Lai, C.W.; Ghasemi, Y.; Soroshnia, S.; Savardashtaki, A.; Babapoor, A.; Pynadathu Rumjit, N.; Goh, S.M.; Amin, N.; Tiong, S.K. Development of Graphene Based Nanocomposites Towards Medical and Biological Applications. Artif. Cells. Nanomed. Biotechnol. 2020, 48 (1), 1189–1205.
- Xu, H.; Wu, X.; Li, X.; Luo, C.; Liang, F.; Orignac, E.; Zhang, J.; Chu, J. Properties of Graphene-Metal Contacts Probed by Raman Spectroscopy. Carbon. N. Y. 2018, 127, 491–497.
- Hicks, J.; Shepperd, K.; Wang, F.; Conrad, E.H. The Structure of Graphene Grown on the SiC Surface. J. Phys. D: Appl. Phys. 2012, 45 (15), 154002.
- Imanzadeh, H.; Bakirhan, N.K.; Kuralay, F.; Amiri, M.; Ozkan, S.A. Achievements of Graphene and Its Derivatives Materials on Electrochemical Drug Assays and Drug-DNA Interactions. Crit. Rev. Anal. Chem. 2021, 41 (9), 1–22.
- Bonaccorso, F.; Lombardo, A.; Hasan, T.; Sun, Z.; Colombo, L.; Ferrari, A.C. Production and Processing of Graphene and 2d Crystals. Mater. Today 2012, 15 (12), 564–589.
- Huang, X.; Guan, J.; Lin, Z.; Liu, B.; Xing, S.; Wang, W.; Guo, J. Epitaxial Growth and Band Structure of Te Film on Graphene. Nano Lett. 2017, 17 (8), 4619–4623.
- Chen, Y.; Li, M.-C.; Wang, Q.-M.; Wang, G.-S.; Wei, X.; Song, G.-F.; Kong, X.-M.; Xu, Y.; Liu, Y. Structure and Electronic Properties of Closed-Ring Defects in Epitaxial Graphene. Mater. Res. Express 2020, 7 (5), 055602.
- Tan, H.; Wang, D.; Guo, Y. Thermal Growth of Graphene: A Review. Coat. 2018, 8 (1), 40.
- Piñas, J.A.; Andrade, T.S.; Oliveira, A.T.; Salomão, P.E.A.; Rodriguez, M.; Silva, A.C.; Oliveira, H.S.; Monteiro, D.S.; Pereira, M.C. Production of Reduced Graphene Oxide Platelets from Graphite Flakes Using the Fenton Reaction as an Alternative to Harmful Oxidizing Agents. J. Nanomater. 2019, 2019, 5736563.
- Ton, N.N.T.; Dao, A.T.N.; Kato, K.; Ikenaga, T.; Trinh, D.X.; Taniike, T. One-pot Synthesis of TiO2/Graphene Nanocomposites for Excellent Visible Light Photocatalysis Based on Chemical Exfoliation Method. Carbon. N. Y. 2018, 133, 109–117.
- Wang, C.; Vinodgopal, K.; Dai, G.-P. Large-area Synthesis and Growth Mechanism of Graphene by Chemical Vapor Deposition. Chem. Vap. Depos. Nanotechnol. 2018, 5, 97–113.
- Pirzado, A.A.; Le Normand, F.; Romero, T.; Paszkiewicz, S.; Papaefthimiou, V.; Ihiawakrim, D.; Janowska, I. Few-layer Graphene from Mechanical Exfoliation of Graphite-Based Materials: Structure-Dependent Characteristics. Chem. Eng. 2019, 3 (2), 37.
- Ghasemi, F.; Razi, S.; Madanipour, K. Single-Step Laser-Assisted Graphene Oxide Reduction and Nonlinear Optical Properties Exploration via CW Laser Excitation. J. Electron. Mater. 2018, 47 (5), 2871–2879.
- Kymakis, E.; Petridis, C.; Anthopoulos, T.D.; Stratakis, E. Laser-assisted Reduction of Graphene Oxide for Flexible, Large-Area Optoelectronics. IEEE J. Sel. Top. Quantum Electron. 2013, 20 (1), 106–115.
- Criado, A.; Melchionna, M.; Marchesan, S.; Prato, M. The Covalent Functionalization of Graphene on Substrates. Angew. Chem. Int. Ed. 2015, 54 (37), 10734–10750.
- Sturala, J.; Luxa, J.; Pumera, M.; Sofer, Z. Chemistry of Graphene Derivatives: Synthesis, Applications, and Perspectives. Chem. Eur. J. 2018, 24 (23), 5992–6006.
- Hossain, M.Z.; Razak, M.B.A.; Yoshimoto, S.; Mukai, K.; Koitaya, T.; Yoshinobu, J.; Sone, H.; Hosaka, S.; Hersam, M.C. Aqueous-phase Oxidation of Epitaxial Graphene on the Silicon Face of SiC (0001). J. Phys. Chem. C 2014, 118 (2), 1014–1020.
