ABSTRACT
Heavy metal compounds are used in a variety of industrial processes, including tanning, chrome plating, anti-corrosion treatments, and wood preservation. Heavy metal ion pollution in water and wastewater is often caused by industrial effluent discharge into open water sources. Toxic heavy metal ions such as As (III), Cr (VI), Cd (II), and Pb (II) are well-known and enter the body through a variety of pathways, including the food chain, respiration, skin absorption, and drinking water. These heavy metal ions produce oxidative stress in cells, resulting in cell organelle destruction. Heavy metals produce toxicity and may cause genetic material mutation or change, histone modification, and epigenetic alteration at various stages. Furthermore, heavy metals are linked to heart failure, renal damage, liver failure, and a variety of skin problems. For heavy metals cleanup, several standard approaches are utilized. Nonetheless, these technologies are costly and result in toxic sludge after treatment. As a result, there is an urgent need for an appropriate, environmentally safe, and efficient heavy metal removal technology. For heavy metal removal, microbial-based approaches are regarded as both environmentally benign and cost-effective. This review focuses on heavy metal pollution in water, its harmful consequences, and heavy metal cleanup by microbiological means.
1. Introduction
An excessively high concentration of unwanted chemicals in the air, water, or soil that exceeds the permissible threshold for such concentrations is referred to as environmental contamination. Changes in the natural environment harm animals and plants (Citation1). Heavy metals are naturally occurring compounds with a density higher than that of water molecules. Because of their extremely hazardous nature, metallic contaminants have piqued the interest of experts. These chemicals are naturally present in trace concentrations in ecosystems (Citation2). Few metal ions, however, exhibit greater toxicity values even at low concentrations (Citation3). Even in trace levels, metallic ions such as chromium (Cr), arsenic (As), lead (Pb), iron (Fe), cadmium (Cd), nickel (Ni), mercury (Hg), and cobalt (Co) are poisonous (Citation4). Metals such as Cr (III), Co (II), Zn (II), Fe (II), K (I), and Cu (II) are, on the other hand, engaged in a variety of metabolic functions. They are regarded as necessary metal ions for the development of living organisms. These heavy metals can be harmful if taken in excess and the body is unable to metabolize them. Furthermore, heavy metal ions penetrate the intracellular or extracellular area of bodily organs. In that instance, they can have several hazardous consequences (Citation5). Heavy metals may easily and swiftly permeate the cell membrane due to their high solubility in water (Citation6).
The initial stage of Pb (II) toxicity includes headache, memory loss and dullness (Citation7). It is also responsible for disturbance in the synthesis of red blood cells (RBCs) and causes anaemia. Pb (II) is also considered a potential carcinogen which causes several types of cancer (Citation8). Cd (II) toxicity is generally associated with cancer in the lungs, kidneys, prostate and stomach, disturbance in pulmonary function, decrease in olfactory function and bone mineral density and osteoporosis (Citation9). Arsenic (As) is a carcinogenic heavy metal ion which can cause cancer in humans. Pb (II) poisoning is commonly linked with liver damage, renal dysfunction, cardiac failure, and neurological impairment (Citation10). Headache, memory loss, and dullness are symptoms of early Pb (II) intoxication (Citation7). It also induces anaemia by interfering with the formation of red blood cells (RBCs). Pb (II) is also regarded as a possible carcinogen, causing a variety of cancers (Citation8). Cd (II) poisoning is typically linked to cancers of the lungs, kidneys, prostate, and stomach, as well as changes in pulmonary function, olfactory function, bone mineral density, and osteoporosis (Citation9). Arsenic (As) is a carcinogenic heavy metal ion that has been linked to cancer in humans (Citation11).
Because heavy metal ions are more harmful, it is vital to remove heavy metal ion pollution before discharging industrial effluent into the aquatic ecosystem. For heavy metal extraction from aqueous medium, several methods are used, including reverse osmosis, membrane filtration, ion exchange, solvent extraction, precipitation, flocculation, electro-dialysis, and coagulation (Citation12). These procedures are costly, only partially efficient in removing trace levels of metal solution from wastewater, and produce secondary chemical sludge (Citation13,Citation14). Biological treatments, on the other hand, such as bioaccumulation, bioreduction, phytoremediation, and biosorption, are cost-effective and environmentally beneficial strategies for eliminating heavy metals from wastewater (Citation15). Microorganisms have better heavy metal tolerance and are regarded as appropriate agents for heavy metal ion removal from polluted water (Citation15). Live bacteria, fungus, and microalgae cells are engaged in heavy metal ion removal via metabolically dependent and metabolically independent pathways (Citation15). Heavy metal ions binding on the microbial cell surface, as well as dead microbial biomass, are evaluated for metabolically independent heavy removal (Citation16). The microbial surface contains various functional groups, including carboxyl, nitrate, and hydroxyl, all of which play an important role in the biosorption of heavy metal ions (Citation17). Heavy metal ion removal is metabolically dependent on living microbial cells. Heavy metal ions enter the bacterial cell via cell surface receptors and cause oxidative stress in the cell. These heavy metal ions either accumulate in the cell through protein binding (metallothionine) or are reduced to a less harmful form (Citation18). Antioxidants present in microbial cells can minimize oxidative stress and enhance the buildup of heavy metal ions in the cells. Microbes are resistant to heavy metal ions due to the antioxidant system and the expression of heavy metal tolerant proteins in microbial cells. Heavy metal-resistant microorganisms may thrive in high concentrations of heavy metal stress and are thought to be good bioremediating agents (Citation18,Citation19).
This review is focused on water contamination due to heavy metal ions, their adverse effects on human health and heavy metals removal by microbial-based approach from contaminated water.
2. Source and toxicity of heavy metals
2.1. Source of heavy metals contamination
Natural abundances of these heavy metals have been on Earth’s surface (Citation20). It is now becoming increasingly evident that the use of heavy metals has increased rapidly, resulting in both terrestrial and aquatic environments having an unprecedented influx of metallic materials due to this increase in use (Citation21). There has been a significant increase in heavy metal pollution due to anthropogenic activity, which is primarily responsible for it (Citation22). This pollution is caused mainly by mining metal, smelting, foundry, and other metal-related industries. Some major sources of heavy metal ions are shown in .
Heavy metal ions in the sediment progressively leach into groundwater and surface water. Several industrial processes are responsible for heavy metal ion pollution in water sources. These industrial processes produce a large volume of wastewater containing heavy metal ions, which causes a variety of health effects in both animals and humans (Citation23). Metals are released from a variety of sources, including landfills, garbage dumps, urine, cattle and chicken dung, runoffs, automobiles, and road construction (Citation23). Heavy metal pollution has been a secondary cause of heavy metal pollution as a result of heavy metal use in agriculture, which includes herbicides, insecticides, fertilizers, and other chemicals (Citation24). Volcanic activity and natural mechanisms such as metal corrosion, evaporation of metals from soil and water, sediment re-suspension, soil erosion, geological weathering, and subsurface erosion all contribute to heavy metal pollution. Heavy metal contamination is mostly caused by volcanic activity (Citation25).
2.2. Heavy metals mediated toxicity
Heavy metal ions enter the human body through four main routes: eating or drinking contaminated heavy metal-contaminated food, drinking contaminated heavy metal-contaminated water, transmitting heavy metals through the skin, or inhaling contaminated heavy metal-contaminated air (Citation26). depicts the harmful consequences of heavy metal ions in the human body.
The combination of heavy metal ions is the most prevalent process by which covalent bonds are generated, and it is responsible for the hazardous character of metalloid compounds. Because heavy metals may form covalent bonds with organic groups, a lipophilic molecule or ion can be incorporated as a result of the covalent link (Citation27). Because of the lipophilic character of these metallic compounds, it is very simple for them to pass through the cell membrane and reach the intracellular region. A toxic effect is produced when these metallic compounds come in contact with the organelles inside a cell due to their contact (Citation28). Several significant heavy metals pose health risks. provides information about the permissible limits of heavy metals in drinking water as per the World Health Organization (WHO) and heavy metals toxicity.
Table 1. Permissible limits of heavy metal ions in drinking water and toxicity mechanism.
2.2.1. Heavy metals binding to the thiol group
When heavy metals enter the cell, they form chemical bonds with proteins, nucleic acids, and lipids, whereby the strongest bonds are formed between lipids and proteins. Enzymes and proteins undergo bonding with metals to generate thiol (-SH) groups on cysteine residues. This can lead to a disturbance in the equilibrium of redox within the cell due to the inhibition of protein functionality (Citation30). Consequently, impairment of the antioxidant defence system can result in damage to hepatocytes. It is worth emphasizing that heavy metals can elicit similar reactions in proteins. Amino acids that possess a –SH functional group play a role in the intricate process of bonding between heavy metals and proteins containing thiol groups (Citation33–35,Citation37).
2.2.2. Heavy metals mediated mutagenicity
It has been noted that heavy metals can cause site-specific damage to DNA by concurrently interacting with nuclear proteins. There is the risk of ‘direct’ injury and ‘indirect’ damage being inflicted. The presence of heavy metals may produce conformational changes in the biomolecules that have been subjected to ‘direct’ damage. Conversely, heavy metals cause ‘indirect’ harm by creating reactive oxygen and nitrogen species (Citation38). These species include endogenous oxidants such as nitric oxide, hydrogen peroxide, and hydroxyl and superoxide radicals. This damage is caused by producing reactive oxygen species (ROS) and nitrogen species. It has been demonstrated that exposure to heavy metals can activate signaling pathways (Citation27). Damage to DNA, disruption of sulphydryl equilibrium, and lipid peroxidation can all be caused by the creation of free radicals, which are triggered by the toxicity of heavy metals. Because of the damage to the membrane, changes have also been seen in the metal-mediated calcium homeostasis. This is related to the fact that membrane damage activates various calcium-dependent processes, including endonucleases. Iron, copper, nickel, chromium, and cadmium have received most of the research on free radical formation (Citation27). The Cr (VI) mediated mutagenicity is described in .
