ABSTRACT
The ultrasound-degraded oxidized konjac glucomannan (U-OKGM) was manufactured and its immune activity in RAW264.7 macrophages was examined. Results showed that ultrasound treatment, which was an effective method to obtain low molecular weight OKGM, did not change the main structure of polysaccharides under the test conditions. Molecular weights (MW) of OKGM and U-OKGM are about 1.58 × 106 g/mol and 5.64 × 105 g/mol, respectively. We showed that U-OKGM could inhibit NO secretion and augment phagocytotic activity of RAW264.7 cell, and 0.8 mg/mL U-OKGM reached significant level (p < 0.05). Meanwhile, TNF-α and IL-1β secretion levels were significantly (p < 0.05) decreased by U-OKGM treatments in LPS-stimulated RAW264.7 cells. Moreover, the mRNA transcription of IL-1β, TNF-α, IL-6 and iNOS were significantly (p < 0.05) inhibited by U-OKGM in LPS-activated RAW264.7 macrophages. The results suggest that ultrasonic treatment provides a viable modification to obtain low-molecular-weight polysaccharide, and U-OKGM has strong anti-inflammatory effects on LPS-stimulated RAW264.7 macrophages.
RESUMEN
Para este estudio, se elaboró glucomanano de konjac oxidado y degradado por ultrasonidos (U-OKGM), sometiéndose a examen la actividad inmune que proporciona a los macrófagos RAW264.7. Los resultados dan cuenta de que el tratamiento por ultrasonidos demostró ser un método efectivo para obtener un bajo peso molecular de la OKGM; por otra parte, dicho tratamiento no modificó la estructura principal de los polisacáridos sometidos a las condiciones de prueba. Los pesos moleculares (MW) del OKGM y el U-OKGM obtenidos fueron de alrededor de 1.58 × 106 g/mol y 5.64 × 105 g/mol, respectivamente. Se constató que el U-OKGM puede inhibir la secreción de NO y aumentar la actividad fagocítica de las células RAW264.7, además de que la cantidad de U-OKGM lograda, 0.8 mg/mL, fue significativa (p < 0.05). Asimismo, como resultado de los tratamientos de células RAW264.7 estimuladas con LPS con U-OKGM, disminuyeron significativamente (p < 0.05) los niveles de secreción de TNF-α y IL-1β. Aunado a ello, las transcripciones de ARNm de IL-1β, TNF-α, IL-6, y iNOS en macrófagos RAW264.7 activados por LPS fueron inhibidas de manera significativa (p < 0.05) por la U-OKGM. Los resultados sugieren que el tratamiento por ultrasonidos proporciona una modificación viable para obtener un polisacárido de bajo peso molecular, mientras que la U-OKGM proporciona fuertes efectos antiinflamatorios a los macrófagos RAW264.7 estimulados por LPS.
Abbreviations: TNF-α: tumor necrosis factor-α; IL-1β: interleukin-1β; LPS: lipopolysaccharide
1. Introduction
Konjac glucomannan (KGM), a high-molecular-weight polysaccharide that is extracted from the root of the Amorphophallus konjac plant, has been widely used in the food industry. KGM consists of β-1, 4-linked D-glucose and D-mannose residues as the main chain, and the ratio of mannose and glucose units is 1.6:1 (Behera & Ray, Citation2016). Many studies indicate that KGM have many different biological activities, such as immunocompetence (Onishi et al., Citation2005), anti-obesity (Keithley & Swanson, Citation2005; Kraemer et al., Citation2007; Onakpoya, Posadzki, & Ernst, Citation2014), and lipid regulation activities (Chen, Sheu, Tai, Liaw, & Chen, Citation2003; Sood, Baker, & Coleman, Citation2008; Vuksan et al., Citation2001). However, its high molecular weight is a major hindrance for its widespread acceptance as a food additive. Recent studies have demonstrated that hydrolysates of KGM have greater bioactivity than KGM itself (Behera & Ray, Citation2016; Yang et al., Citation2017). A recent study reported that konjac glucomannan hydrolysates inhibit the adhesion of pathogens (e.g. Escherichia coli) to human epithelial cells. This could provide a potential prophylactic and therapeutic approach for the prevention of pathogen–skin adhesion (especially in the gut) and hence restrict infections (Al-Ghazzewi & Tester, Citation2014). Jian et al. (Citation2017) found that degraded KGM showed marked protective effect against oxidative damage and excellent dispersibility. Furthermore, it was reported that hydrolysed glucomannan could encourage wound healing due to a number of immunosupportive effects (Al-Ghazzewi, Elamir, Tester, & Elzagoze, Citation2015). In addition, Yeh, Lin, and Chen (Citation2010) showed that the prebiotic effects of partially hydrolyzed KGM were better than that of KGM.
Oxidized konjac glucomannan (OKGM) is available at high purity as an oxidative degradation product of the plant extract. OKGM can suppress inflammatory cytokines, improve intestinal villus morphology and intestinal flora in C57BL/6J mice fed a high-fat diet (Wu & Wang, Citation2013), and it also can enhance the immune function in mice (Zhao & Wu, Citation2011). However, the molecular weight of OKGM is sufficiently high, which resulted that the application of OKGM in functional food has been very limited to date. It has been reported that OKGM acidolysis products exhibit higher immunomodulatory activity than undegraded OKGM on fish (Zheng et al., Citation2016, Citation2015). However, the effects of low-molecular-weight OKGM on mammals remain unknown. Interestingly, many polysaccharides have similar biological activities in both mammals and aquatic animals, such as Astragalus polysaccharides, and konjac mannanoligosaccharide (KMOS) (Wu et al., Citation2014; Yuan et al., Citation2008).