- Hossain, M.Z.; Johns, J.E.; Bevan, K.H.; Karmel, H.J.; Liang, Y.T.; Yoshimoto, S.; Mukai, K.; Koitaya, T.; Yoshinobu, J.; Kawai, M.; Lear, A.M.; Kesmodel, L.L.; Tait, S.L.; Hersam, M.C. Chemically Homogeneous and Thermally Reversible Oxidation of Epitaxial Graphene. Nat. Chem. 2012, 4 (4), 305–309.
- Yuan, L.; Zhang, C.; Zhang, X.; Lou, M.; Ye, F.; Jacobson, C.R.; Dong, L.; Zhou, L.; Lou, M.; Cheng, Z.; Ajayan, P.M.; Nordlander, P.; Halas, N.J. Photocatalytic Hydrogenation of Graphene Using Pd Nanocones. Nano Lett. 2019, 19 (7), 4413–4419.
- Elias, D.C.; Nair, R.R.; Mohiuddin, T.M.G.; Morozov, S.V.; Blake, P.; Halsall, M.P.; Ferrari, A.C.; Boukhvalov, D.W.; Katsnelson, M.I.; Geim, A.K.; Novoselov, K.S. Control of Graphene's Properties by Reversible Hydrogenation: Evidence for Graphane. Sci. 2009, 323 (5914), 610–613.
- Zhou, S.; Shang, L.; Zhao, Y.; Shi, R.; Waterhouse, G.I.N.; Huang, Y.; Zheng, L.; Zhang, T. Pd Single-Atom Catalysts on Nitrogen-Doped Graphene for the Highly Selective Photothermal Hydrogenation of Acetylene to Ethylene. Adv. Mater. 2019, 31 (18), 1900509.
- Bullock, C.J.; Bussy, C. Biocompatibility Considerations in the Design of Graphene Biomedical Materials. Adv. Mater. Interfaces. 2019, 6 (11), 1900229.
- Reina, G.; González-Domínguez, J.M.; Criado, A.; Vázquez, E.; Bianco, A.; Prato, M. Promises, Facts and Challenges for Graphene in Biomedical Applications. Chem. Soc. Rev. 2017, 46 (15), 4400–4416.
- Adhikari, M.; Orasugh, J.T.; Chattopadhyay, D. Biomedical Application of Polymer-Graphene Composites, in Polymer Nanocomposites Containing Graphene; Elsevier, Woodhead Publishing Limited: Cambridge, 2022; pp 507–535.
- Marsden, A.J.; Papageorgiou, D.G.; Vallés, C.; Liscio, A.; Palermo, V.; Bissett, M.A.; Young, R.J.; Kinloch, I.A. Electrical Percolation in Graphene–Polymer Composites. 2D Mater. 2018, 5 (3), 032003.
- Du, J.; Cheng, H.M. The Fabrication, Properties, and Uses of Graphene/Polymer Composites. Macromol. Chem. Phys. 2012, 213 (10–11), 1060–1077.
- Silva, M.; Leow, C.; Kreider, P.B.; Notthoff, C.; Kluth, P.; Tricoli, A.; Compston, P. 3D Printing of Graphene-Based Polymeric Nanocomposites for Biomedical Applications. Funct Compos. Mater. 2021, 2 (1), 1–21.
- Liu, T.; Zhu, C.; Wu, W.; Liao, K.-N.; Gong, X.; Sun, Q.; Li, R.K.Y. Facilely Prepared Layer-by-Layer Graphene Membrane-Based Pressure Sensor with High Sensitivity and Stability for Smart Wearable Devices. J. Mater. Sci. Technol. 2020, 45, 241–247.
- Lee, T.; Yun, T.; Park, B.; Sharma, B.; Song, H.-K.; Kim, B.-S. Hybrid Multilayer Thin Film Supercapacitor of Graphene Nanosheets with Polyaniline: Importance of Establishing Intimate Electronic Contact Through Nanoscale Blending. J. Mater. Chem. 2012, 22 (39), 21092–21099.
- Park, J.S.; Cho, S.M.; Kim, W.-J.; Yoo, P.J. Fabrication of Graphene Thin Films Based on Layer-by-Layer Self-Assembly of Functionalized Graphene Nanosheets. ACS Appl. Mater. Interfaces 2011, 3 (2), 360–368.
- Lee, T.; Min, S.H.; Gu, M.; Jung, Y.K.; Seong, D.G.; Kim, B.-S. Layer-by-layer Assembly for Graphene-Based Multilayer Nanocomposites: Synthesis and Applications. Chem. Mater. 2015, 27 (11), 3785–3796.
- Dubey, N.; Bentini, R.; Islam, I.; Cao, T.; Castro Neto, A.H.; Rosa, V. Graphene: A Versatile Carbon-Based Material for Bone Tissue Engineering. Stem. Cells. Int. 2015, 2015, 1–12.
- Vlassiouk, I.; Polizos, G.; Cooper, R.; Ivanov, I.; Keum, J.K.; Paulauskas, F.; Datskos, P.; Smirnov, S. Strong and Electrically Conductive Graphene-Based Composite Fibers and Laminates. ACS Appl. Mater. Interfaces 2015, 7 (20), 10702–10709.