Mutagenicity of DNA base changes caused by metal-mediated free radicals provides evidence of a connection between oxidative damage and cancer development. The formation of free radicals results in a range of DNA base changes, most mutagenic (Citation39). This demonstrates the essential connection between the oxidative damage from metals and the carcinogenic potential of the metals themselves. Cd (II), Ni (II), and As (III) are three examples of metal ions that are known to hinder the DNA repair pathways (Citation40). The oxidative processes in DNA are characterized by the following: (i) base modification, which can be observed by Cr (VI) and Ni (II); (ii) cross-linking, which can be observed by Ni (II), Cu (II), Fe (II); (iii) strand scission, which can be observed by nickels, copper, chromiums and oxidants; and (iv) depurination, which can be observed by copper, chromium, and nickel (Citation27,Citation41).
3. Microbial mechanisms of heavy metals removal
Microbial-mediated heavy metal ions removal is generally described as a metabolically dependent process. Living microorganisms/plants are involved in the metabolically dependent process for the removal of heavy metals removal.
3.1. Bioaccumulation
According to Diep et al. (Citation42) and Deng et al. (Citation43), bioaccumulation is the accumulation of harmful metal ions within the intracellular space of an organism. This process is slower and more complicated than biosorption since it involves many metabolic pathways (Citation44). According to Timkova et al. (Citation45), bioaccumulation is a metabolic-dependent process that relies on energy availability and is carried out by living cells (Citation46). Ali et al. (Citation47) found that the bioaccumulation process was quite sensitive to the experimental circumstances. According to Yilmazer and Saracoglu (Citation2), the contaminants present in the medium might assemble on the surface of the organisms, which influences bioaccumulation. There have been several species of fungus, bacteria and microalgae, all of which have been shown to play key roles in removing heavy metals (Citation2).
Jacob et al (Citation48) found that among these biological systems, bacteria have an extraordinary resilience to heavy metals and can bio-accumulate within their internal space via the cell surface receptors on their surfaces. The heavy metal ions remediation by bacteria and their intracellular mechanisms are shown in .
Bacterial species that are very resistant to heavy metals have been discovered, including Pseudomonas (Citation49), Klebsiella (Citation50), Microbacterium (Citation51), and Bacillus (Citation52). In addition, bioremediation facilitated by bacteria is regarded as a method that is economical and kind to the environment (Citation53). According to research by Liu et al. (Citation54), bacterial strains that may be identified in environments polluted with heavy metals typically exhibit particular heavy metal resistance. Researchers have successfully isolated microbacterium species from areas polluted with heavy metals. According to the findings of Henson et al. (Citation55), Microbacterium sp. (Cr-K29) is capable of eliminating up to 88% of Cr (VI). Pattanapipitpaisal et al. (Citation56) isolated Cr (VI) reducing Microbacterium liquefaciens from a polluted site and assessed the organism’s capacity for bioremediation. The researchers found that Microbacterium liquefaciens could remove 81% of Cr (VI) from water that had been polluted. Bacteria utilize various routes to remove heavy metals, such as using heavy metals as an electron acceptor or producing soluble enzymes to detoxify the heavy metal (Citation57). When microbial cells are subjected to harmful heavy metals, ROS are produced. ROS may either cause damage to the cell’s organelles or interfere with many metabolic operations, both of which can disrupt the regular functioning of the cell (Citation58,Citation59). According to Joutey et al. (Citation60), the antioxidant activity of bacterial cells actively participates in the detoxification and bioremediation of Cr (VI). The microbial species capable of eliminating heavy metals are listed in .
Table 2. Cr (VI) removal efficiency of microorganisms.
Table 3. Pb (II) removal efficiency of microorganisms.
Table 4. Cd (II) removal efficiency of microorganisms.
Table 5. As (III/V) removal efficiency of microorganisms.
Moreover, fungi can quickly adapt to their natural surroundings and degrade organic and inorganic matter (Citation87,Citation88). They can be grown in highly hostile environments with high pH, temperature, and salt concentration (Citation89). These properties of the fungus make them suitable for extracting heavy metal ions from polluted sites (Citation81).
According to Zheng et al (Citation90), Pleurotus sp. is an effective method for the remediation of polluted water and soil. In addition to this, the Pleurotus species is distinguished by its exceptional ability to be grown without difficulty on a wide variety of solid substrates (biomass), even when subjected to harsh environmental conditions. They have several therapeutic uses, such as immune-stimulatory, anti-inflammatory, antioxidant, and anti-cancerous (Citation91–93). P. florida species also produce extracellular enzymes such as lignin peroxidase, cellulase, and laccase, which break down agricultural waste and plant residues containing cellulose, hemicelluloses, and lignin (Citation94,Citation95). The fungal cell digests these degraded components through its enzymatic system. Despite its complexity and heterogeneity, this enzymatic system is non-specific. It can degrade toxic compounds, including polycyclic aromatics, polychlorinated biphenyls, and dioxins. (Citation96–101).
3.2. Bioleaching
In the field of environmental remediation, bioleaching is regarded as one of the most intricate and diverse processes now in use. It involves a wide variety of microbial species, with a concentration of acidophiles. The capacity of these chemolithotrophic bacteria to oxidize Fe (II) to Fe (III) is a noteworthy feature. To develop in low pH settings (usually below 2.0), they reduce sulphur to sulfuric acid, which allows them to thrive in growth-promoting circumstances (Citation102,Citation103). Sulfuric acid is thought to create protons and ferric ions that aid in the solubilization of metals from ores, allowing metals in solids to be separated from water-soluble phases and eventually recovered (Citation104). The capacity of microorganisms to convert solid compounds into soluble substances that can be removed and recovered has a significant impact on recovery effectiveness. In light of this, the use of bioremediation to recover raw materials from wastewater has been advocated. 98.7% of Cd (II) was recovered using 0.1 M HCl and an absorbent developed from Annona squamosa (Citation104,Citation105). When combined with a highly concentrated solution of hydrochloric acid (0.1 M HCl), Pseudomonas aeruginosa biomass was found to be very effective in retrieving cadmium (II), with recovery rates reaching an impressive 82% (Citation106). After being immobilized for many weeks in a volcanic rock matrix at a pH of 2.35, P. putida cells demonstrated an astounding 100% recovery rate for the extraction of Cu for products that had been synthesized with cyanobacterial metallothioneins on their surfaces. Similarly, it has been reported that 100% recovery of copper (II) has been obtained in activated sludge at a pH level of 1.0 (Citation107). The inclusion of Enterobacter sp. J1 led to a notable increase in copper and lead ion recovery, which approached 90% at a pH of 2. This is a very important finding (Citation108–110).
3.3. Biotransformation
It has been demonstrated that a molecule’s biotransformation is a change in its chemical structure that eventually releases a molecule that is relatively more polar than the original component (Citation111). On the other hand, hazardous metals are changed into less hazardous heavy metal ions by the interaction of metals with microbes (Citation112). Micro-transformations may be produced by bacteria through a variety of processes, such as the production of carbon bonds, isomerization, the introduction of functional groups, oxidation, reduction, condensation, hydrolysis, methylation, and demethylation (Citation113). According to reports, microorganisms may change metals by making use of the resources they offer. The most toxic As (III) has been reported to be oxidized extremely efficiently by Micrococcus sp. and Acinetobacter sp., as these organisms can rapidly convert it into a compound called arsenic acid or arsenates, which are less soluble and less toxic than As (III) (Citation114,Citation115).
Furthermore, both aerobic and anaerobic bacteria are capable of facilitating the microbially mediated Cr (VI) transition. Certain vitamins and proteins, such as glutathione, are needed as electron donors in the anaerobic environment for the Cr (VI) reduction process. Through the process of biotransformation, poisonous Cr (VI) is transformed into less hazardous Cr (III). NADH and NADPH are necessary for biotransformation and serve as cofactors for the enzyme chromate reductase in the anaerobic reduction of Cr (VI). Cr (VI) ions eventually transform into stable oxidation states and less hazardous Cr (III) after converting into fewer oxidation stages like Cr (V) and (IV) (Citation116). Soluble deadly Cr (VI) ions become less poisonous insoluble Cr (III) ions throughout the aerobic process. Soluble chromate reductase is the enzyme responsible for this aerobic reduction of Cr (VI) (Citation117). The anaerobic and aerobic reduction of Cr (VI) ions into Cr (III) is shown in .
Chromate ions function as terminal electron acceptors in the respiratory system of bacteria, acting at the bacterial membrane, when oxygen is not present. On the other hand, the chromate reductase enzyme reduces the Cr (VI) ions into the lower oxidation state species like Cr(III) in an oxygenated environment. ROS also generated as intermediate products during the reduction process. Additionally, some research has revealed that cytochrome-b actively contributes to the anaerobic conditions in which electron transfer takes place (Citation118,Citation119). Numerous investigations have indicated that the process of extracellular electron transfer is associated with processes based on the cytochrome electron shuttle (Citation120,Citation121).
3.4. Rhizoremediation
The rhizoremediation process is employed to remove heavy metal ions from contaminated soil. In this process, microorganisms associate with plant roots and perform a heavy metals removal process. This method is an association of phytoremediation and bioaccumulation, involving two biological processes (Citation122). Organic pollutants and heavy metal contaminants are plaguing our environment. Rhizoremediation addresses this issue through a highly innovative and cutting-edge approach (Citation123). As part of the restoration process, this method uses plants and rhizospheric microorganisms to restore polluted environments because it can be highly effective when combined with plants and rhizospheric microorganisms. Rhizoremediation is one of the most effective methods of dealing with pollution, as it offers a combination of eco-friendliness, cost-effectiveness, and high efficiency for resolving the problem of contamination (Citation123). For rhizoremediation to be successful at a given site, several factors must be considered, including the level of contamination in the soil, the amount of metal contaminants present therein, and the plant’s ability to absorb metals aggressively. Rhizoremediation must, therefore, be carefully considered and taken into account as a means to ensure its effectiveness (Citation124). To safeguard our environment for future generations, we should continue exploring and developing rhizoremediation as a crucial step forward in the fight against pollution (Citation125).