Various means have been used for partial depolymerization of KGM and other polysaccharides, such as ultrasonic treatment, acidolysis, γ-irradiation, enzyme hydrolysis. Comparing with other methods, the emerging ultrasonic method has advantage of saving energy and time, reducing not only the consumption of organic solvents, but also the waste produced by chemical reaction on the environment and so on (Zhu, Citation2018). In several recent studies, ultrasound has been applied to the controlled degradation of natural polysaccharides such as KGM and xanthan (Li & Feke, Citation2015; Li, Li, Geng, Song, & Wu, Citation2017), leading to changes in water solubility, viscosity and other properties; however, the chemical structure of the polysaccharides remains mostly unchanged (Goodwin et al., Citation2011). Ultrasonic degradation occurs mainly by cleavage of glycosidic bonds of the main chain of polysaccharide. The mechanism of ultrasound degradation of polysaccharides is generally attributed to cavitation effect. Cavitation is well explained by the rapid formation and collapse of cavitation bubbles within the irradiated liquid media, generating intense stress and resulting in irreversible chain scission (Yu et al., Citation2015). It was reported that ultrasound degraded Porphyra yezoensis significantly inhibited the activity of SGC7901 cancer cell (Yu et al., Citation2015). Moreover, it was showed that the antioxidant activity of Cyclocarya paliurus was promoted after ultrasonic treatment (Li et al., Citation2017; Tang et al., Citation2016). In this study, we evaluate the immunomodulatory effects of U-OKGM in LPS-activated mouse RAW264.7 macrophages by measuring IL-1β, TNF-α, and NO production and IL-6, IL-1β, TNF-α, and iNOS mRNA expression to provide a pharmacological rationale for treating inflammatory diseases with U-OKGM.
2. Materials and methods
2.1. Materials and reagents
KGM (purity = 95%) was purchased from Pai Te Konjac Biological Technology Co., Ltd. (Sichuan, China). The mouse macrophage RAW 264.7 cell line was obtained from the Institute of Animal Nutrition (Sichuan Agricultural University). Dulbecco’s Modified Eagle’s Medium (DMEM) and penicillin/streptomycin were purchased from Gibco (Grand Island, NY, USA). ELISA kits for measuring IL-1β and TNF-α expression were purchased from Nanjing Jiancheng Bioengineering Institute (Nanjing, China). Lipopolysaccharide (LPS) was obtained from Sigma (St. Louis, MO, USA). Fetal bovine serum (FBS) was obtained from Zhejiang Tianhang Biotechnology Co., Ltd. (Hangzhou, China). A nitric oxide (NO) assay kit and enhanced cell counting kit-8 (CCK-8) were obtained from the Beijing Biyuntian Company (Beijing, China).
2.2. Preparation of OKGM
KGM (50 g) was dissolved in 250 mL 40% EtOH solution by stirring for 5 min at 180 r/min, holding the temperature under 40°C. Ten-percent hydrochloric acid was used to adjust the pH to 4.3. To oxidize the KGM, 8.75 mL H2O2 (30%) was added to the liquid for 3 times in 30 min at 10 min intervals. After 4 h, Na2SO3 (1 mol/L) was added to terminate the reaction, and 10% NaOH added to adjust pH to 7. The OKGM was filtered with a vacuum filter and washed in three cycles with 50%, 70% and 90% EtOH, and then filtered again. The wet OKGM was dried at 90°C in a drum-wind drying oven and stored.
2.3. Ultrasonic degradation
Dry OKGM (10 g) was weighed and dissolved in 200 mL 50% EtOH solution and then treated with ultrasound. Ultrasonic treatment of 500 W and pulsed as 5s/2s (on/off) was carried out for 20 min by using Scientz-IIE ultrasonic homogenizer (Ningbo Scientz Biotechnology Company, Ltd., Ningbo, Zhejiang, China), and the solution was maintained at 30°C. It was shown in our previous experiments that the ultrasound treatment products of OKGM prepared under these conditions have strongest antioxidant activity. The U-OKGM was washed three times with 70% and 90% EtOH and subsequently dried at 90°C in the drum-wind drying oven. Upon grinding to a powder, the U-OKGM could be passed through a 120-mesh sieve.
2.4. Measurement of molecular weight (MW)
The molecular weight of OKGM and U-OKGM were measured by GPC-MALLS. In the experiment, an Agilent 1260 HPLC system (Agilent Technologies, USA) was coupled to a Brookhaven Instruments-MWA 8-angle MALLS detector (Brookhaven, USA). Separation was achieved using an Ohpak SB-804 HQ column at a flow rate of 0.8 mL/min and 25°C. A NaCl solution (0.25 mol/L) with 0.35% Na2HPO4 filtered through a 0.2-μm Millipore filter was used as the eluent. The sample was diluted and filtered (0.45-μm Millipore filter) prior to injection (100 μL).
2.5. FT-IR analysis
All polysaccharide samples were imbedded in KBr discs, and IR spectra were recorded in the range of 4000 to 400 cm−1 with a resolution of 4 cm−1 at room temperature using the TENSOR27 spectrometer (Bruker, Pittcon, Germany) (Qiu, Zhang, Wang, Liu, & Regenstein, Citation2016).
2.6. Scanning electron microscopy (SEM)
OKGM and U-OKGM samples were placed on an aluminum stub and then sputter-coated with gold and transferred to a Zeiss EVO10 environmental scanning electron microscope (Zeiss, Gottingen, Germany).
2.7. XRD analysis
The polycrystalline lattice parameters of OKGM and U-OKGM polymers were analyzed by X-ray diffraction (XRD). The experiments were performed using an X’pert Pro X-ray diffractometer (PANalytical Company, Netherlands) (Chen et al., Citation2016).
2.8. RAW264.7 macrophage cell culture
RAW264.7 cells were cultured in DMEM containing 10% heat-inactivated FBS and 1% penicillin/streptomycin at 37°C in a moist atmosphere (5% CO2, 95% air).
2.9. Cell viability assay
The viability of RAW264.7 cells was determined using the cell counting kit-8 (CCK-8) assay (Beyotime, Wuhan, China). RAW 264.7 cells were seeded in 96-well plates at a density of 2 × 103 cells/mL in 100 μL medium overnight. The cells were treated with U-OKGM at various concentrations (0, 0.2, 0.4, 0.6, or 0.8 mg/mL dissolved in serum-free medium) or LPS (10 μg/mL dissolved in serum-free medium) at 37°C and 5% CO2. After incubation for 24 h or 48 h, each well was assayed for viability using 10 μL stock CCK-8 solution following the manufacturer’s protocol (1 h exposure in the dark). Optical density was measured at 450 nm on a SpectraMax M2 microplate reader (Molecular Devices, USA). Cell viability was expressed as a percentage of the control.