- Ferrari, A.C.; Bonaccorso, F.; Fal'ko, V.; Novoselov, K.S.; Roche, S.; Bøggild, P.; Borini, S.; Koppens, F.H.L.; Palermo, V.; Pugno, N. Science and Technology Roadmap for Graphene, Related two-Dimensional Crystals, and Hybrid Systems. Nanoscale. 2015, 7 (11), 4598–4810.
- Lee, C.; Wei, X.; Kysar, J.W.; Hone, J. Measurement of the Elastic Properties and Intrinsic Strength of Monolayer Graphene. Science 2008, 321 (5887), 385–388.
- Dikin, D.A.; Stankovich, S.; Zimney, E.J.; Piner, R.D.; Dommett, G.H.B.; Evmenenko, G.; Nguyen, S.T.; Ruoff, R.S. Preparation and Characterization of Graphene Oxide Paper. Nature 2007, 448 (7152), 457–460.
- Bai, J.; Allaoui, A. Effect of the Length and the Aggregate Size of MWNTs on the Improvement Efficiency of the Mechanical and Electrical Properties of Nanocomposites—Experimental Investigation. Compos. Part A Appl. Sci. Manuf. 2003, 34 (8), 689–694.
- Bao, C.; Guo, Y.; Song, L.; Hu, Y. Poly (Vinyl Alcohol) Nanocomposites Based on Graphene and Graphite Oxide: A Comparative Investigation of Property and Mechanism. J. Mater. Chem. 2011, 21 (36), 13942–13950.
- Wang, J.; Hu, H.; Xu, C.; Zhang, M.; Shang, X. Preparation and Mechanical and Electrical Properties of Graphene Nanosheets–Poly (Methyl Methacrylate) Nanocomposites via in Situ Suspension Polymerization. J. Appl. Polym. Sci. 2011, 122 (3), 1866–1871.
- Ramanathan, T.; Abdala, A.A.; Stankovich, S.; Dikin, D.A.; Herrera-Alonso, M.; Piner, R.D.; Adamson, D.H.; Schniepp, H.C.; Chen, X.; Ruoff, R.S.; Nguyen, S.T.; Aksay, I.A.; Prud'Homme, R.K.; Brinson, L.C. Functionalized Graphene Sheets for Polymer Nanocomposites. Nat. Nanotechnol. 2008, 3 (6), 327–331.
- Zhu, Y.; Murali, S.; Cai, W.; Li, X.; Suk, J.W.; Potts, J.R.; Ruoff, R.S. Graphene and Graphene Oxide: Synthesis, Properties, and Applications. Adv. Mater. 2010, 22 (35), 3906–3924.
- Phiri, J.; Johansson, L.-S.; Gane, P.; Maloney, T. A Comparative Study of Mechanical, Thermal and Electrical Properties of Graphene-, Graphene Oxide-and Reduced Graphene Oxide-Doped Microfibrillated Cellulose Nanocomposites. Compos. Part B: Eng. 2018, 147, 104–113.
- Yu, A.; Ramesh, P.; Itkis, M.E.; Bekyarova, E.; Haddon, R.C. Graphite Nanoplatelet− Epoxy Composite Thermal Interface Materials. J. Phys. Chem. C 2007, 111 (21), 7565–7569.
- Guo, Y.; Bao, C.; Song, L.; Yuan, B.; Hu, Y. In Situ Polymerization of Graphene, Graphite Oxide, and Functionalized Graphite Oxide Into Epoxy Resin and Comparison Study of on-the-Flame Behavior. Ind. Eng. Chem. Res. 2011, 50 (13), 7772–7783.
- Fang, M.; Wang, K.; Lu, H.; Yang, Y.; Nutt, S. Single-layer Graphene Nanosheets with Controlled Grafting of Polymer Chains. J. Mater. Chem. 2010, 20 (10), 1982–1992.
- Liang, J.; Huang, Y.; Zhang, L.; Wang, Y.; Ma, Y.; Guo, T.; Chen, Y. Molecular-Level Dispersion of Graphene Into Poly (Vinyl Alcohol) and Effective Reinforcement of Their Nanocomposites. Adv. Funct. Mater. 2009, 19 (14), 2297–2302.
- Kim, I.H.; Jeong, Y.G. Polylactide/Exfoliated Graphite Nanocomposites with Enhanced Thermal Stability, Mechanical Modulus, and Electrical Conductivity. J. Polym. Sci., Part B: Polym. Phys. 2010, 48 (8), 850–858.
- Hu, Y.; Shen, J.; Li, N.; Ma, H.; Shi, M.; Yan, B.; Huang, W.; Wang, W.; Ye, M. Comparison of the Thermal Properties Between Composites Reinforced by Raw and Amino-Functionalized Carbon Materials. Compos. Sci. Technol. 2010, 70 (15), 2176–2182.
- Bui, K.; Duong, H.M.; Striolo, A.; Papavassiliou, D.V. Effective Heat Transfer Properties of Graphene Sheet Nanocomposites and Comparison to Carbon Nanotube Nanocomposites. J. Phys. Chem. C 2011, 115 (10), 3872–3880.