The hyperaccumulators and non-hyperaccumulators can be classified as plants employed in the rhizoremediation process. Heavy metal-accumulating plants comprise the first group despite low biomass productivity (Citation126). However, using the latter extraction method, the capacity to extract heavy metals is somewhat lower than that of hyperaccumulators. However, their overall biomass yield is significantly higher than that of hyperaccumulators. Moreover, non-hyperaccumulator plants show rapid growth patterns (Citation127). The heavy metals removal from contaminated sites by plants is shown in .
Figure 6. Heavy metals uptake mechanisms by plants and microbes (Citation127).
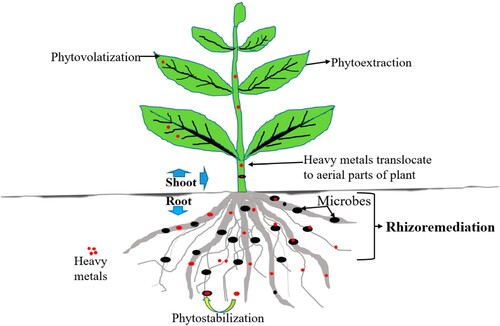
Some plant species benefit from rhizoremediation because they can extract heavy metals into their roots, tolerate various metals, and adapt to various environments (Citation127). A study by Eid et al. (Citation101) revealed that aquatic hydrophytes such as E. crassipes, E. stagnina, L. stolonifera, and P. australis possess the capability to accumulate Cd (II), Ni (II), and Pb (II) and can be utilized to indicate and remediate heavy metal pollution in wetlands. According to the findings, Phragmites australis can accumulate Cd (II) and Ni (II). In contrast, Echinochloa stagnina can get a high Pb (II) level in its tissues. However, except for Ni (II) in Echinochloa stagnina and Cd (II) in Ludwigia stolonifera, these species are suitable for phytoextraction and phytostabilization of heavy metals. This suggests that phytoremediation is a scientifically proven and eco-friendly technique for eliminating heavy metals from contaminated sites (Citation101,Citation127).
By phytostabilization, heavy metals are immobilized in the soil after adsorption or precipitation by the plant, making them less corrosive. Phytoextraction refers to transferring heavy metal ions from soil into plants (roots, stems, leaves) to accumulate the metal in them. Plant roots absorb heavy metals and push them up through xylem streams in phytovolatilization. Plants release heavy metal ions into the atmosphere by transferring them to their upper parts, such as leaves and stems (Citation128,Citation129). A comprehensive method for soil renewal has been developed by integrating this scientific approach with several other techniques. In the current state of the art, an evaluation is being done on how reliable and environmentally acceptable it would be to apply plants and plant growth-promoting bacteria (PGPBs) to soil to renew its health and fertility (Citation130). Heavy metals are absorbed or PGPB greatly facilitates plants’ adsorption in the soil close to their roots. PGPB also stimulates plant growth through the secretion of hormones and metabolites and its remediation capabilities (Citation130).
4. Microbial resistance to heavy metal ions
Heavy metals can induce toxicity in microorganisms. As a result, they can either cause the microorganism to die or thrive when they evolve mechanisms to deal with the toxicity of the metals. A potent bioremediation agent can be selected based on the resistance of microorganisms to the toxic effects of metals that can be used in the bioremediation of heavy metals. As a result of the development of the extracellular barrier and intracellular sequestration mechanisms, as well as the active transport of metal ions and enzymes for detoxifying metal ions (Citation131). The passage of heavy metals into the bacteria cells is prevented by the presence of barriers such as cell walls, plasma membranes, EPS, and biofilms located at the surface of the bacteria. In addition to their ability to adsorb metal ions. The surfaces of microbial cells also play an essential role in preventing metal ions from entering the bacterium by providing them with a barrier and preventing their entry (Citation132). Some fungi and bacteria isolates can be shown to be capable of absorbing heavy metals such as Cu (II), Pb (II), and Cr (VI) from the environment via biosorption and prevent entry of heavy metals into the cell (Citation133,Citation134). A biofilm is a layer of extracellular polymers produced by microbes that can accumulate metal ions and thus serve as a protective shield for the cells within them. According to Teitzel and Parsek (Citation135), the biofilm of Pseudomonas aeruginosa has been able to tolerate Pb (II) ions, Zn (II) ions, and Cu (II) ions. A biofilm of Rhodotorula mucilaginosa increased the efficiency of removing metal from 91.71% to 95.35% (Citation136). There is also evidence that EPS is an effective barrier against heavy metals other than biofilms and cell walls. For instance, the biosorption of Pb (II) ions in P. aeruginosa, Acinetobacter junii L Pb1, and Azotobacter chroococcum XU1 has been reported (Citation137). It is found that the cell membrane of microbes contains a wide variety of proteins and metabolic products that are capable of chelating metal ions (complex structure). As a result of extracellular sequestration, metal ions become insoluble compounds, or they accumulate in the periplasm of a cell. Among the copper-resistant Pseudomonas syringae strains, copper-inducible proteins (PolA, CopB, and CopC) are produced. They are responsible for binding copper ions and microbial colonies during copper induction. Copper-inducible proteins, therefore, are responsible for preventing infection from other microorganisms and bacteria. Using an efflux mechanism, the Synechocystis PCC 6803 strain can pass Zn (II) ions from its cytoplasm into its periplasm (Citation113). Desulfuromonas spp., Geobacter spp., and other iron- and sulfur-reducing bacteria can be used to reduce harmful metals such as Pb (II), Hg (II), Li (II) into less or non-hazardous metal ions. It has been proven that an obligate anaerobe known as G metallireducens is capable of reducing toxic Mg (II) to less toxic Mg (II) as well as toxic uranium (U) to deadly uranium (III) (IV) (Citation138). Metal ions can be taken up by different compounds within the cytoplasm of the cell in the process of intracellular sequestration. Heavy metals can accumulate in the cells of microorganisms due to their interaction with ligands present on the surface and slow transport into the cells. It has been found that bacteria are capable of accumulating heavy metals intracellularly in a wide range of applications, especially waste treatment. Cu (II), Cd (II), and Zn (II) ions were sequestered intracellularly by Cd(II)-tolerant Pseudomonas putida strains that contained cysteine-rich low molecular weight proteins (Citation139). Cd (II) ion sequestration by glutathione in Rhizobium leguminosarum cells has also been demonstrated (Citation140). Numerous fungi accumulate metals in their mycelium and spores, which assists in decontaminating metal ions by taking up energy, precipitating in the intracellular space, and transferring valences to other metals. The second strategy to manage intracellular heavy metal ion concentrations is via efflux mechanisms, which can effectively regulate intracellular heavy metal ion concentration in response to heavy metal stress (Citation141).
In the respiratory system of bacteria, chromate ions act at the bacterial membrane as terminal electron acceptors in the absence of oxygen. Conversely, in an oxygenated environment, the chromate reductase enzyme reduces Cr (VI) ions into the lower oxidation state [Cr(III)]. During the reduction process, ROS were also produced as intermediate products. Furthermore, several studies have shown that cytochrome-b actively supports the anaerobic environments necessary for electron transport (Citation118,Citation119). Several studies have shown that mechanisms based on the cytochrome electron shuttle are linked to the extracellular electron transfer process (Citation142).
Because of the enzymes that convert the highly toxic forms of heavy metal ions into less toxic forms when they contact with microorganisms, heavy metal ions are extremely poisonous to bacteria (Citation143). Lowering or oxidizing heavy metal ions can efficiently alter their redox state, hence lowering their toxicity (Citation144). Detoxification enzymes have an impact on microbial resistance genes, which regulate these defensive systems. Through the mercuric ion reductase enzyme, Bacillus species exhibit tolerance to mercury ions (Citation145). Mercuric reductase transforms the mercuric ion into metallic mercury through the membrane (Citation146). Acinetobacter sp. and Micrococcus sp. can oxidize hazardous ingredients such as acetic acid, acetate, and sulfur dioxide (III) into less soluble and non-toxic materials such as acetone (V) (Citation121).
5. Factors influencing microbial remediation of heavy metals
Heavy metals tend to either stimulate or inhibit microorganisms depending on their chemical forms, concentrations of metal ions, and other factors such as redox potential, which determine the extent to which they are toxic to microorganisms (Citation113). Several environmental factors can affect a heavy metal’s change, transport, valence state, and bioavailability to microorganisms after the heavy metal is transformed. These factors include temperature, pH, low molecular weight organic acids, and humic acids (Citation147). There is a clear correlation between temperature and the remediation of heavy metals. As the temperature increases, the solubility of heavy metals increases, which means that heavy metals are more likely to be bioavailable as the temperature rises. In addition, microorganisms will be able to perform more efficiently using an appropriate range of temperatures, which will enable their metabolisms and enzyme activities to increase, thereby accelerating bioremediation. pH is another important parameter for the growth of organisms and bioremediation of heavy metal ions (Citation113,Citation125). Therefore, its suitable pH range is required for microbial growth for better remediation efficiency. Moreover, microbial remediation of heavy metal ions also depends on the type of microbial species, concentration of heavy metal ions in the medium, toxicity of heavy metal ions, the chemical composition of the substrate, supply of oxygen, agitation rate and incubation time (Citation148).
6. Advantages and disadvantages of microbial remediation of heavy metals
The microbial remediation of heavy metals is classified into several categories such as bioaccumulation, bio-conversion/ bio-transformation and bioleaching and rhizoremediation. These microbial remediation methods are less toxic and eco-friendly (Citation113). The advantages and disadvantages of some important heavy metal removal methods including microbial methods are discussed in .
Table 6. The advantages and limitations of the heavy metal removal method.
7. Technology challenges and future prospects
There has been an increased incidence of heavy metal contamination in aquatic environments, as well as eco-friendly methods of detoxifying them from contaminated water. As a potential method for heavy metal removal, microbial detoxification represents a very promising technique, since it has a high capacity to remove heavy metals while also being eco-friendly, although some areas still require improvement. As a result of a critical review of the literature, it appears that the following areas of research need to be further investigated, as follows:
A primary concern is the successful implementation of bioremediation techniques at a commercial scale, particularly in water treatment plants.
Several strategies can be employed to improve the removal of heavy metals from aqueous media, such as manipulating bacterial strain immobilization onto solid substrates and utilizing composite biosorbents or biopolymers.