2.10. Macrophage phagocytosis assay
RAW264.7 cells were seeded in 96-well plates at a density of 2 × 103 cells/mL in 100 μL medium overnight. Macrophages were cultured in the presence of LPS (10 μg/mL) and U-OKGM at various concentrations at 37°C and 5% CO2 for 24 h. Neutral red dye solution (0.1%, 100 μL) was added and then incubated for 3 h. The supernatant was discarded, and the plates were washed three times with PBS to remove any neutral red dye that had not been phagocytosed by the macrophages. Cells were lysed in an ethanol:acetic acid (1:1) solution; 100 μL of cell lysates were allowed to stand for 2 h at room temperature, and the absorbance at 540 nm was measured on a microplate reader.
2.11. Measurement of NO production
RAW264.7 cells were seeded in 96-well plates at a density of 2 × 103 cells/mL in 100 μL medium overnight. The macrophages were then cultured in the presence of LPS (10 μg/mL) and U-OKGM at various concentrations at 37°C and 5% CO2 for 24 h (Jia et al., Citation2012). Supernatant was collected from the plates, and the concentration of NO was determined using a nitric oxide (NO) assay kit (Beyotime, Wuhan, China). The assay was carried out according to the manufacturer’s directions.
2.12. Cytokine assays
RAW264.7 cells were seeded in a 24-well plate at a density of 1 × 105 cells/mL overnight. The medium was then supplemented with LPS (10 μg/mL) and U-OKGM at various concentrations. Plates were incubated at 37°C in a humidified incubator with 5% CO2 for 24 h. Cell-free supernatants were collected to determine IL-1β and TNF-α concentrations by ELISA according to the manufacturers’ protocol (Nanjing Jiancheng Bioengineering Institute, China).
2.13. Reverse transcription-polymerase chain reaction (RT-PCR)
RAW264.7 macrophages (1 × 105 cells) were seeded in a 24-well plate in DMEM/10% FBS and incubated in a 5% CO2 incubator for 24 h. The cells were rinsed with PBS, and the medium was replaced with DMEM/10% FBS containing the various concentrations of U-OKGM in the presence or absence of LPS (10 μg/mL). Total RNA was isolated from the tissue samples using Trizol reagent (TaKaRa, Dalian, China) and treated with RNase-free DNase I according to the manufacturer’s instructions. Approximately 2 μg of total RNA was used for first-strand cDNA synthesis using the PrimeScript RT Kit with gDNA Eraser (TaKaRa, Dalian, China). The primers were designed using Primer Premier 5.0 (PREMIER Biosoft International, CA, USA). We carried out real-time PCR of TNFα, IL-1β, IL-6, iNOS and β-actin on an ABI QS6 Real-Time PCR machine using a SYBR Green marker (TaKaRa, Dalian, China). All of the reactions were repeated three times. To avoid DNA sample contamination, we performed negative RT-PCR control reactions using each total RNA sample as a template. Gene expression results were analyzed using the 2−∆∆Ct method. The primers (Beijing Genomics Institute) are listed in .
Table 1. Sequences of primers for real-time PCR in the study.
Tabla 1. Secuencias en tiempo real de cebadores para la PCR en este estudio.
2.14. Statistical analysis
All data are presented as the mean ± S.E.M and subjected to LSD tests using the software SPSS 16.0 statistical software package (SPSS Inc., Chicago, IL, USA). P-values of < 0.05 were considered to be significant.
3. Results and discussion
3.1. Molecular weight of OKGM and U-OKGM
Much research has shown that low-molecular-weight polysaccharides exhibit higher biological activities because of the low apparent viscosity, simpler structure and good water-solubility (Du, Zeng, Yang, Bian, & Xu, Citation2016; Wang, Cheung, Leung, & Wu, Citation2010). Previous studies have also found that ultrasonic treatment fragmented the polysaccharide from Schizophyllum commune, which produced greater anti-inflammatory activity than the native polymer (Du et al., Citation2016). The increase in the bioactivity may due to the following reason: The particle size, degree of polymerization and molecular weight (MW) of polysaccharide were decreased by hydrolyzing, and these changes can increase the strength of interactions between groups of polysaccharide and protein of the body cell (Yu et al., Citation2015). On the other hand, lower MW polysaccharides with a mild effect of intra-molecular hydrogen bonds would have more free hydroxyl and amino groups (Du et al., Citation2016). Furthermore, low-molecular-weight KGM is more easily utilized or fermentable by intestinal beneficial bacteria (Connolly, Lovegrove, & Tuohy, Citation2010). In addition, it is easy for the low-molecular-weight polysaccharides with these properties to pass through organizational barriers to enter the interior of the cell or attach to the receptors (Kalitnik et al., Citation2013; Zhang, Wang, Mo, & Qi, Citation2013). In the present study, the average molecular weight of OKGM and U-OKGM that were investigated by GPC-MALLS are shown in . We found that our procedure converted OKGM polymers having a Mw of 1.58 × 106 g/mol to a Mw for U-OKGM of 5.64 × 105 g/mol (). It suggested that ultrasonic degradation is an efficient method to fragment OKGM. Following the example of Guo et al. (Citation2014), who reported that ultrasound treatment efficiently decreased the molecular weight of fucoidan.
Table 2. The molecular weight and molecular weight of OKGM and U-OKGM.
Tabla 2. Peso molecular de OKGM y U-OKGM.
3.2. Scanning electron microscopy
shows the surface micrographs of OKGM and U-OKGM powders. After ultrasound treatment, U-OKGM grains appeared smaller than OKGM grains. It could be because of the three effects of ultrasound treatment: intensive cavitation, turbulence shear, and instantaneous high pressure drop. Ultrasonic energy was sufficient to break down the strong bonds, such as glycosidic linkages, connecting the monosaccharide units. Yan, Wang, Ma, and Wang (Citation2016) showed that ultrasound treatment fragmented the microstructure of Phellinus linteus mycelium polysaccharides, producing small segments or pores.