- Bai, S.; Jiang, L.; Xu, N.; Jin, M.; Jiang, S. Enhancement of Mechanical and Electrical Properties of Graphene/Cement Composite due to Improved Dispersion of Graphene by Addition of Silica Fume. Constr. Build. Mater. 2018, 164, 433–441.
- Cusati, T.; Fiori, G.; Gahoi, A.; Passi, V.; Lemme, M.C.; Fortunelli, A.; Iannaccone, G. Electrical Properties of Graphene-Metal Contacts. Sci. Rep. 2017, 7 (1), 1–11.
- Nimbalkar, A.; Kim, H. Opportunities and Challenges in Twisted Bilayer Graphene: A Review. Nano-Micro Lett. 2020, 12 (1), 1–20.
- Pham, V.H.; Cuong, T.V.; Dang, T.T.; Hur, S.H.; Kong, B.-S.; Kim, E.J.; Shin, E.W.; Chung, J.S. Superior Conductive Polystyrene–Chemically Converted Graphene Nanocomposite. J. Mater. Chem. 2011, 21 (30), 11312–11316.
- Raghu, A.V.; Lee, Y.R.; Jeong, H.M.; Shin, C.M. Preparation and Physical Properties of Waterborne Polyurethane/Functionalized Graphene Sheet Nanocomposites. Macromol. Chem. Phys. 2008, 209 (24), 2487–2493.
- Du, J.; Zhao, L.; Zeng, Y.; Zhang, L.; Li, F.; Liu, P.; Liu, C. Comparison of Electrical Properties Between Multi-Walled Carbon Nanotube and Graphene Nanosheet/High Density Polyethylene Composites with a Segregated Network Structure. Carbon. N. Y. 2011, 49 (4), 1094–1100.
- Stankovich, S.; Dikin, D.A.; Dommett, G.H.B.; Kohlhaas, K.M.; Zimney, E.J.; Stach, E.A.; Piner, R.D.; Nguyen, S.T.; Ruoff, R.S. Graphene-Based Composite Materials. Nature 2006, 442 (7100), 282–286.
- Hyo Won, K.; Kim, H.W.; Yoon, H.W.; Yoon, S.-M.; Yoo, B.M.; Ahn, B.K.; Cho, Y.H.; Shin, H.J.; Yang, H.; Paik, U.; Kwon, S.; Choi, J.-Y.; Park, H.B. Selective Gas Transport Through Few-Layered Graphene and Graphene Oxide Membranes. Science 2013, 342 (6154), 91–95.
- Wang, J.; Xu, C.; Zhang, M.; Shang, X. Preparation of Graphene/Poly (Vinyl Alcohol) Nanocomposites with Enhanced Mechanical Properties and Water Resistance. Polym. Int. 2011, 60 (5), 816–822.
- Kim, H.M.; Lee, J.K.; Lee, H.S. Transparent and High gas Barrier Films Based on Poly (Vinyl Alcohol)/Graphene Oxide Composites. Thin Solid Films 2011, 519 (22), 7766–7771.
- Kim, H.; Miura, Y.; Macosko, C.W. Graphene/Polyurethane Nanocomposites for Improved Gas Barrier and Electrical Conductivity. Chem. Mater. 2010, 22 (11), 3441–3450.
- Kim, H.; Macosko, C.W. Processing-property Relationships of Polycarbonate/Graphene Composites. Polymer 2009, 50 (15), 3797–3809.
- Tadyszak, K.; Wychowaniec, J.K.; Litowczenko, J. Biomedical Applications of Graphene-Based Structures. Nanomaterials 2018, 8 (11), 944.
- Aydin, T.; Gurcan, C.; Taheri, H.; Yilmazer, A. Graphene Based Materials in Neural Tissue Regeneration. Cell Biol. Translational Med. 2018, 3, 129–142.
- Xia, M.-Y.; Xie, Y.; Yu, C.-H.; Chen, G.-Y.; Li, Y.-H.; Zhang, T.; Peng, Q. Graphene-based Nanomaterials: The Promising Active Agents for Antibiotics-Independent Antibacterial Applications. J. Controlled Release 2019, 307, 16–31.
- Zare, P.; Aleemardani, M.; Seifalian, A.; Bagher, Z.; Seifalian, A.M. Graphene Oxide: Opportunities and Challenges in Biomedicine. Nanomaterials 2021, 11 (5), 1083.
- Fatima, N.; Qazi, U.Y.; Mansha, A.; Bhatti, I.A.; Javaid, R.; Abbas, Q.; Nadeem, N.; Rehan, Z.A.; Noreen, S.; Zahid, M. Recent Developments for Antimicrobial Applications of Graphene-Based Polymeric Composites: A Review. J. Ind. Eng. Chem. 2021, 100, 40–58.
- Wan, C.; Chen, B. Poly (ϵ-Caprolactone)/Graphene Oxide Biocomposites: Mechanical Properties and Bioactivity. Biomed. Mater. 2011, 6 (5), 055010.