Economic analysis of microbial-based heavy metals detoxification (including media costs, energy consumption, labor, etc.) should be conducted. It is rare for research to provide a simple cost assessment of the materials used for heavy metals remediation.
8. Conclusion
The heavy metal ions are released into the environment through anthropogenic and natural processes and cause water, soil and air contamination. Exposure to heavy metal ions in humans and animals causes several harmful health impacts such as kidney and liver damage, skin problems, mental dullness, reproductive disorders, cardiovascular illness and cancers. Various conventional approaches are employed to eliminate heavy metal ions. However, these conventional methods have several disadvantages. Hence, eco-friendly and cost-effective biological methods for heavy metal ions must be developed to replace the dependency on conventional methods. In this review, we have discussed the microbial-mediated heavy metals removal. Microorganisms are highly resistant to the heavy metal ions mediated stress and can be considered suitable bioremediating agents. Microbial-mediated heavy metal ion remediation offers an excellent alternative to conventional methods and contributes to a potential role in wastewater treatment.
Author contributions
Conceptualization, G.P. and Q.Y.; validation, G.P. and Q.Y.; formal analysis, G.P. and Q.Y.; investigation, G.P. and Q.Y.; resources, G.P. and Q.Y.; writing – original draft preparation, G.P. and Q.Y.; writing – review and editing, Q.Y.; visualization, G.P. and Q.Y.; supervision, Q.Y. All authors have read and agreed to the published version of the manuscript.
Acknowledgements:
The authors are thankful to the Academy of Forestry Science, China, College of Life Science, Baicheng Normal University, Baicheng, 137000, China, and the Institute of Grassland Research, Chinese Academy of Agricultural Sciences, Hohhot, China, for providing necessary support for this research work.
Disclosure statement
No potential conflict of interest was reported by the author(s).
Data availability statement
Data mentioned in this manuscript.
Additional information
Funding
References
- Manisalidis, I.; Stavropoulou, E.; Stavropoulos, A.; Bezirtzoglou, E. Environmental and Health Impacts of Air Pollution: A Review. Front. Public. Health. 2020, 8. doi:10.3389/fpubh.2020.00014.
- Ali, H.; Khan, E.; Ilahi, I. Environmental Chemistry and Ecotoxicology of Hazardous Heavy Metals: Environmental Persistence, Toxicity, and Bioaccumulation. J. Chem. 2019, 1–14. doi:10.1155/2019/6730305.
- Herawati, S.; Suzuki, N.; Hayashi, K.; Rivai, I.F. Cadmium, Copper, and Zinc Levels in Rice and Soil of Japan, Indonesia, and China by Soil Type. Bull. Environ. Contam. Toxicol. 2000, 64 (1), 33–39. doi:10.1007/s001289910006.
- Wu, X.; Cobbina, S.J.; Mao, G.; Xu, H.; Zhang, Z.; Yang, L. A Review of Toxicity and Mechanisms of Individual and Mixtures of Heavy Metals in the Environment. Environ. Sci. Pollut. Res. 2016, 23 (9), 8244–8259. doi:10.1007/s11356-016-6333-x.
- Briffa, J.; Sinagra, E.; Blundell, R. Heavy Metal Pollution in the Environment and Their Toxicological Effects on Humans. Heliyon 2020, 6 (9), e04691. doi:10.1016/j.heliyon.2020.e04691.
- Hamdzah, M.; Ujang, Z.; Nasef, M.M.; Abdullah, N.; Dahalan, F.A. Removal of Ni(II). Zn(II) and Pb(II) from Aqueous Solutions Using Cation-Exchange Resin in Fixed-Bed Column. Desalin. Water Treat. 2016, 57 (40), 18770–18781. doi:10.1080/19443994.2015.1095118.
- Health Effects of Lead Exposure | Lead | CDC. https://www.atsdr.cdc.gov/csem/leadtoxicity/signs_and_symptoms.html
- Olufemi, A.C.; Mji, A.; Mukhola, M.S. Potential Health Risks of Lead Exposure from Early Life Through Later Life: Implications for Public Health Education. Int. J. Environ. Res. Public Health. 2022, 19 (23), 16006. doi:10.3390/ijerph192316006.
- Bernhoft, R.A. Cadmium Toxicity and Treatment. Scientific World J. 2013, 1–7. doi:10.1155/2013/394652.
- Balali-Mood, M.; Naseri, K.; Tahergorabi, Z.; Khazdair, M.R.; Sadeghi, M. Toxic Mechanisms of Five Heavy Metals: Mercury, Lead, Chromium, Cadmium, and Arsenic. Front. Pharmacol. 2021, 12. doi:10.3389/fphar.2021.643972.
- Singh Sankhla, M. Arsenic-Induced Neurotoxic & Carcinogenic Effects on Humans. Open Access J. Toxicol. 2018, 3 (4). doi:10.19080/OAJT.2018.03.555617.
- Qasem, N.A.A.; Mohammed, R.H.; Lawal, D.U. Removal of Heavy Metal Ions from Wastewater: A Comprehensive and Critical Review. Npj Clean Water 2021, 4 (1). doi:10.1038/s41545-021-00127-0.
- Sun, Y.; Wang, H.; Long, X.; Xi, H.; Biao, P.; Yang, W. Advance in Remediated of Heavy Metals by Soil Microbial Fuel Cells: Mechanism and Application. Front. Microbiol. 2022, 13. doi:10.3389/fmicb.2022.997732.
- Jin, R.; Liu, Y.; Liu, G.; Tian, T.; Qiao, S.; Zhou, J. Characterization of Product and Potential Mechanism of Cr(VI) Reduction by Anaerobic Activated Sludge in a Sequencing Batch Reactor. Sci. Rep. 2017, 7 (1). doi:10.1038/s41598-017-01885-z.
- Jobby, R.; Jha, P.; Yadav, A.K.; Desai, N. Biosorption and Biotransformation of Hexavalent Chromium [Cr(VI)]: A Comprehensive Review. Chemosphere 2018, 207, 255–266. doi:10.1016/j.chemosphere.2018.05.050.
- Ungureanu, E.L.; Mocanu, A.L.; Stroe, C.A.; Panciu, C.M.; Berca, L.; Sionel, R.M.; Mustatea, G. Agricultural Byproducts Used as Low-Cost Adsorbents for Removal of Potentially Toxic Elements from Wastewater: A Comprehensive Review. Sustainability 2023, 15 (7), 5999. doi:10.3390/su15075999.
- Youssef, A.M.; Abdel-Aziz, M.E.; El-Sayed, E.S.A.; Abdel-Aziz, M.S.; Abd El-Hakim, A.A.; Kamel, S.; Turky, G. Morphological, Electrical & Antibacterial Properties of Trilayered Cs/PAA/PPy Bionanocomposites Hydrogel Based on Fe3O4-NPs. Carbohydr. Polym. 2018, 196, 483–493. doi:10.1016/j.carbpol.2018.05.065.
- Singh, V.; Singh, N.; Rai, S.N.; Kumar, A.; Singh, A.K.; Singh, M.P.; Sahoo, A.; Shekhar, S.; Vamanu, E.; Mishra, V. Heavy Metal Contamination in the Aquatic Ecosystem: Toxicity and Its Remediation Using Eco-Friendly Approaches. Toxics 2023, 11 (2), 147. doi:10.3390/toxics11020147.
- Hama Aziz, K.H.; Mustafa, F.S.; Omer, K.M.; Hama, S.; Hamarawf, R.F.; Rahman, K.O. Heavy Metal Pollution in the Aquatic Environment: Efficient and Low-Cost Removal Approaches to Eliminate Their Toxicity: A Review. RSC Adv. 2023, 13 (26), 17595–17610. doi:10.1039/D3RA00723E.
- Chen, X.; Hossain, M.F.; Duan, C.; Lu, J.; Tsang, Y.F.; Islam, M.S.; Zhou, Y. Isotherm Models for Adsorption of Heavy Metals from Water – A Review. Chemosphere 2022, 307, 135545. doi:10.1016/j.chemosphere.2022.135545.
- Abou Seeda, M. A.; El-Sayed, A. A.; Yassen, A. A.; Zaghloul, S. M.; Khater, A.. . Heavy Metals, Sources, Chemistry, Risks and Best Applicable Approach for Remediationof Contaminated Soils: A Review. Middle East J. Appl. Sci 2019. doi:10.36632/mejas/2019.9.4.2.
- Ghugare, G.S.; Katkar, P.S. Heavy Metals in the Coal Mines Environment, Their Toxicity and Remediation. Int. J. Res.Biosci. Agric. Technol 2022. doi:10.29369/ijrbat.2022.010.3.0017.
- Yi, Y.; Yang, Z.; Zhang, S. Ecological Risk Assessment of Heavy Metals in Sediment and Human Health Risk Assessment of Heavy Metals in Fishes in the Middle and Lower Reaches of the Yangtze River Basin. Environ. Pollut. 2011, 159 (10), 2575–2585. doi:10.1016/j.envpol.2011.06.011.
- Tijani, M.N.; Jinno, K.; Hiroshiro, Y. Environmental Impact of Heavy Metals Distribution in Water and Sediments of Ogunpa River, Ibadan Area, Southwestern Nigeria. J. Min. Geol 2004, 40. doi:10.4314/jmg.v40i1.18811.
- Alengebawy, A.; Abdelkhalek, S.T.; Qureshi, S.R.; Wang, M.-Q. Heavy Metals and Pesticides Toxicity in Agricultural Soil and Plants: Ecological Risks and Human Health Implications. Toxics 2021, 9 (3), 42. doi:10.3390/toxics9030042.
- Yan, X.; Liu, M.; Zhong, J.; Guo, J.; Wu, W. How Human Activities Affect Heavy Metal Contamination of Soil and Sediment in a Long-Term Reclaimed Area of the Liaohe River Delta, North China. Sustainability 2018, 10 (2), 338. doi:10.3390/su10020338.
- Jaishankar, M.; Tseten, T.; Anbalagan, N.; Mathew, B.B.; Beeregowda, K.N. Toxicity, Mechanism and Health Effects of Some Heavy Metals. Interdiscip. Toxicol. 2014, 7 (2), 60–72. doi:10.2478/intox-2014-0009.