3.3. FT-IR analysis of OKGM and U-OKGM
The FT-IR spectra of OKGM and U-OKGM are shown in . The absorption band at 3332–3334 cm−1 and the peaks at 2883–2884 cm−1 were assigned to the stretching of – OH groups and C–H of methyl groups, respectively. The absorption peak of the carbonyl group appears at 1725 cm−1. The broad peak at 1640–1635 cm−1 is assigned to the stretching frequency of C–O of the associated hydroxyl group. The characteristic absorption bands of mannose appear at 873 and 803 cm−1 (Jin et al., Citation2014; Li et al., Citation2017). As shown in , the spectrum of U-OKGM appears identical to that of OKGM. The IR spectra do not exhibit any significant differences in the chemical structures of the two compounds. Therefore, we infer that ultrasonic degradation conserved the molecular identity. Zhou, Yu, Zhang, He, and Ma (Citation2012) also found that ultrasound degradation does not change the overall structure of polysaccharides. Similarly, Yan et al. (Citation2016) found that the primary structure of Phellinus linteus mycelium polysaccharides is not changed by ultrasound treatment.
3.4. XRD analysis of OKGM and U-OKGM
X-ray diffraction (XRD) analysis has the capacity to provide atomic coordinates, structural features, and measures of amorphous or crystalline content (Chandrasekaran, Citation1998). XRD patterns of OKGM and U-OKGM are shown in . OKGM and U-OKGM samples showed a diffuse scattering and broad peak around 20.1°. These results showed no significant differences in the molecular packing or structural arrangements between OKGM and U-OKGM that can be discerned. This could be due to the fact that KGM is composed two types of monosaccharides with acetyl groups on the side chains that cause the polymeric chain to lack stearic regularity (Dave, Sheth, McCarthy, Ratto, & Kaplan, Citation1998), and the crystalline properties of KGM has been described as “unordered in the long range and ordered in the short range” (Zhang, Li, Wang, Xue, & Xue, Citation2016). Similarly, it was observed that the molecular structure of KGM and deacetylated KGM was loosely arranged, and the molecular interaction was very weak (Zhang et al., Citation2016).
3.5. Effects of U-OKGM on RAW264.7 cell viability
RAW264.7 cells are often used for immunomodulation studies (Hartley et al., Citation2008) and have been found to be useful for measuring immune responses to pro-inflammatory mediators (Dyer et al., Citation2007). Many studies find that lipopolysaccharide (LPS) activates multiple signaling pathways in macrophages, leading to production of pro-inflammatory cytokines such as TNF-α and IL-1β (Kim, Ahn, & Je, Citation2016; Wen et al., Citation2016). The toxicity of U-OKGM on RAW264.7 cell viability was tested by the CCK-8 assay (). Within the tested concentration range (0.2–0.8 mg/mL), our results revealed that U-OKGM had no cytotoxic effects on cells for exposures of either 24 h or 48 h. Since the viability at 48 h was similar to that at 24 h, macrophages were treated with U-OKGM in the concentration range 0.2–0.8 mg/mL for 24 h in all further experiments.
Figure 5. Effect of U-OKGM and LPS on the proliferation of RAW264.7 cells. The endotoxin LPS were selected to stimulate the inflammatory mediator secretions by RAW264.7 macrophages in the present model. Each cell population (2 × 103 cells/ml) was treated with various concentrations of U-OKGM and LPS, respectively. ***p < 0.001, compared with the control group (n = 6).
Figura 5. Efecto de U-OKGM y LPS en la proliferación de células RAW264.7. En este modelo, la endotoxina LPS fue seleccionada para estimular las secreciones mediadoras inflamatorias de los macrófagos RAW264.7. Cada población de células (2 × 103 células/ml) fue tratada con varias concentraciones de U-OKGM y LPS, respectivamente. ***p < 0.001, comparada con el grupo de control (n = 6).
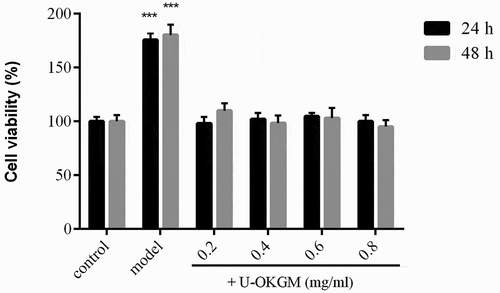
3.6. Neutral red uptake assay
Phagocytosis is the first and key step in the immune response (Kasimu, Chen, Xie, & Li, Citation2017). We used the neutral red uptake assay to monitor phagocytosis of RAW264.7 cells. As shown in , LPS significantly increased dye uptake in RAW264.7 cells compared with the control group that received no exposure (p < 0.01). Moreover, addition of U-OKGM decreased dye uptake levels under the same LPS conditions. Dye uptake by cells receiving 0.8 mg/mL U-OKGM was significantly lower than the group receiving no U-OKGM (p < 0.05). This result showed that the addition of U-OKGM could block or retard activation to a certain extent. Du et al. (Citation2016) reported that similar anti-inflammatory activity of a polysaccharide from Schizophyllum commune could be improved by ultrasound treatment. It was reported that the polysaccharide from Schizophyllum is partially depolymerized by ultrasonication. Of possible relevance is the finding that fragmented and degraded Schizophyllan polysaccharides also exhibit pronounced antitumor activity (Tabata, Ito, Kojima, Kawabata, & Misaki, Citation1981). Mannan-oligosaccharide (MOS), which is obtained by degrading konjac glucomannan chemically or biologically, exhibit several biological activities, such as anti-inflammatory, reducing hyperlipidemia and regulating the intestinal flora and so on (Wang, Zhang, et al., Citation2018). It might be because the structure of U-OKGM is similar to MOS or glucan, therefore, they showed the similar immune effect (Tabata et al., Citation1981; Xing et al., Citation2004). Our work confirms the results of many studies that show that lower-molecular-weight polysaccharides exhibited higher immunogenic activity.
Figure 6. Effect of U-OKGM on macrophage phagocytosis (A), NO production (B), TNF-α production (C) and IL-1β production (D) in RAW264.7 cells with LPS. *p < 0.05, **p < 0.01, ***p < 0.001 compared with the control group (n = 6), #p < 0.05, ##p < 0.01, ###p < 0.001 compared with LPS treatment group (n = 6).
Figura 6. Efecto de la U-OKGM en la fagocitosis de los macrófagos (a), producción de NO (b), producción de TNF-α (c) y producción de IL-1β (d) en células RAW264.7 con LPS. *p < 0.05, **p < 0.01, ***p < 0.001 comparadas con el grupo de control (n = 6), #p < 0.05, ##p < 0.01, ###p < 0.001, comparadas con el grupo de control LPS (n = 6).