- Kumar, R.; Sahoo, S.; Joanni, E.; Singh, R.K.; Yadav, R.M.; Verma, R.K.; Singh, D.P.; Tan, W.K.; Pérez del Pino, A.; Moshkalev, S.A.; Matsuda, A. A Review on Synthesis of Graphene, h-BN and MoS 2 for Energy Storage Applications: Recent Progress and Perspectives. Nano Res. 2019, 12 (11), 2655–2694.
- Sookhakian, M.; Basirun, W.J.; Teridi, M.A.M.; Mahmoudian, M.R.; Azarang, M.; Zalnezhad, E.; Yoon, G.H.; Alias, Y. Prussian Blue-Nitrogen-Doped Graphene Nanocomposite as Hybrid Electrode for Energy Storage Applications. Electrochim. Acta 2017, 230, 316–323.
- Nag, A.; Mitra, A.; Mukhopadhyay, S.C. Graphene and its Sensor-Based Applications: A Review. Sens. Actuators, A 2018, 270, 177–194.
- Omar, N.A.S.; Fen, Y.; Saleviter, S.; Daniyal, W.; Anas, N.; Ramdzan, N.; Roshidi, M. Development of a Graphene-Based Surface Plasmon Resonance Optical Sensor Chip for Potential Biomedical Application. Materials 2019, 12 (12), 1928.
- Rodder, M.A.; Vasishta, S.; Dodabalapur, A. Double-gate MoS2 Field-Effect Transistor with a Multilayer Graphene Floating Gate: A Versatile Device for Logic, Memory, and Synaptic Applications. ACS Appl. Mater. Interfaces. 2020, 12 (30), 33926–33933.
- Zafar, Z.; Wang, W.-H.; Liu, M.-Y.; Ni, Z.-H.; You, Y.-M. Nonvolatile Memory Based on Molecular Ferroelectric/Graphene Field Effect Transistor. ACS Appl. Mater. Interfaces. 2018, 10 (45), 39187–39193.
- Yao, Y.; Ping, J. Recent Advances in Graphene-Based Freestanding Paper-Like Materials for Sensing Applications. TrAC, Trends Anal. Chem. 2018, 105, 75–88.
- Lin, S.; Ju, S.; Shi, G.; Zhang, J.; He, Y.; Jiang, D. Ultrathin Nitrogen-Doping Graphene Films for Flexible and Stretchable EMI Shielding Materials. J. Mater. Sci. 2019, 54 (9), 7165–7179.
- Yao, Y.; Jiang, C.; Ping, J. Flexible Freestanding Graphene Paper-Based Potentiometric Enzymatic Aptasensor for Ultrasensitive Wireless Detection of Kanamycin. Biosens. Bioelectron. 2019, 123, 178–184.
- Jiang, C.; Li, X.; Yao, Y.; Lan, L.; Shao, Y.; Zhao, F.; Ying, Y.; Ping, J. A Multifunctional and Highly Flexible Triboelectric Nanogenerator Based on MXene-Enabled Porous Film Integrated with Laser-Induced Graphene Electrode. Nano Energy 2019, 66, 104121.
- Won, S.; Van Lam, D.; Lee, J.Y.; Jung, H.-J.; Hur, M.; Kim, K.-S.; Lee, H.-J.; Kim, J.-H. Graphene-based Stretchable and Transparent Moisture Barrier. Nanotechnology 2018, 29 (12), 125705.
- Ruan, H.; Zhang, Q.; Liao, W.; Li, Y.; Huang, X.; Xu, X.; Lu, S. Enhancing Tribological, Mechanical, and Thermal Properties of Polyimide Composites by the Synergistic Effect Between Graphene and Ionic Liquid. Mater. Des. 2020, 189, 108527.
- Zou, D.; Ma, X.; Liu, X.; Zheng, P.; Hu, Y. Thermal Performance Enhancement of Composite Phase Change Materials (PCM) Using Graphene and Carbon Nanotubes as Additives for the Potential Application in Lithium-ion Power Battery. Int. J. Heat Mass Transfer 2018, 120, 33–41.
- Fan, Z.; Pereira, L.F.C.; Hirvonen, P.; Ervasti, M.M.; Elder, K.R.; Donadio, D.; Ala-Nissila, T.; Harju, A. Thermal Conductivity Decomposition in two-Dimensional Materials: Application to Graphene. Phys. Rev. B 2017, 95 (14), 144309.
- Zhang, F.; Li, Y.-H.; Li, J.-Y.; Tang, Z.-R.; Xu, Y.-J. 3D Graphene-Based Gel Photocatalysts for Environmental Pollutants Degradation. Environ. Pollut. 2019, 253, 365–376.
- Zakeri, A.; Kouhbanani, M.A.J.; Beheshtkhoo, N.; Beigi, V.; Mousavi, S.M.; Hashemi, S.A.R.; Karimi Zade, A.; Amani, A.M.; Savardashtaki, A.; Mirzaei, E.; Jahandideh, S.; Movahedpour, A. Polyethylenimine-based Nanocarriers in Co-Delivery of Drug and Gene: A Developing Horizon. Nano Rev. Exp. 2018, 9 (1), 1488497.