- Jan, A.; Azam, M.; Siddiqui, K.; Ali, A.; Choi, I.; Haq, Q. Heavy Metals and Human Health: Mechanistic Insight Into Toxicity and Counter Defense System of Antioxidants. Int. J. Mol. Sci. 2015, 16, 29592–29630. doi:10.3390/ijms161226183.
- Li, L.; Zhang, Y.; Ippolito, J.A.; Xing, W.; Qiu, K.; Yang, H. Lead Smelting Effects Heavy Metal Concentrations in Soils, Wheat, and Potentially Humans. Environ. Pollut. 2020, 257, 113641. doi:10.1016/j.envpol.2019.113641.
- Wise, J. P. Jr.; Young, J. L.; Cai, J.; Cai, L. Current understanding of hexavalent chromium [Cr(VI)] neurotoxicity and new perspectives. Environ. Int. 2022, 158 (1), 106877. doi:10.1016/j.envint.2021.106877.
- Honeycutt, M.E. Hexavalent Chromium in Texas Drinking Water. Toxicol. Sci. 2011, 119 (2), 423–424. doi:10.1093/toxsci/kfq347.
- Leyssens, L.; Vinck, B.; Van Der Straeten, C.; Wuyts, F.; Maes, L. Cobalt Toxicity in Humans – A Review of the Potential Sources and Systemic Health Effects. Toxicology 2017, 387, 43–56.
- Toxicological Profile for Nickel. https://www.atsdr.cdc.gov/toxprofiles/tp15.pdf. (Accessed on August 27, 2023).
- ATSDR Toxicological Profile for Copper. https://www.atsdr.cdc.gov/toxprofiles/tp132.pdf.
- Haynes, W. M.. Book Listing of CRC Handbook of Chemistry and Physics, 92nd ed., 2011–2012. J. Am. Chem. Soc. 2011, 133 (34), 13766. doi:10.1021/ja2071225.
- Singh, V.; Singh, M.P.; Mishra, V. Bioremediation of Toxic Metal Ions from Coal Washery Effluent. Desalin. Water Treat. 2020, 197, 300–318. doi:10.5004/dwt.2020.25996.
- Mason, L. H.; Harp, J. P.; Han, D. Y.. Pb Neurotoxicity: Neuropsychological Effects of Lead Toxicity. BioMed Res. Int. 2014, 840547. doi:10.1155/2014/840547.
- ATSDR Toxicological Profile for Lead. (2020) https://www.atsdr.cdc.gov/toxprofiles/tp13.pdf
- Valko, M.; Morris, H.; Cronin, M. Metals, Toxicity and Oxidative Stress. Curr. Med. Chem. 2005, 12 (10), 1161–1208. doi:10.2174/0929867053764635.
- Kumari, S.; Sharma, S.; Advani, D.; Khosla, A.; Kumar, P.; Ambasta, R.K. Unboxing the Molecular Modalities of Mutagens in Cancer. Environ. Sci. Poll. Res 2022, 29 (41), 62111–62159. doi:10.1007/s11356-021-16726-w.
- Koedrith, P.; Seo, Y.R. Advances in Carcinogenic Metal Toxicity and Potential Molecular Markers. Int. J. Mol. Sci. 2011, 12, 9576–9595. doi:10.3390/ijms12129576.
- Martemucci, G.; Costagliola, C.; Mariano, M.; D’andrea, L.; Napolitano, P.; D’Alessandro, A.G. Free Radical Properties, Source and Targets, Antioxidant Consumption and Health. Oxygen 2022, 2 (2), 48–78. doi:10.3390/oxygen2020006.
- Diep, P.; Mahadevan, R.; Yakunin, A.F. Heavy Metal Removal by Bioaccumulation Using Genetically Engineered Microorganisms. Front. Bioeng. Biotechnol. 2018, 6. doi:10.3389/fbioe.2018.00157.
- Deng, X.; Yi, X.E.; Liu, G. Cadmium Removal from Aqueous Solution by Gene-Modified Escherichia Coli JM109. J. Hazard. Mater. 2007, 139 (2), 340–344. doi:10.1016/j.jhazmat.2006.06.043.
- Zhang, H.; Tan, X.; Qiu, T.; Zhou, L.; Li, R.; Deng, Z. A Novel and Biocompatible Fe3O4 Loaded Chitosan Polyelectrolyte Nanoparticles for the Removal of Cd2+ Ion. Int. J. Biol. Macromol. 2019, 141, 1165–1174. doi:10.1016/j.ijbiomac.2019.09.040.
- Timková, I.; Sedláková-Kaduková, J.; Pristaš, P. Biosorption and Bioaccumulation Abilities of Actinomycetes/Streptomycetes Isolated from Metal Contaminated Sites. Separations 2018, 5 (4), 54. doi:10.3390/separations5040054.
- Arishi, A.; Mashhour, I. Microbial Mechanisms for Remediation of Hexavalent Chromium and Their Large-Scale Applications; Current Research and Future Directions. J Pure Appl. Microbiol 2021, 15 (1), 53–67. doi:10.22207/JPAM.15.1.32.
- Yilmazer, P.; Saracoglu, N. Bioaccumulation and Biosorption of Copper(II) and Chromium(III) from Aqueous Solutions byPichia Stipitisyeast. J. Chem. Technol. Biotechnol. 2009, 84 (4), 604–610. doi:10.1002/jctb.2088.
- Jacob, J.M.; Karthik, C.; Saratale, R.G.; Kumar, S.S.; Prabakar, D.; Kadirvelu, K.; Pugazhendhi, A. Biological Approaches to Tackle Heavy Metal Pollution: A Survey of Literature. J. Environ. Manag. 2018, 217, 56–70. doi:10.1016/j.jenvman.2018.03.077.
- El-Naggar, N.E.-A.; El-khateeb, A.Y.; Ghoniem, A.A.; El-Hersh, M.S.; Saber, W.I.A. Innovative low-Cost Biosorption Process of Cr6+ by Pseudomonas Alcaliphila NEWG-2. Sci. Rep. 2020, 10 (1). doi:10.1038/s41598-020-70473-5.
- Tekerlekopoulou, A.G.; Tsiflikiotou, M.; Akritidou, L.; Viennas, A.; Tsiamis, G.; Pavlou, S.; Bourtzis, K.; Vayenas, D.V. Modelling of Biological Cr(VI) Removal in Draw-Fill Reactors Using Microorganisms in Suspended and Attached Growth Systems. Water Res. 2013, 47 (2), 623–636. doi:10.1016/j.watres.2012.10.034.
- Humphries, A.C.; Nott, K.P.; Hall, L.D.; Macaskie, L.E. Reduction of Cr(VI) by Immobilized Cells of Desulfovibrio Vulgaris NCIMB 8303 and Microbacterium Sp. NCIMB 13776. Biotechnol. Bioeng. 2005, 90 (5), 589–596. doi:10.1002/bit.20450.
- Li, R.; Hu, D.; Hu, K.; Deng, H.; Zhang, M.; Wang, A.; Qiu, R.; Yan, K. Coupling Adsorption-Photocatalytic Reduction of Cr(VI) by Metal-Free N-Doped Carbon. Sci. Total Environ. 2020, 704, 135284. doi:10.1016/j.scitotenv.2019.135284.
- Ibrahim, A.S.S.; El-Tayeb, M.A.; Elbadawi, Y.B.; Al-Salamah, A.A.; Antranikian, G. Hexavalent Chromate Reduction by Alkaliphilic Amphibacillus Sp. KSUCr3 Is Mediated by Copper-Dependent Membrane-Associated Cr(VI) Reductase. Extremophiles 2012, 16 (4), 659–668. doi:10.1007/s00792-012-0464-x.
- Liu, Z.; Wu, Y.; Lei, C.; Liu, P.; Gao, M. Chromate Reduction by a Chromate-Resistant Bacterium, Microbacterium Sp. World J. Microbiol. Biotechnol. 2012, 28 (4), 1585–1592. doi:10.1007/s11274-011-0962-5.
- Henson, M.W.; Santo Domingo, J.W.; Kourtev, P.S.; Jensen, R.V.; Dunn, J.A.; Learman, D.R. Metabolic and Genomic Analysis Elucidates Strain-Level Variation inMicrobacterium Spp.Isolated from Chromate Contaminated Sediment. PeerJ. 2015, 3, e1395. doi:10.7717/peerj.1395.
- Pattanapipitpaisal, P.; Brown, N.; Macaskie, L. Chromate Reduction and 16S rRNA Identification of Bacteria Isolated from a Cr(VI)-Contaminated Site. Appl. Microbiol. Biotechnol. 2001, 57 (1–2), 257–261. doi:10.1007/s002530100758.
- Ahemad, M. Enhancing Phytoremediation of Chromium-Stressed Soils Through Plant-Growth-Promoting Bacteria. J. Genet. Eng. Biotechnol. 2015, 13 (1), 51–58. doi:10.1016/j.jgeb.2015.02.001.
- Kubrak, O.I.; Lushchak, O.V.; Lushchak, J.V.; Torous, I.M.; Storey, J.M.; Storey, K.B.; Lushchak, V.I. Chromium Effects on Free Radical Processes in Goldfish Tissues: Comparison of Cr(III) and Cr(VI) Exposures on Oxidative Stress Markers, Glutathione Status and Antioxidant Enzymes. Comp. Biochem. Physiol. C: Toxicol. Pharmacol. 2010, 152 (3), 360–370. doi:10.1016/j.cbpc.2010.06.003.
- Kumar, M.S.; Praveenkumar, R.; Ilavarasi, A.; Rajeshwari, K.; Thajuddin, N. Biochemical Changes of Fresh Water Cyanobacteria Dolichospermum Flos-Aquae NTMS07 to Chromium-Induced Stress with Special Reference to Antioxidant Enzymes and Cellular Fatty Acids. Bull. Environ. Contam. Toxicol. 2013, 90 (6), 730–735. doi:10.1007/s00128-013-0984-9.
- Tahri Joutey, N.; Bahafid, W.; Sayel, H.; Maâtaoui, H.; Errachidi, F.; El Ghachtouli, N. Use of Experimental Factorial Design for Optimization of Hexavalent Chromium Removal by a Bacterial Consortium: Soil Microcosm Bioremediation. Soil Sediment Contam.: Int. J 2015, 24 (2), 129–142. doi:10.1080/15320383.2014.922931.