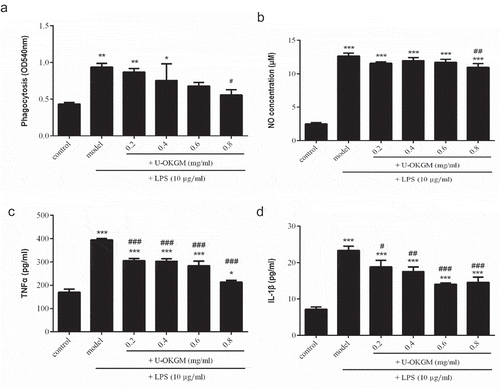
3.7. Effect of U-OKGM on NO release from RAW264.7 cells
Nitric oxide, which is released by activated macrophages, is a chemical messenger and key effector molecule in the immune system. It has been reported that high levels of NO are cytotoxic in inflammation and endotoxemia (Kröncke, Fehsel, & Kolb-Bachofen, Citation1997). We measured NO production after LPS stimulation and found it was higher in stimulated vs. unstimulated groups (p < 0.001, ). Furthermore, cells that were presented with 0.8 mg/mL U-OKGM for 24 h showed significantly inhibited NO production, despite having received the same LPS bolus (p < 0.01). Similar results have been shown for xanthan gum immunosuppressant (Liu et al., Citation2017) that, like U-OKGM, is composed of D-mannose and D-glucose units. Xu, Yasuda, Nakamura-Tsuruta, Mizuno, and Ashida (Citation2012) reported that treatment with β-glucan also results in the striking inhibition of NO production in LPS-activated RAW264.7 cells.
3.8. Effect of U-OKGM on TNF-α and IL-1β expression
LPS-induced activation of macrophages is known to increase the production of cytokines. Cytokines play important roles in immunomodulatory responses and inflammatory reactions. The pro-inflammatory cytokines IL-1β and TNF-α are produced early in inflammation and can regulate the production of many other cytokines. We sought to determine the effect of U-OKGM on TNF-α and IL-1β cytokine release in the LPS-stimulated RAW264.7 cells. As shown in ,), a dose of 10 μg/mL LPS significantly induced IL-1β and TNF-α release. Addition of 0.2–0.8 mg/kg U-OKGM to LPS-activated cells decreased the level of TNF-α and IL-1β in a dose-dependent manner (p < 0.05). A similar decrease was also observed in curdlan-sulfate-suppressed cells (Li et al., Citation2014). In addition, it has been reported that xanthan gum suppresses the production of IL-1β and TNF-α in a dose-dependent manner through the inhibition of mRNA transcription (Liu et al., Citation2017). Interestingly, U-OKGM, curdlan sulfate and xanthan gum all contain the β-glucan structure, and all of them have shown similar immunomodulatory effects. β-glucans exhibit strong immune-suppression (Bonfim-Mendonça, Capoci, Tobaldini-Valerio, Negri, & Svidzinski, Citation2017; Mantovani et al., Citation2008; Yan et al., Citation2018) that depends on their ability to induce or inhibit cytokines in macrophages or to regulate macrophage phagocytosis (Vannucci et al., Citation2013). The first step by which β-glucans exert immune function effects is by their recognition by cells possessing pattern recognition receptors (PRRS), such as the β-receptor. We hypothesize that U-OKGM is also recognized by the β-receptor.
3.9. Effect of U-OKGM on the mRNA expression of TNF-α, IL-1β, IL-6 and iNOS in RAW264.7 cells
IL-6 is a protein secreted by immune cells that plays a key role in triggering the synthesis of acute phase proteins of the liver and regulating the transition from acute to chronic inflammation in general (Kim et al., Citation2016; Wang, Tian, et al., Citation2018). Mihara, Hashizume, Yoshida, Suzuki, and Shiina (Citation2012) demonstrated that IL-6 is strongly involved in experimentally induced autoimmune diseases. They also showed that increased IL-6 levels were associated with Castleman’s disease and rheumatoid arthritis.
To understand how U-OKGM regulates inflammatory factors, the cellular mRNA levels of inflammatory mediators for TNF-α, IL-1β, IL-6 and iNOS were measured by RT-PCR analysis (). Compared with the control group, the LPS group showed significantly increased mRNA levels of all these cytokines (p < 0.001, ). The results indicate that the NO production was up-regulated by LPS stimulation, which preceded by the up-regulation of iNOS mRNA. High levels of NO are known to be cytotoxic in inflammation and endotoxemia (Kröncke et al., Citation1997). That U-OKGM significantly decreases iNOS mRNA levels in LPS-activated macrophages and that NO levels are eventually diminished suggests that U-OKGM could effectively inhibit NO production via inhibition of iNOS transcription. One mechanism might be because U-OKGM could be recognized by some cells possessing pattern recognition receptors (PRRS), then caused an immune response, and the resultant decrease in the expression of iNOS mRNA (Wen et al., Citation2016). Furthermore, ultrasound treated KGM have been evaluated as prebiotic substrate for the growth of probiotic bacteria of human or animal gut microbiota (Mao, Song, Yao, & Wu, Citation2018). Prebiotic carbohydrates are non-digestible food ingredients such as carbohydrate fibres that can selectively stimulate the growth and metabolic activity of certain bacteria in the colon in a way beneficial to the host health, and then exert direct effects on the immune system (Yahfoufi, Mallet, Graham, & Matar, Citation2018).” Moreover, Chen et al. (Citation2017) also showed that A-OKGM promotes IL-1β, IL-6, and TNFα gene expression in S. prenanti gut, and then induced an immune response. We also show that 0.2–0.8 mg/kg U-OKGM, administered over 24 h, inhibited the expression of TNF-α, IL-1β and IL-6 compared with the LPS group (). Our results suggest that U-OKGM may be used to manage the over-expression of inflammatory cytokines. A similar effect is also observed in the response to xanthan gum, which has been reported to significantly inhibit mRNA expression of TNF-α, IL-1β, IL-6 and iNOS in LPS-stimulated RAW264.7 macrophages (Yu et al., Citation2015).
Figure 7. Effect of U-OKGM on TNFα (a), IL-1β (b), iNOS (c) and IL-6 (d) mRNA expression in RAW264.7 cells with LPS. *p < 0.005, **p < 0.01, ***p < 0.001, compared with the control group (n = 3); #p < 0.05, ##p < 0.01, ###p < 0.001 compared with LPS treatment group (n = 3).