- Choudhary, P.; Das, S.K. Bio-reduced Graphene Oxide as a Nanoscale Antimicrobial Coating for Medical Devices. ACS Omega 2019, 4 (1), 387–397.
- Qu, Y.; He, F.; Yu, C.; Liang, X.; Liang, D.; Ma, L.; Zhang, Q.; Lv, J.; Wu, J. Advances on Graphene-Based Nanomaterials for Biomedical Applications. Mater. Sci. Eng. C 2018, 90, 764–780.
- Sun, X.; Liu, Z.; Welsher, K.; Robinson, J.T.; Goodwin, A.; Zaric, S.; Dai, H. Nano-graphene Oxide for Cellular Imaging and Drug Delivery. Nano Res. 2008, 1 (3), 203–212.
- Ma, X.; Tao, H.; Yang, K.; Feng, L.; Cheng, L.; Shi, X.; Li, Y.; Guo, L.; Liu, Z. A Functionalized Graphene Oxide-Iron Oxide Nanocomposite for Magnetically Targeted Drug Delivery, Photothermal Therapy, and Magnetic Resonance Imaging. Nano Res. 2012, 5 (3), 199–212.
- Yang, K.; Feng, L.; Shi, X.; Liu, Z. Nano-graphene in Biomedicine: Theranostic Applications. Chem. Soc. Rev. 2013, 42 (2), 530–547.
- Yang, K.; Hu, L.; Ma, X.; Ye, S.; Cheng, L.; Shi, X.; Li, C.; Li, Y.; Liu, Z. Multimodal Imaging Guided Photothermal Therapy Using Functionalized Graphene Nanosheets Anchored with Magnetic Nanoparticles. Adv. Mater. 2012, 24 (14), 1868–1872.
- Chen, J.; Sekone, A.K.; Lu, M.-C.; Liu, C.-A.; Lee, M.-T. Indocyanine Green Loaded Reduced Graphene Oxide for in Vivo Photoacoustic/Fluorescence Dual-Modality Tumor Imaging. Nanoscale Res. Lett. 2016, 11 (1), 1–11.
- Yue, L.; Wang, J.; Dai, Z.; Hu, Z.; Chen, X.; Qi, Y.; Zheng, X.; Yu, D. pH-responsive, Self-Sacrificial Nanotheranostic Agent for Potential in Vivo and in Vitro Dual Modal MRI/CT Imaging, Real-Time, and In Situ Monitoring of Cancer Therapy. Bioconjugate Chem. 2017, 28 (2), 400–409.
- Lin, J.; Huang, Y.; Huang, P. Graphene-based Nanomaterials in Bioimaging. Biomed. Appl. Funct. Nanomater. 2018, 1, 247–287.
- Mansuriya, B.D.; Altintas, Z. Applications of Graphene Quantum Dots in Biomedical Sensors. Sensors 2020, 20 (4), 1072.
- Ping, J.; Wang, Y.; Wu, J.; Ying, Y. Development of an Electrochemically Reduced Graphene Oxide Modified Disposable Bismuth Film Electrode and its Application for Stripping Analysis of Heavy Metals in Milk. Food Chem. 2014, 151, 65–71.
- Feng, W.; Wang, Z. Biomedical Applications of Chitosan-Graphene Oxide Nanocomposites. Iscience 2022, 25 (1), 103629.
- Huang, H.; Su, S.; Wu, N.; Wan, H.; Wan, S.; Bi, H.; Sun, L. Graphene-based Sensors for Human Health Monitoring. Front. Chem. 2019, 7, 399.
- Mehmood, A.; Mubarak, N.M.; Khalid, M.; Walvekar, R.; Abdullah, E.C.; Siddiqui, M.T.H.; Baloch, H.A.; Nizamuddin, S.; Mazari, S. Graphene Based Nanomaterials for Strain Sensor Application—A Review. J. Environmen. Chem. Eng. 2020, 8 (3), 103743.
- Singh, D.P.; Herrera, C.E.; Kumar, R. Graphene Oxide: An Efficient Material and Recent Approach for Biotechnological and Biomedical Applications. Mater. Sci. Eng. C 2018, 86, 173–197.
- Hashemzadeh, H.; Raissi, H. Understanding Loading, Diffusion and Releasing of Doxorubicin and Paclitaxel Dual Delivery in Graphene and Graphene Oxide Carriers as Highly Efficient Drug Delivery Systems. Appl. Surf. Sci. 2020, 500, 144220.
- Iannazzo, D.; Pistone, A.; Salamò, M.; Galvagno, S.; Romeo, R.; Giofré, S.V.; Branca, C.; Visalli, G.; Di Pietro, A. Graphene Quantum Dots for Cancer Targeted Drug Delivery. Int. J. Pharm. 2017, 518 (1–2), 185–192.
- Liu, X.; Cheng, X.; Wang, F.; Feng, L.; Wang, Y.; Zheng, Y.; Guo, R. Targeted Delivery of SNX-2112 by Polysaccharide-Modified Graphene Oxide Nanocomposites for Treatment of Lung Cancer. Carbohydr. Polym. 2018, 185, 85–95.