- Mary Mangaiyarkarasi, M.S.; Vincent, S.; Janarthanan, S.; Subba Rao, T.; Tata, B.V.R. Bioreduction of Cr(VI) by Alkaliphilic Bacillus Subtilis and Interaction of the Membrane Groups. Saudi J. Biol. Sci. 2011, 18 (2), 157–167. doi:10.1016/j.sjbs.2010.12.003.
- Karthik, C.; Barathi, S.; Pugazhendhi, A.; Ramkumar, V.S.; Thi, N.B.D.; Arulselvi, P.I. Evaluation of Cr(VI) Reduction Mechanism and Removal by Cellulosimicrobium Funkei Strain AR8, a Novel Haloalkaliphilic Bacterium. J. Hazard. Mater. 2017, 333, 42–53. doi:10.1016/j.jhazmat.2017.03.037.
- Zahoor, A.; Rehman, A. Isolation of Cr(VI) Reducing Bacteria from Industrial Effluents and Their Potential Use in Bioremediation of Chromium Containing Wastewater. J. Environ. Sci. 2009, 21, 814–820. doi:10.1016/S1001-0742(08)62346-3.
- Basu, S.; Dasgupta, M.; Chakraborty, B. Removal of Chromium (VI) by Bacillus Subtilis Isolated from East Calcutta Wetlands, West Bengal, India. Int. J. Biosci. Biochem. Bioinf 2014, 7–10. doi:10.7763/IJBBB.2014.V4.300.
- Srivastava, S.; Thakur, I.S. Evaluation of Biosorption Potency of Acinetobacter Sp. for Removal of Hexavalent Chromium from Tannery Effluent. Biodegradation 2007, 18 (5), 637–646. doi:10.1007/s10532-006-9096-0.
- Zhang, K.; Xue, Y.; Xu, H.; Yao, Y. Lead Removal by Phosphate Solubilizing Bacteria Isolated from Soil Through Biomineralization. Chemosphere 2019, 224, 272–279. doi:10.1016/j.chemosphere.2019.02.140.
- Bahavar, A.; Monavari, S.H.R.; Keyvani, H.; Esghaei, M.; Ghorbani, S.; Ataei-Pirkooh, A. Sero-Prevalence of Herpes Simplex and Epstein Barr Viruses in HIV Positive Patients in Tehran. Iran. J. Virol 2015, 9 (4), 29–34. doi:10.21859/isv.9.4.29.
- Cephidian, A.; Makhdoumi, A.; Mashreghi, M.; Mahmudy Gharaie, M.H. Removal of Anthropogenic Lead Pollutions by a Potent Bacillus Species AS2 Isolated from Geogenic Contaminated Site. Int. J. Environ. Sci. Technol. 2016, 13 (9), 2135–2142. doi:10.1007/s13762-016-1023-2.
- Ogbo, E.M.; Okhuoya, J.A. Bio-Absorption of Some Heavy Metals by Pleurotus Tuber-Regium Fr. Singer (An Edible Mushroom) from Crude Oil Polluted Soils Amended with Fertilizers and Cellulosic Wastes. Int. J. Soil Sci. 2010, 6 (1), 34–48. doi:10.3923/ijss.2011.34.48.
- Albert, Q.; Leleyter, L.; Lemoine, M.; Heutte, N.; Rioult, J.-P.; Sage, L.; Baraud, F.; Garon, D. Comparison of Tolerance and Biosorption of Three Trace Metals (Cd, Cu, Pb) by the Soil Fungus Absidia Cylindrospora. Chemosphere 2018, 196, 386–392. doi:10.1016/j.chemosphere.2017.12.156.
- Vaseem, H.; Singh, V.K.; Singh, M.P. Heavy Metal Pollution Due to Coal Washery Effluent and Its Decontamination Using a Macrofungus, Pleurotus Ostreatus. Ecotoxicol. Environ. Saf. 2017, 145, 42–49. doi:10.1016/j.ecoenv.2017.07.001.
- Shanab, S.; Essa, A.; Shalaby, E. Bioremoval Capacity of Three Heavy Metals by Some Microalgae Species (Egyptian Isolates). Plant. Signal. Behav. 2012, 7 (3), 392–399. doi:10.4161/psb.19173.
- Ye, J.; Xiao, H.; Xiao, B.; Xu, W.; Gao, L.; Lin, G. Bioremediation of Heavy Metal Contaminated Aqueous Solution by Using Red Algae Porphyra Leucosticta. Water Sci. Technol. 2015, 72 (9), 1662–1666. doi:10.2166/wst.2015.386.
- wang, Y.; Wang, B.; Peng, Q.; Chen, X.; Huang, Y. Adsorption of Cr(VI) by Iron Loaded Porous Carbon with High Cycling Properties. Mater. Lett. 2020, 274, 127988. doi:10.1016/j.matlet.2020.127988.
- Heidari, P.; Panico, A. Sorption Mechanism and Optimization Study for the Bioremediation of Pb(II) and Cd(II) Contamination by Two Novel Isolated Strains Q3 and Q5 of Bacillus Sp. Int. J. Environ. Res. Public Health 2020, 17 (11), 4059. doi:10.3390/ijerph17114059.
- Chen, H.; Zhong, C.; Berkhouse, H.; Zhang, Y.; Lv, Y.; Lu, W.; Yang, Y.; Zhou, J. Removal of Cadmium by Bioflocculant Produced by Stenotrophomonas Maltophilia Using Phenol-Containing Wastewater. Chemosphere 2016, 155, 163–169. doi:10.1016/j.chemosphere.2016.04.044.
- Choińska-Pulit, A.; Sobolczyk-Bednarek, J.; Łaba, W. Optimization of Copper, Lead and Cadmium Biosorption Onto Newly Isolated Bacterium Using a Box-Behnken Design. Ecotoxicol. Environ. Saf. 2018, 149, 275–283. doi:10.1016/j.ecoenv.2017.12.008.
- Abbas, S.Z.; Rafatullah, M.; Ismail, N.; Lalung, J. Isolation, Identification, Characterization, and Evaluation of Cadmium Removal Capacity ofEnterobacterspecies. J. Basic Microbiol. 2014, 54 (12), 1279–1287. doi:10.1002/jobm.201400157.
- Mitra, S.; Pramanik, K.; Sarkar, A.; Ghosh, P.K.; Soren, T.; Maiti, T.K. Bioaccumulation of Cadmium by Enterobacter Sp. and Enhancement of Rice Seedling Growth Under Cadmium Stress. Ecotoxicol. Environ. Saf. 2018, 156, 183–196. doi:10.1016/j.ecoenv.2018.03.001.
- El-Gendy, M.M.A.A.; Ten, N.M.; Ibrahim, H.A.E.-H.; Abd El-Baky, D.H. Heavy Metals Biosorption from Aqueous Solution by Endophytic Drechslera Hawaiiensis of Morus Alba L. Derived from Heavy Metals Habitats. Mycobiology 2017, 45 (2), 73–83. doi:10.5941/myco.2017.45.2.73.
- García, M.; Alonso, J.; Melgar, M. Agaricus Macrosporusas a Potential Bioremediation Agent for Substrates Contaminated with Heavy Metals. J. Chem. Technol. Biotechnol. 2005, 80 (3), 325–330. doi:10.1002/jctb.1203.
- Dey, U.; Chatterjee, S.; Mondal, N.K. Isolation and Characterization of Arsenic-Resistant Bacteria and Possible Application in Bioremediation. Biotechnol. Rep. 2016, 10, 1–7. doi:10.1016/j.btre.2016.02.002.
- Pandey, N.; Manjunath, K.; Sahu, K. Screening of Plant Growth Promoting Attributes and Arsenic Remediation Efficacy of Bacteria Isolated from Agricultural Soils of Chhattisgarh. Arch. Microbiol. 2020, 202 (3), 567–578. doi:10.1007/s00203-019-01773-2.
- Kepel, B.; Bodhi, W.; Tallei, T.E. Isolation and Identification of Arsenic-Resistant Bacteria for Possible Application in Arsenic Bioremediation. Pak. J. Biol. Sci. 2019, 23 (1), 63–67. doi:10.3923/pjbs.2020.63.67.
- Ghodsi, H. Investigation of Bioremediation of Arsenic by Bacteria Isolated from Contaminated Soil. Afr. J. Microbiol. Res. 2011, 5. doi:10.5897/ajmr11.837.
- Acioly, L.M.; Cavalcanti, D.; Luna, M.C.; Júnior, J.C.; Andrade, R.F.; e Silva, T.A.D.L.; La Rotta, C.E.; Campos-Takaki, G.M. Cadmium Removal from Aqueous Solutions by Strain of Pantoea Agglomerans UCP1320 Isolated from Laundry Effluent. Open. Microbiol. J. 2018, 12 (1), 297–307. doi:10.2174/1874285801812010297.
- Archana; Jaitly, A.K. Analysis of Trace Metals in Underground Drinking Water of Bareilly. Invertis J. Renewable Energy 2016, 6 (2), 106. doi:10.5958/2454-7611.2016.00014.x.
- Agnello, A.C.; Bagard, M.; van Hullebusch, E.D.; Esposito, G.; Huguenot, D. Comparative Bioremediation of Heavy Metals and Petroleum Hydrocarbons Co-Contaminated Soil by Natural Attenuation, Phytoremediation, Bioaugmentation and Bioaugmentation-Assisted Phytoremediation. Sci. Total Environ. 2016, 563–564, 693–703. doi:10.1016/j.scitotenv.2015.10.061.
- Zheng, C.; Zheng, H.; Sun, Y.; Xu, B.; Wang, Y.; Zheng, X.; Wang, Y. Simultaneous Adsorption and Reduction of Hexavalent Chromium on the Poly(4-Vinyl Pyridine) Decorated Magnetic Chitosan Biopolymer in Aqueous Solution. Bioresour. Technol. 2019, 293, 122038. doi:10.1016/j.biortech.2019.122038.