Figura 7. Efecto del U-OKGM en TNFα (a), IL-1β (b), iNOS (c) e IL-6 (d) en la expresión del ARNm en células RAW264.7 con LPS. *p < 0.005, **p < 0.01, ***p < 0.001, comparada con el grupo de control (n = 3); #p < 0.05, ##p < 0.01, ###p < 0.001 comparada con el grupo de tratamiento LPS (n = 3).
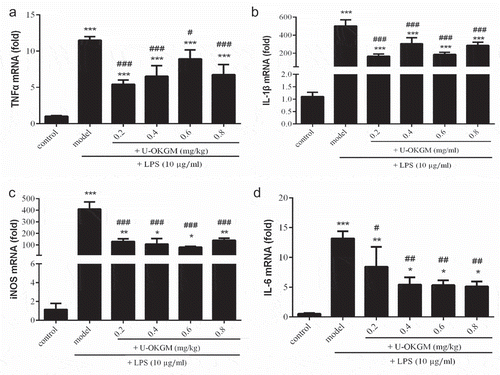
4. Conclusion
In summary, our results indicate that ultrasonication is an effective method to reduce the molecular weight and raise the immune-suppression capability of OKGM. We found that 0.2–0.8 mg/kg U-OKGM administered over a 24 h period significantly inhibited the expression of TNF-α, IL-1β and NO in LPS-stimulated RAW264.7 macrophages in a dose-dependent manner (p < 0.05). Because cellular levels of mRNA for TNF-α, IL-1β, IL-6 and iNOS also fall after the administration of U-OKGM (p < 0.05), we suggest that the immuno-suppressor might be acting at the level of transcription. We hypothesize that it may be used to control disease-associated, pro-inflammatory cytokine expression. The molecular mechanism(s) involved in this process will be further studied in future work.
Acknowledgments
We thank Prof. DW Chen, Dr. AM Wu, Dr. JW Yang and H Li at Institute of Animal Nutrition, Sichuan Agricultural University for their help in providing test instruments and technical suggestions. We thank LetPub (www.letpub.com) for its linguistic assistance during the preparation of this manuscript.
Disclosure statement
No potential conflict of interest was reported by the authors.
Additional information
Funding
References
- Al-Ghazzewi, F., Elamir, A., Tester, R., & Elzagoze, A. (2015). Effect of depolymerised konjac glucomannan on wound healing. Bioactive Carbohydrates and Dietary Fibre, 5(2), 125–128.
- Al-Ghazzewi, F. H., & Tester, R. F. (2014). Inhibition of the adhesion of Escherichia coli to human epithelial cells by carbohydrates. Bioactive Carbohydrates and Dietary Fibre, 4(1), 1–5.
- Behera, S. S., & Ray, R. C. (2016). Konjac glucomannan, a promising polysaccharide of Amorphophallus konjac K. Koch in health care. International Journal of Biological Macromolecules, 92, 942–956.
- Bonfim-Mendonça, P. S., Capoci, I. R. G., Tobaldini-Valerio, F. K., Negri, M., & Svidzinski, T. (2017). Overview of β-glucans from laminaria spp.: Immunomodulation properties and applications on biologic models. International Journal of Molecular Sciences, 18(9), 1629.
- Chandrasekaran, R. (1998). X-ray diffraction of food polysaccharides. Advances in Food and Nutrition Research, 38, 131.
- Chen, H. L., Sheu, W. H., Tai, T. S., Liaw, Y. P., & Chen, Y. C. (2003). Konjac supplement alleviated hypercholesterolemia and hyperglycemia in type 2 diabetic subjects-a randomized double-blind trial. Journal of the American College of Nutrition, 22, 36–42.
- Chen, M., Wang, S., Liang, X., Ma, D., He, L., & Liu, Y. (2017). Effect of dietary acidolysis-oxidized konjac glucomannan supplementation on serum immune parameters and intestinal immune-related gene expression of Schizothorax prenanti. International Journal of Molecular Sciences, 18(12), 2558.
- Chen, Y., Zhao, H., Liu, X., Li, Z., Liu, B., Wu, J., … Li, Y. (2016). TEMPO-oxidized konjac glucomannan as appliance for the preparation of hard capsules. Carbohydrate Polymers, 143, 262–269.
- Connolly, M. L., Lovegrove, J. A., & Tuohy, K. M. (2010). Konjac glucomannan hydrolysate beneficially modulates bacterial composition and activity within the faecal microbiota. Journal of Functional Foods, 2, 219–224.
- Dave, V., Sheth, M., McCarthy, S. P., Ratto, J. A., & Kaplan, D. L. (1998). Liquid crystalline, rheological and thermal properties of konjac glucomannan. Polymer, 39(5), 1139–1148.
- Du, B., Zeng, H., Yang, Y., Bian, Z., & Xu, B. (2016). Anti-inflammatory activity of polysaccharide from Schizophyllum commune as affected by ultrasonication. International Journal of Biological Macromolecules, 91, 100–105.
- Dyer, K. D., Schellens, I. M. M., Bonville, C. A., Martin, B. V., Domachowske, J. B., & Rosenberg, H. F. (2007). Efficient replication of pneumonia virus of mice (PVM) in a mouse macrophage cell line. Virology Journal, 4(1), 48.
- Goodwin, D. J., Picout, D. R., Ross-Murphy, S. B., Holland, S. J., Martini, L. G., & Lawrence, M. J. (2011). Ultrasonic degradation for molecular weight reduction of pharmaceutical cellulose ethers. Carbohydrate Polymers, 83(2), 843–851.
- Guo, X., Ye, X., Sun, Y., Wu, D., Wu, N., Hu, Y., & Chen, S. (2014). Ultrasound effects on the degradation kinetics, structure, and antioxidant activity of sea cucumber fucoidan. Journal of Agricultural and Food Chemistry, 62(5), 1088–1095.
- Hartley, J. W., Evans, L. H., Green, K. Y., Naghashfar, Z., Macias, A. R., Zerfas, P. M., & Ward, J. M. (2008). Expression of infectious murine leukemia viruses by RAW264. 7 cells, a potential complication for studies with a widely used mouse macrophage cell line. Retrovirology, 5(1), 1.