- Zare-Zardini, H.; Taheri-Kafrani, A.; Amiri, A.; Bordbar, A.-K. New Generation of Drug Delivery Systems Based on Ginsenoside Rh2-, Lysine-and Arginine-Treated Highly Porous Graphene for Improving Anticancer Activity. Sci. Rep. 2018, 8 (1), 1–15.
- Alemi, F.; Zarezadeh, R.; Sadigh, A.R.; Hamishehkar, H.; Rahimi, M.; Majidinia, M.; Asemi, Z.; Ebrahimi-Kalan, A.; Yousefi, B.; Rashtchizadeh, N. Graphene Oxide and Reduced Graphene Oxide: Efficient Cargo Platforms for Cancer Theranostics. J. Drug. Deliv. Sci. Technol. 2020, 60, 101974.
- Shin, S.R.; Li, Y.-C.; Jang, H.L.; Khoshakhlagh, P.; Akbari, M.; Nasajpour, A.; Zhang, Y.S.; Tamayol, A.; Khademhosseini, A. Graphene-based Materials for Tissue Engineering. Adv. Drug Delivery Rev. 2016, 105, 255–274.
- Goenka, S.; Sant, V.; Sant, S. Graphene-based Nanomaterials for Drug Delivery and Tissue Engineering. J. Control. Release 2014, 173, 75–88.
- Solanki, A.; Chueng, S.-T.D.; Yin, P.T.; Kappera, R.; Chhowalla, M.; Lee, K.-B. Axonal Alignment and Enhanced Neuronal Differentiation of Neural Stem Cells on Graphene-Nanoparticle Hybrid Structures. Adv. Mater. 2013, 25 (38), 5477–5482.
- Priyadarsini, S.; Mohanty, S.; Mukherjee, S.; Basu, S.; Mishra, M. Graphene and Graphene Oxide as Nanomaterials for Medicine and Biology Application. J. Nanostruct. Chem. 2018, 8 (2), 123–137.
- Zuchowska, A.; Chudy, M.; Dybko, A.; Brzozka, Z. Graphene as a new Material in Anticancer Therapy-in Vitro Studies. Sens. Actuators B: Chem. 2017, 243, 152–165.
- Usman, A.; Hussain, Z.; Riaz, A.; Khan, A.N. Enhanced Mechanical, Thermal and Antimicrobial Properties of Poly (Vinyl Alcohol)/Graphene Oxide/Starch/Silver Nanocomposites Films. Carbohydr. Polym. 2016, 153, 592–599.
- Li, P.; Sun, S.; Dong, A.; Hao, Y.; Shi, S.; Sun, Z.; Gao, G.; Chen, Y. Developing of a Novel Antibacterial Agent by Functionalization of Graphene Oxide with Guanidine Polymer with Enhanced Antibacterial Activity. Appl. Surf. Sci. 2015, 355, 446–452.
- Arriagada, P.; Palza, H.; Palma, P.; Flores, M.; Caviedes, P. Poly (Lactic Acid) Composites Based on Graphene Oxide Particles with Antibacterial Behavior Enhanced by Electrical Stimulus and Biocompatibility. J. Biomed. Mater. Res. A 2018, 106 (4), 1051–1060.
- Kumar, P.; Huo, P.; Zhang, R.; Liu, B. Antibacterial Properties of Graphene-Based Nanomaterials. Nanomaterials 2019, 9 (5), 737.
- Fu, L.; Xie, K.; Zheng, Y.; Zhang, L.; Su, W. Graphene ink Film Based Electrochemical Detector for Paracetamol Analysis. Electronics. 2018, 7 (2), 15.
- Jakus, A.E.; Secor, E.B.; Rutz, A.L.; Jordan, S.W.; Hersam, M.C.; Shah, R.N. Three-dimensional Printing of High-Content Graphene Scaffolds for Electronic and Biomedical Applications. ACS Nano 2015, 9 (4), 4636–4648.
- Shareena, T.P.D.; McShan, D.; Dasmahapatra, A.K.; Tchounwou, P. B. A Review on Graphene-Based Nanomaterials in Biomedical Applications and Risks in Environment and Health. Nano-micro Lett. 2018, 10 (3), 1–34.
- Pinto, A.M.; Magalhães, F.D.; Gonçalves, I.C. Polymer Surface Adsorption as a Strategy to Improve the Biocompatibility of Graphene Nanoplatelets. Colloids Surf. B 2016, 146, 818–824.
- Li, Y.; Wang, J.; Zhao, F.; Bai, B.; Nie, G.; Nel, A.E.; Zhao, Y. Nanomaterial Libraries and Model Organisms for Rapid High-Content Analysis of Nanosafety. Natl. Sci. Rev. 2018, 5 (3), 365–388.
- Graham, U.M.; Jacobs, G.; Yokel, R.A.; Davis, B.H.; Dozier, A.K.; Birch, M.E.; Tseng, M.T.; Oberdörster, G.; Elder, A.; DeLouise, L. From Dose to Response: In Vivo Nanoparticle Processing and Potential Toxicity. Model. Toxicity Nanoparticles 2017, 947, 71–100.