- Synytsya, A.; Míčková, K.; Synytsya, A.; Jablonský, I.; Spěváček, J.; Erban, V.; Kováříková, E.; Čopíková, J. Glucans from Fruit Bodies of Cultivated Mushrooms Pleurotus Ostreatus and Pleurotus Eryngii: Structure and Potential Prebiotic Activity. Carbohydr. Polym. 2009, 76 (4), 548–556. doi:10.1016/j.carbpol.2008.11.021.
- Barros, L.; Baptista, P.; Estevinho, L.; Ferreira, I. Bioactive Properties of the Medicinal Mushroom Leucopaxillus Giganteus Mycelium Obtained in the Presence of Different Nitrogen Sources. Food Chem. 2007, 105 (1), 179–186. doi:10.1016/j.foodchem.2007.03.063.
- Gadd, G.M. Geomycology: Biogeochemical Transformations of Rocks, Minerals, Metals and Radionuclides by Fungi, Bioweathering and Bioremediation. Mycol. Res. 2007, 111 (1), 3–49. doi:10.1016/j.mycres.2006.12.001.
- Kumla, J.; Suwannarach, N.; Sujarit, K.; Penkhrue, W.; Kakumyan, P.; Jatuwong, K.; Vadthanarat, S.; Lumyong, S. Cultivation of Mushrooms and Their Lignocellulolytic Enzyme Production Through the Utilization of Agro-Industrial Waste. Molecules 2020, 25 (12), 2811. doi:10.3390/molecules25122811.
- Kumar, A.; Chandra, R. Ligninolytic Enzymes and Its Mechanisms for Degradation of Lignocellulosic Waste in Environment. Heliyon 2020, 6 (2), e03170. doi:10.1016/j.heliyon.2020.e03170.
- Hestbjerg, H.; Willumsen, P.A.; Christensen, M.; Andersen, O.; Jacobsen, C.S. Bioaugmentation of Tar-Contaminated Soils under Field Conditions using Pleurotus Ostreatusrefuse from Commercial Mushroom Production. Environ. Toxicol. Chem. 2003, 22 (4), 692–698. doi:10.1002/etc.5620220402.
- Kapahi, M.; Sachdeva, S. Mycoremediation Potential of Pleurotus Species for Heavy Metals: A Review. Bioresources Bioprocess. 2017, 4 (1). doi:10.1186/s40643-017-0162-8.
- Muthusaravanan, S.; Sivarajasekar, N.; Vivek, J.S.; Paramasivan, T.; Naushad, M.; Prakashmaran, J.; Gayathri, V.; Al-Duaij, O.K. Phytoremediation of Heavy Metals: Mechanisms, Methods and Enhancements. Environ. Chem. Lett. 2018, 16 (4), 1339–1359. doi:10.1007/s10311-018-0762-3.
- Tangahu, B.V.; Sheikh Abdullah, S.R.; Basri, H.; Idris, M.; Anuar, N.; Mukhlisin, M. A Review on Heavy Metals (As, Pb, and Hg) Uptake by Plants Through Phytoremediation. Int. J. Chem. Eng. 2011, 1–31. doi:10.1155/2011/939161.
- Awalla, C. Phytoremediation of Sewage Sludge in Soils Contaminated with Heavy Metals. Global J. Environ. Sci. 2015, 12 (1), 13. doi:10.4314/gjes.v12i1.2.
- Eid, E.M.; Galal, T.M.; Sewelam, N.A.; Talha, N.I.; Abdallah, S.M. Phytoremediation of Heavy Metals by Four Aquatic Macrophytes and Their Potential Use as Contamination Indicators: A Comparative Assessment. Environ. Sci. Poll. Res. 2020, 27 (11), 12138–12151. doi:10.1007/s11356-020-07839-9.
- Costa, W.D.; da Silva Bento, A.M.; de Araújo, J.A.S.; Menezes, J.M.C.; da Costa, J.G.M.; da Cunha, F.A.B.; Coutinho, H.D.M.; de Paula Filho, F.J.; Pereira Teixeira, R.N. Removal of Copper(II) Ions and Lead(II) from Aqueous Solutions Using Seeds of Azadirachta Indica A. Juss as Bioadsorvent. Environ. Res. 2020, 183, 109213. doi:10.1016/j.envres.2020.109213.
- Parashar, D.; Satyanarayana, T. An Insight Into Ameliorating Production, Catalytic Efficiency, Thermostability and Starch Saccharification of Acid-Stable α-Amylases from Acidophiles. Front. Bioeng. Biotechnol. 2018, 6, 125.
- Wang, Y.S.; Pan, Z.Y.; Lang, J.M.; Xu, J.M.; Zheng, Y.G. Bioleaching of Chromium from Tannery Sludge by Indigenous Acidithiobacillus Thiooxidans. J. Hazard. Mater. 2007, 147 (1–2), 319–324.
- Branca, T.A.; Colla, V.; Algermissen, D.; Granbom, H.; Martini, U.; Morillon, A.; Pietruck, R.; Rosendahl, S. Reuse and Recycling of By-Products in the Steel Sector: Recent Achievements Paving the Way to Circular Economy and Industrial Symbiosis in Europe. Metals. (Basel) 2020, 10, 345.
- Álvarez, M.L.; Fidalgo, J.M.; Gascó, G.; Méndez, A. Hydrometallurgical Recovery of Cu and Zn from a Complex Sulfide Mineral by Fe3+/H2SO4 Leaching in the Presence of Carbon-Based Materials. Metals. (Basel) 2021, 11, 286.
- Sarkodie, E.K.; Jiang, L.; Li, K.; Yang, J.; Guo, Z.; Shi, J.; Deng, Y.; Liu, H.; Jiang, H.; Liang, Y.; Yin, H.; Liu, X. A Review on the Bioleaching of Toxic Metal(Loid)s from Contaminated Soil: Insight Into the Mechanism of Action and the Role of Influencing Factors. Front. Microbiol. 2022, 13, 1049277.
- Ummalyma, S.B.; Sahoo, D.; Pandey, A. Resource Recovery Through Bioremediation of Wastewaters and Waste Carbon by Microalgae: A Circular Bioeconomy Approach. Environ. Sci. Pollut. Res. Int. 2021, 28, 58837–58856.
- Dickerhof, N.; Isles, V.; Pattemore, P.; Hampton, M.B.; Kettle, A.J. Exposure of Pseudomonas Aeruginosa to Bactericidal Hypochlorous Acid During Neutrophil Phagocytosis is Compromised in Cystic Fibrosis. J. Biol. Chem. 2019, 294, 13502–13514.
- Cha, M.; Lee, N.; Kim, M.; Kim, M.; Lee, S. Heterologous Production of Pseudomonas Aeruginosa EMS1 Biosurfactant in Pseudomonas Putida. Bioresour. Technol. 2008, 99, 2192–9.
- Bayramoglu, G.; Arica, M.Y. Preparation of a Composite Biosorbent Using Scenedesmus Quadricauda Biomass and Alginate/Polyvinyl Alcohol for Removal of Cu(II) and Cd(II) Ions: Isotherms, Kinetics, and Thermodynamic Studies. Water Air Soil Pollut. 2011, 221, 391–403.
- Shanu-Wilson, J.; Evans, L.; Wrigley, S.; Steele, J.; Atherton, J.; Boer, J. Biotransformation: Impact and Application of Metabolism in Drug Discovery. ACS. Med. Chem. Lett. 2020, 11, 2087–2107.
- Igiri, B.E.; Okoduwa, S.I.R.; Idoko, G.O.; Akabuogu, E.P.; Adeyi, A.O.; Ejiogu, I.K. Toxicity and Bioremediation of Heavy Metals Contaminated Ecosystem from Tannery Wastewater: A Review. J. Toxicol. 2018, 1–16. doi:10.1155/2018/2568038.
- Smitha, M.S.; Singh, S.; Singh, R. Microbial bio Transformation: A Process for Chemical Alterations. J Bacteriol Mycol Open Access 2017, 4, 47–51.
- Irawati, W.; Parhusip, A.J.N.; Sopiah, N.; Tnunay, J.A. The Role of Heavy Metals-Resistant Bacteria Acinetobacter sp. in Copper Phytoremediation Using Eichhornia Crasippes [(Mart.) Solms]. KnE Life Sciences 2017, 3, 208–220.
- Viti, C.; Marchi, E.; Decorosi, F.; Giovannetti, L. Molecular Mechanisms of Cr(VI) Resistance in Bacteria and Fungi. FEMS Microbiol. Rev. 2014, 38, 633–659.
- Dhal, B.; Thatoi, H.N.; Das, N.N.; Pandey, B.D. Chemical and Microbial Remediation of Hexavalent Chromium from Contaminated Soil and Mining/Metallurgical Solid Waste: A Review. J. Hazard. Mater. 2013, 250-251, 272–291.
- Joutey, N.T.; Sayel, H.; Bahafid, W.; El-hachtouli, N. Mechanisms of Hexavalent Chromium Resistance and Removal by Microorganisms. Rev. Environ. Contam. Toxicol. 2015, 233, 45–69.
- Miransari, M. Hyperaccumulators, Arbuscular Mycorrhizal Fungi and Stress of Heavy Metals. Biotechnol. Adv. 2011, 29, 645–653.
- Li, F.; Li, Y.X.; Sun, L.M.; Chen, X.L.; An, X.J.; Yin, C.J.; Cao, Y.X.; Wu, H.; Song, H. Modular Engineering Intracellular NADH Regeneration Boosts Extracellular Electron Transfer of Shewanella Oneidensis MR-1. ACS. Synth. Biol. 2018, 7, 885–895.
- Nagvenkar, G.S.; Ramaiah, N. Arsenite Tolerance and Biotransformation Potential in Estuarine Bacteria. Ecotoxicology 2010, 19 (4), 604–613. doi:10.1007/s10646-009-0429-8.
- Correa-García, S.; Pande, P.; Séguin, A.; St-Arnaud, M.; Yergeau, E. Rhizoremediation of Petroleum Hydrocarbons: A Model System for Plant Microbiome Manipulation. Microb. Biotechnol. 2018, 11, 819–832.