- Jia, Y., Ji, L., Zhang, S., Xu, L., Yin, L., Li, L., … Peng, J. (2012). Total flavonoids from Rosa Laevigata Michx fruit attenuates hydrogen peroxide induced injury in human umbilical vein endothelial cells. Food and Chemical Toxicology, 50(9), 3133–3141.
- Jian, W., Tu, L., Wu, L., Xiong, H., Pang, J., & Sun, Y. M. (2017). Physicochemical properties and cellular protection against oxidation of degraded konjac glucomannan prepared by γ-irradiation. Food Chemistry, 231, 42–50.
- Jin, W., Xu, W., Li, Z., Li, J., Zhou, B., Zhang, C., & Li, B. (2014). Degraded konjac glucomannan by γ-ray irradiation assisted with ethanol: Preparation and characterization. Food Hydrocolloids, 36, 85–92.
- Kalitnik, A. A., Barabanova, A. O. B., Nagorskaya, V. P., Renunov, A. V., Glazunov, V. P., Solov’eva, T. F., & Yermak, I. M. (2013). Low molecular weight derivatives of different carrageenan types and their antiviral activity. Journal of Applied Phycology, 25(1), 65–72.
- Kasimu, R., Chen, C., Xie, X., & Li, X. (2017). Water-soluble polysaccharide from Erythronium sibiricum bulb: Structural characterisation and immunomodulating activity. International Journal of Biological Macromolecules, 105, 452–462.
- Keithley, J., & Swanson, B. (2005). Glucomannan and obesity: A critical review. Alternatives Therapies in Health and Medicine, 11, 30–34.
- Kim, Y. S., Ahn, C. B., & Je, J. Y. (2016). Anti-inflammatory action of high molecular weight Mytilus edulis hydrolysates fraction in LPS-induced RAW264. 7 macrophage via NF-κB and MAPK pathways. Food Chemistry, 202, 9–14.
- Kraemer, W. J., Vingren, J. L., Silvestre, R., Spiering, B. A., Hatfield, D. L., Ho, J. Y., … Volek, J. S. (2007). Effect of adding exercise to a diet containing glucomannan. Metabolism Clinical and Experimental, 56, 1149–1158.
- Kröncke, K. D., Fehsel, K., & Kolb-Bachofen, V. (1997). Nitric oxide: Cytotoxicity versus cytoprotection—How, why, when, and where? Nitric Oxide, 1(2), 107–120.
- Li, J., Li, B., Geng, P., Song, A. X., & Wu, J. Y. (2017). Ultrasonic degradation kinetics and rheological profiles of a food polysaccharide (konjac glucomannan) in water. Food Hydrocolloids, 70, 14–19.
- Li, P., Zhang, X., Cheng, Y., Li, J., Xiao, Y., Zhang, Q., … Wang, F. (2014). Preparation and in vitro immunomodulatory effect of curdlan sulfate. Carbohydrate Polymers, 102, 852–861.
- Li, R., & Feke, D. L. (2015). Rheological and kinetic study of the ultrasonic degradation of xanthan gum in aqueous solution: Effects of pyruvate group. Carbohydrate Polymers, 124, 216–221.
- Liu, F., Zhang, X., Ling, P., Liao, J., Zhao, M., Mei, L., … Wang, F. (2017). Immunomodulatory effects of xanthan gum in LPS-stimulated RAW 264.7 macrophages. Carbohydrate Polymers, 169, 65–74.
- Mantovani, M. S., Bellini, M. F., Angeli, J. P. F., Oliveira, R. J., Silva, A. F., & Ribeiro, L. R. (2008). β-Glucans in promoting health: Prevention against mutation and cancer. Mutation Research, 658(3), 154–161.
- Mao, Y. H., Song, A. X., Yao, Z. P., & Wu, J. Y. (2018). Protective effects of natural and partially degraded konjac glucomannan on Bifidobacteria against antibiotic damage. Carbohydrate Polymers, 181, 368–375.
- Mihara, M., Hashizume, M., Yoshida, H., Suzuki, M., & Shiina, M. (2012). IL-6/IL-6 receptor system and its role in physiological and pathological conditions. Clinical Science, 122(4), 143–159.
- Onakpoya, I., Posadzki, P., & Ernst, E. (2014). The efficacy of glucomannan supplementation in overweight and obesity: A systematic review and meta-analysis of randomized clinical trials. Journal of the American College of Nutrition, 33(1), 70–78.
- Onishi, N., Kawamoto, S., Nishimura, M., Nakano, T., Aki, T., Shigeta, S., … Ono, K. (2005). A new immunomodulatory function of low-viscous konjac glucomannan with a small particle size: Its oral intake suppresses spontaneously occurring dermatitis in NC/Nga mice. International Archives of Allergy and Immunology, 136(3), 258–265.
- Qiu, J., Zhang, H., Wang, Z., Liu, S., & Regenstein, J. M. (2016). Response surface methodology for the synthesis of an Auricularia auriculajudae polysaccharides-CDDP complex. International Journal of Biological Macromolecules, 93, 333–343.
- Sood, N., Baker, W. L., & Coleman, C. I. (2008). Effect of glucomannan on plasma lipid and glucose concentrations, body weight and blood pressure: Systemic review and meta-analysis. American Journal of Clinical Nutrition, 88, 1167–1175.
- Tabata, K., Ito, W., Kojima, T., Kawabata, S., & Misaki, A. (1981). Ultrasonic degradation of schizophyllan, an antitumor polysaccharide produced by Schizophyllum commune fries. Carbohydrate Research, 89(1), 121–135.
- Tang, W., Lin, L., Xie, J., Wang, Z., Wang, H., Dong, Y., … Xie, M. (2016). Effect of ultrasonic treatment on the physicochemical properties and antioxidant activities of polysaccharide from Cyclocarya paliurus. Carbohydrate Polymers, 151, 305–312.
- Vannucci, L., Krizan, J., Sima, P., Stakheev, D., Caja, F., Rajsiglova, L., … Saieh, M. (2013). Immunostimulatory properties and antitumor activities of glucans. International Journal of Oncology, 43(2), 357–364.
- Vuksan, V., Sievenpiper, J. L., Xu, Z., Wong, E. Y. Y., Jenkins, A. L., Beljan-Zdravkovic, U., … Stavro, M. P. (2001). Konjac-mannan and American ginsing: Emerging alternatives therapies for type 2 diabetes mellitus. Journal of the American College of Nutrition, 20, 370S–380S.