- Mao, L.; Morfeld, P.; Bruch, J.; Levy, L.; Ngiewih, Y.; Chaudhuri, I.; Muranko, H.J.; Myerson, R.; McCunney, R.J. Biodistribution and Toxicity of Radio-Labeled few Layer Graphene in Mice After Intratracheal Instillation. Part. Fibre Toxicol. 2015, 13 (1), 1–12.
- Czarny, B.; Georgin, D.; Berthon, F.; Plastow, G.; Pinault, M.; Patriarche, G.; Thuleau, A.; L’Hermite, M.M.; Taran, F.; Dive, V. Carbon Nanotube Translocation to Distant Organs After Pulmonary Exposure: Insights from in Situ 14C-Radiolabeling and Tissue Radioimaging. ACS Nano 2014, 8 (6), 5715–5724.
- Bussy, C.; Kostarelos, K. Culture Media Critically Influence Graphene Oxide Effects on Plasma Membranes. Chem 2017, 2 (3), 322–323.
- Guo, Z.; Xie, C.; Zhang, P.; Zhang, J.; Wang, G.; He, X.; Ma, Y.; Zhao, B.; Zhang, Z. Toxicity and Transformation of Graphene Oxide and Reduced Graphene Oxide in Bacteria Biofilm. Sci. Total Environ. 2017, 580, 1300–1308.
- Thakur, B.; Zhou, G.; Chang, J.; Pu, H.; Jin, B.; Sui, X.; Yuan, X.; Yang, C.-H.; Magruder, M.; Chen, J. Rapid Detection of Single E. Coli Bacteria Using a Graphene-Based Field-Effect Transistor Device. Biosens. Bioelectron. 2018, 110, 16–22.
- Xiong, T.; Yuan, X.; Wang, H.; Leng, L.; Li, H.; Wu, Z.; Jiang, L.; Xu, R.; Zeng, G. Implication of Graphene Oxide in Cd-Contaminated Soil: A Case Study of Bacterial Communities. J. Environ. Manag. 2018, 205, 99–106.
- Alayande, A.B.; Park, H.; Vrouwenvelder, J.S.; Kim, I.S. Implications of Chemical Reduction Using Hydriodic Acid on the Antimicrobial Properties of Graphene Oxide and Reduced Graphene Oxide Membranes. Small 2019, 15 (28), 1901023.
- Fadeel, B.; Bussy, C.; Merino, S.; Vázquez, E.; Flahaut, E.; Mouchet, F.; Evariste, L.; Gauthier, L.; Koivisto, A.J.; Vogel, U.; Martín, C.; Delogu, L.G.; Buerki-Thurnherr, T.; Wick, P.; Beloin-Saint-Pierre, D.; Hischier, R.; Pelin, M.; Candotto Carniel, F.; Tretiach, M.; Cesca, F.; Benfenati, F.; Scaini, D.; Ballerini, L.; Kostarelos, K.; Prato, M.; Bianco, A. Safety Assessment of Graphene-Based Materials: Focus on Human Health and the Environment. ACS Nano 2018, 12 (11), 10582–10620.
- Lu, X.; Feng, X.; Werber, J.R.; Chu, C.; Zucker, I.; Kim, J. -H.; Osuji, C.O.; Elimelech, M. Enhanced Antibacterial Activity Through the Controlled Alignment of Graphene Oxide Nanosheets. Proc. Natl. Acad. Sci. U.S.A. 2017, 114 (46), E9793–E9801.
- Li, R.; Mansukhani, N.D.; Guiney, L.M.; Ji, Z.; Zhao, Y.; Chang, C.H.; French, C.T.; Miller, J.F.; Hersam, M.C.; Nel, A.E.; Xia, T. Identification and Optimization of Carbon Radicals on Hydrated Graphene Oxide for Ubiquitous Antibacterial Coatings. ACS Nano 2016, 10 (12), 10966–10980.
- Navarro, E.; Baun, A.; Behra, R.; Hartmann, N.B.; Filser, J.; Miao, A.-J.; Quigg, A.; Santschi, P.H.; Sigg, L. Environmental Behavior and Ecotoxicity of Engineered Nanoparticles to Algae, Plants, and Fungi. Ecotoxicology 2008, 17 (5), 372–386.
- Cheng, C.; Li, S.; Thomas, A.; Kotov, N.A.; Haag, R. Functional Graphene Nanomaterials Based Architectures: Biointeractions, Fabrications, and Emerging Biological Applications. Chem. Rev. 2017, 117 (3), 1826–1914.
- Du, S.; Zhang, P.; Zhang, R.; Lu, Q.; Liu, L.; Bao, X.; Liu, H. Reduced Graphene Oxide Induces Cytotoxicity and Inhibits Photosynthetic Performance of the Green Alga Scenedesmus Obliquus. Chemosphere 2016, 164, 499–507.
- Zhao, J.; Cao, X.; Wang, Z.; Dai, Y.; Xing, B. Mechanistic Understanding Toward the Toxicity of Graphene-Family Materials to Freshwater Algae. Water Res. 2017, 111, 18–27.