- Dixit, R.; Wasiullah, X.; Malaviya, D.; Pandiyan, K.; Singh, U.B.; Sahu, A.; Shukla, R.; Singh, B.P.; Rai, J.P.; Sharma, P.K.; Lade, H. Bioremediation of Heavy Metals from Soil and Aquatic Environment: An Overview of Principles and Criteria of Fundamental Processes. Sustainability 2015, 7, 2189–2212.
- Singh, H.; Pant, G. Phytoremediation: Low Input-Based Ecological Approach for Sustainable Environment. Appl. Water. Sci. 2023, 13, 85.
- Ojuederie, O.B.; Babalola, O.O. Microbial and Plant-Assisted Bioremediation of Heavy Metal Polluted Environments: A Review. Int. J. Environ. Res. Public Health 2017, 14 (12), 1504. doi:10.3390/ijerph14121504.
- Skuza, L.; Szućko-Kociuba, I.; Filip, E.; Bożek, I. Natural Molecular Mechanisms of Plant Hyperaccumulation and Hypertolerance Towards Heavy Metals. Int. J. Mol. Sci. 2022, 23, 9335.
- Zhou, B.; Zhang, T.; Wang, F. Microbial-Based Heavy Metal Bioremediation: Toxicity and Eco-Friendly Approaches to Heavy Metal Decontamination. Appl. Sci 2023, 13, 8439. doi:10.3390/app13148439.
- Bian, F.; Zhong, Z.; Wu, S.; Zhang, X.; Yang, C.; Xiong, X. Comparison of Heavy Metal Phytoremediation in Monoculture and Intercropping Systems of Phyllostachys Praecox and Sedum Plumbizincicola in Polluted Soil. Int. J. Phytoremediation 2018, 20, 490–498.
- Verma, P.; Rawat, S. Rhizoremediation of Heavy Metal- and Xenobiotic-Contaminated Soil: An Eco-Friendly Approach. In Removal of Emerging Contaminants Through Microbial Processes: Shah, M.P., Eds.; Springer, Singapore, 2021.
- Poria, V.; Dębiec-Andrzejewska, K.; Fiodor, A.; Lyzohub, M.; Ajijah, N.; Singh, S.; Pranaw, K. Plant Growth-Promoting Bacteria (PGPB) Integrated Phytotechnology: A Sustainable Approach for Remediation of Marginal Lands. Front. Plant Sci. 2022, 13, 999866.
- Ilias, M.; Rafiqullah, I.M.; Debnath, B.C.; Mannan, K.S.; Mozammel Hoq, M. Isolation and Characterization of Chromium(VI)-Reducing Bacteria from Tannery Effluents. Indian J. Microbiol. 2011, 51, 76–81.
- Pande, V.; Pandey, S.C.; Sati, D.; Bhatt, P.; Samant, M. Microbial Interventions in Bioremediation of Heavy Metal Contaminants in Agroecosystem. Front. Microbiol. 2022, 13. doi:10.3389/fmicb.2022.824084.
- Medfu Tarekegn, M.; Zewdu Salilih, F.; Ishetu, A.I. Microbes Used as a Tool for Bioremediation of Heavy Metal from the Environment. Cogent Food & Agriculture 2020, 6 (1), 1783174. doi:10.1080/23311932.2020.1783174.
- Bhati, A.; Anand, S.R.; Saini, D.; Gunture; Sonkar, S.K. Sunlight-Induced Photoreduction of Cr(VI) to Cr(III) in Wastewater by Nitrogen-Phosphorus-Doped Carbon Dots. Npj Clean Water 2019, 2 (12), 1–8. doi:10.1038/s41545-019-0036-z.
- Teitzel, G.M.; Parsek, M.R. Heavy Metal Resistance of Biofilm and Planktonic Pseudomonas Aeruginosa. Appl. Environ. Microbiol. 2003, 69 (4), 2313–2320. doi:10.1128/AEM.69.4.2313-2320.2003.
- Grujić, S.; Vasić, S.; Čomić, L.; Ostojić, A.; Radojević, I. Heavy Metal Tolerance and Removal Potential in Mixed-Species Biofilm. Water Sci. Technol. 2017, 76 (4), 806–812. doi:10.2166/wst.2017.248.
- Rasulov, O.; Schwarz, M.; Horváth, A.; Zoirov, F.; Fayz, N. Analysis of Soil Contamination with Heavy Metals in (the Three) Highly Contaminated Industrial Zones. SN Applied Sciences 2020, 2 (12). doi:10.1007/s42452-020-03813-9.
- Pfennig, N.; Biebl, H. Desulfuromonas Acetoxidans Gen. Nov. and Sp. Nov., a New Anaerobic, Sulfur-Reducing. Acetate-Oxidizing Bacterium. Arch. Microbiol. 1976, 110 (1), 3–12. doi:10.1007/BF00416962.
- Esser, J.; Brunnert, H. Isolation and Partial Purification of Cadmium-Binding Components from Fruiting Bodies of Agaricus Bisporus. Environ. Poll. Ser. A, Ecol. Biol. 1986, 41 (3), 263–275. doi:10.1016/0143-1471(86)90074-7.
- Lopes-Lima, M.; Burlakova, L.E.; Karatayev, A.Y.; Mehler, K.; Seddon, M.; Sousa, R. Conservation of Freshwater Bivalves at the Global Scale: Diversity, Threats and Research Needs. Hydrobiologia 2018, 810 (1), 1–14. doi:10.1007/s10750-017-3486-7.
- Remenár, M.; Kamlárová, A.; Harichová, J.; Zámocký, M.; Ferianc, P. The Heavy-Metal Resistance Determinant of Newly Isolated Bacterium from a Nickel-Contaminated Soil in Southwest Slovakia. Pol. J. Microbiol. 2018, 67 (2), 191–201. doi:10.21307/pjm-2018-022.
- Zammit, C.M.; Weiland, F.; Brugger, J.; Wade, B.; Winderbaum, L.J.; Nies, D.H.; Southam, G.; Hoffmann, P.; Reith, F. Proteomic Responses to Gold(Iii)-Toxicity in the Bacterium Cupriavidus Metallidurans CH34. Metallomics 2016, 8 (11), 1204–1216. doi:10.1039/C6MT00142D.
- Liu, S.-H.; Zeng, G.-M.; Niu, Q.-Y.; Liu, Y.; Zhou, L.; Jiang, L.-H.; Tan, X.; Xu, P.; Zhang, C.; Cheng, M. Bioremediation Mechanisms of Combined Pollution of PAHs and Heavy Metals by Bacteria and Fungi. A Mini Rev. Bioresour. Technol. 2017, 224, 25–33. doi:10.1016/j.biortech.2016.11.095.
- Giovanella, P.; Cabral, L.; Bento, F.M.; Gianello, C.; Camargo, F.A.O. Mercury (II) Removal by Resistant Bacterial Isolates and Mercuric (II) Reductase Activity in a New Strain of Pseudomonas Sp. B50A. New Biotechnol. 2016, 33 (1), 216–223. doi:10.1016/j.nbt.2015.05.006.
- Manikandan, P.; Moopantakath, J.; Imchen, M.; Kumavath, R.; SenthilKumar, P.K. Identification of Multi-Potent Protein Subtilisin A from Halophilic Bacterium Bacillus Firmus VE2. Microb. Pathog. 2021, 157, 105007. doi:10.1016/j.micpath.2021.105007.
- Zhang, W.; Chen, L.; Liu, D. Characterization of a Marine-Isolated Mercury-Resistant Pseudomonas Putida Strain SP1 and Its Potential Application in Marine Mercury Reduction. Appl. Microbiol. Biotechnol. 2012, 93 (3), 1305–1314. doi:10.1007/s00253-011-3454-5.
- Njoku, K.L.; Akinyede, O.R.; Obidi, O.F. Microbial Remediation of Heavy Metals Contaminated Media by Bacillus Megaterium and Rhizopus Stolonifer. Scientific African 2020, 10, e00545. doi:10.1016/j.sciaf.2020.e00545.
- Jin, Y.; Luan, Y.; Ning, Y.; Wang, L. Effects and Mechanisms of Microbial Remediation of Heavy Metals in Soil: A Critical Review. Appl. Sci. 2018, 8 (8), 1336. doi:10.3390/app8081336.
- Ariffin, N.; Abdullah, M.M.A.B.; Mohd Arif Zainol, M.R.R.; Murshed, M.F.; Hariz-Zain; Faris, M.A.; Bayuaji, R. Review on Adsorption of Heavy Metal in Wastewater by Using Geopolymer. MATEC Web Conferences 2017, 97, 01023. doi:10.1051/matecconf/20179701023.
- Al-Enezi, G.; Hamoda, M.F.; Fawzi, N. Ion Exchange Extraction of Heavy Metals from Wastewater Sludges. J. Enviro. Sci. Health Part A 2004, 39 (2), 455–464. doi:10.1081/ESE-120027536.
- Djedidi, Z.; Bouda, M.; Souissi, M.A.; Cheikh, R.B.; Mercier, G.; Tyagi, R.D.; Blais, J.-F. Metals Removal from Soil, Fly Ash and Sewage Sludge Leachates by Precipitation and Dewatering Properties of the Generated Sludge. J. Hazard. Mater. 2009, 172 (2–3), 1372–1382. doi:10.1016/j.jhazmat.2009.07.144.
- Perumal, S.; Atchudan, R.; Thirukumaran, P.; Yoon, D.H.; Lee, Y.R.; Cheong, I.W. Simultaneous Removal of Heavy Metal Ions Using Carbon Dots-Doped Hydrogel Particles. Chemosphere 2022, 286, 131760. doi:10.1016/j.chemosphere.2021.131760.
- Blöcher, C.; Dorda, J.; Mavrov, V.; Chmiel, H.; Lazaridis, N.K.; Matis, K.A. Hybrid Flotation—Membrane Filtration Process for the Removal of Heavy Metal Ions from Wastewater. Water Res. 2003, 37 (16), 4018–4026. doi:10.1016/S0043-1354(03)00314-2.
- Verma, N.; Sharma, R. Bioremediation of Toxic Heavy Metals: A Patent Review. Recent Pat. Biotechnol. 2017, 11 (3). doi:10.2174/1872208311666170111111631.