- Wang, H., Zhang, X., Wang, S., Li, H., Lu, Z., Shi, J., & Xu, Z. (2018). Mannan-oligosaccharide modulates the obesity and gut microbiota in high-fat diet-fed mice. Food & Function, 9(7), 3916–3929.
- Wang, Y., Tian, Y., Shao, J., Shu, X., Jia, J., Ren, X., & Guan, Y. (2018). Macrophage immunomodulatory activity of the polysaccharide isolated from Collybia radicata mushroom. International Journal of Biological Macromolecules, 108, 300–306.
- Wang, Z. M., Cheung, Y. C., Leung, P. H., & Wu, J. Y. (2010). Ultrasonic treatment for improved solution properties of a high-molecular weight exopolysaccharide produced by a medicinal fungus. Bioresource Technology, 101(14), 5517–5522.
- Wen, Z. S., Xiang, X. W., Jin, H. X., Guo, X. Y., Liu, L. J., Huang, Y. N., … Qu, Y. L. (2016). Composition and anti-inflammatory effect of polysaccharides from Sargassum horneri in RAW264.7 macrophages. International Journal of Biological Macromolecules, 88, 403–413.
- Wu, Y. L., & Wang, X. (2013). Effect of oxidized konjac glucomannan on inflammatory cytokines in C57BL/6J mice fed a high-fat diet. Food Science, 34(23), 321–325. (in Chinese).
- Wu, Z. X., Yu, Y. M., Chen, X., Liu, H., Yuan, J. F., Shi, Y., & Chen, X. X. (2014). Effect of prebiotic konjac mannanoligosaccharide on growth performances, intestinal microflora, and digestive enzyme activities in yellow catfish, Pelteobagrus fulvidraco. Fish Physiology and Biochemistry, 40(3), 763–771.
- Xing, R., Liu, S., Yu, H., Zhang, Q., Li, Z., & Li, P. (2004). Preparation of low-molecular-weight and high-sulfate-content chitosans under microwave radiation and their potential antioxidant activity in vitro. Carbohydrate Research, 339(15), 2515–2519.
- Xu, X., Yasuda, M., Nakamura-Tsuruta, S., Mizuno, M., & Ashida, H. (2012). β-Glucan from Lentinus edodes inhibits nitric oxide and tumor necrosis factor-α production and phosphorylation of mitogen-activated protein kinases in lipopolysaccharide-stimulated murine RAW 264.7 macrophages. Journal of Biological Chemistry, 287(2), 871–878.
- Yahfoufi, N., Mallet, J. F., Graham, E., & Matar, C. (2018). Role of probiotics and prebiotics in immunomodulation. Current Opinion in Food Science, 20, 82–91.
- Yan, J., Han, Z., Qu, Y., Yao, C., Shen, D., Tai, G., … Zhou, Y. (2018). Structure elucidation and immunomodulatory activity of a β-glucan derived from the fruiting bodies of Amillariella mellea. Food Chemistry, 240, 534–543.
- Yan, J. K., Wang, Y. Y., Ma, H. L., & Wang, Z. B. (2016). Ultrasonic effects on the degradation kinetics, preliminary characterization and antioxidant activities of polysaccharides from Phellinus linteus mycelia. Ultrasonics Sonochemistry, 29, 251–257.
- Yang, J., Vittori, N., Wang, W., Shi, Y. C., Hoeflinger, J. L., Miller, M. J., & Pan, Y. (2017). Molecular weight distribution and fermentation of mechanically pre-treated konjac enzymatic hydrolysates. Carbohydrate Polymers, 159, 58–65.
- Yeh, S., Lin, M., & Chen, H. (2010). Partial hydrolysis enhances the inhibitory effects of konjac glucomannan from Amorphophallus konjac C. Koch on DNA damage induced by fecal water in Caco-2 cells. Food Chemistry, 119, 614–618.
- Yu, X., Zhou, C., Yang, H., Huang, X., Ma, H., Qin, X., & Hu, J. (2015). Effect of ultrasonic treatment on the degradation and inhibition cancer cell lines of polysaccharides from Porphyra yezoensis. Carbohydrate Polymers, 117, 650–656.
- Yuan, C., Pan, X., Gong, Y., Xia, A., Wu, G., Tang, J., & Han, X. (2008). Effects of Astragalus polysaccharides (APS) on the expression of immune response genes in head kidney, gill and spleen of the common carp, Cyprinus carpio L. International Immunopharmacology, 8(1), 51–58.
- Zhang, T., Li, Z., Wang, Y., Xue, Y., & Xue, C. (2016). Effects of konjac glucomannan on heat-induced changes of physicochemical and structural properties of surimi gels. Food Research International, 83, 152–161.
- Zhang, Z., Wang, X., Mo, X., & Qi, H. (2013). Degradation and the antioxidant activity of polysaccharide from Enteromorpha linza. Carbohydrate Polymers, 92(2), 2084–2087.
- Zhao, Q., & Wu, Y. L. (2011). Effects of oxidized konjac glucomannan on immune function in mice. Acta Nutrimenta Sinica, 5, 11.
- Zheng, Q., Wu, Y., Xu, H., Wang, H., Tang, H., Xia, X., & Feng, J. (2016). Immune responses to Aeromonas hydrophila infection in Schizothorax Prenanti fed with oxidized konjac glucomannan and its acidolysis products. Fish & Shellfish Immunology, 49, 260–267.
- Zheng, Q., Wu, Y., Xu, H., Yao, Y., Xia, X., Feng, J., … Wang, H. (2015). The effects of dietary oxidized konjac glucomannan and its acidolysis products on the immune response, expression of immune related genes and disease resistance of Schizothorax prenanti. Fish & Shellfish Immunology, 45(2), 551–559.
- Zhou, C., Yu, X., Zhang, Y., He, R., & Ma, H. (2012). Ultrasonic degradation, purification and analysis of structure and antioxidant activity of polysaccharide from Porphyra yezoensis Udea. Carbohydrate Polymers, 87(3), 2046–2051.
- Zhu, F. (2018). Modifications of konjac glucomannan for diverse applications. Food Chemistry, 256, 419–426.