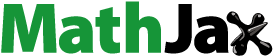
ABSTRACT
This research aimed to develop nanosuspensions as carriers of Annona muricata acetogenins (ACGs) using different concentrations of soy lecithin (SL), hydroxypropyl-β-cyclodextrin (βCD) and the dispersion method (low agitation, middle agitation and ultrasound) on particle size and polydispersity index (PDI). A response surface analysis was performed to find an optimized, validated and characterized nanosuspension (OβCDSL-ACGs-NSps) and to measure its antibacterial activity. The best conditions to formulate OβCDSL-ACGs-NSps with spherical nanoparticles (133.77 nm), PDI (0.20) and good stability (−37 mV) were 10 mg/mL SL, 0.16% βCD and ultrasound as a dispersion method. Nanoparticles from OβCDSL-ACGs-NSps exhibited a high entrapment (85.33%) and encapsulation efficiency (56.09%). The highest release (84.15%) of ACGs was observed until 36 h. OβCDSL-ACGs-NSps also showed significant antibacterial inhibition against E. faecalis (87%) and L. monocytogenes (75%) at 320 µg/mL. βCDSL-ACGs-NSps is an attractive and promising alternative to improve the solubility and bioavailability of ACGs in pharmaceutical applications.
1. Introduction
Recent studies have shown that Annona muricata seeds are a rich source of acetogenins (ACGs) and could be used as a raw material for obtaining these compounds (Aguilar-Hernández et al., Citation2022). ACGs are molecules with 35–37 carbon atoms attached to one, two or three tetrahydrofuran or tetrahydropyran rings in their central region, several oxygenated groups (−OH), and an unsaturated or saturated γ-lactone-α-β (Bermejo et al., Citation2005).
The biological activities of ACGs have been attributed to the inhibition of complex I of mitochondria and NADH oxidase of plasma membranes in tumoral cells, causing apoptosis (Akhmad et al., Citation2022). Consequently, they have been used in traditional medicine to treat various diseases, including antibacterial and antihemolytic activities (Coria-Téllez et al., Citation2018).
León-Fernández et al. (Citation2019) demonstrated the antibacterial activity of rich extracts in ACGs (2 mg/mL) from A. muricata pulp. These authors found a percentage inhibition of 26–40% on E. faecalis. On the other hand, Aguilar-Hernández et al. (Citation2023a) demonstrated that isolated ACGs (12.5–4000 µg/mL) from A. muricata seeds had an antibacterial effect (57–98.42%) against Gram-positive and Gram-negative bacteria.
Although ACGs exhibit a broad spectrum of biological activities, their poor solubility in aqueous media limits their bioavailability as a drug (Hong et al., Citation2016a, Citation2016b). A low solubility represents a lower bioavailability and irregular absorption, resulting in undesirable therapeutic effects such as ineffectiveness of the treatment and increased morbidity, toxicity, and risk of death (Gutiérrez et al., Citation2020). In addition, some studies have reported that isolated ACGs have neurotoxic effects at high doses that can trigger various disorders such as Parkinson’s disease. Nonetheless, it has been shown that ACGs at doses of 10 mg/kg orally and 0.5 mg/kg intravenously (murine model) can cross the blood-brain barrier and show no signs of toxicity (Ferreira et al., Citation2023). Therefore, an alternative to solve solubility and toxicity issues is developing a system to deliver ACGs; nanosuspensions (NSPs) could be an effective and viable option (Hong et al., Citation2017).
NSps are biphasic systems of dispersed drug particles in an aqueous vehicle coated by a polymeric matrix with a diameter of less than 200 nm (Chamundeeswari et al., Citation2018). It has been demonstrated that NSps help increase the solubility, biocompatibility, and bioavailability of highly insoluble active ingredients, thus enhancing their use in pharmaceutical applications (Kim et al., Citation2021; Ma et al., Citation2023). A study developed by Hong et al. (Citation2016a) demonstrated that NSps loaded with bullatacin (43%) using hydroxypropyl-β-cyclodextrin and soybean lecithin as stabilizers showed a mean particle size of 144.4 nm, 0.20 PDI, good stability (−22 mV); however, a low drug loading (46.17%). Similarly, Hong et al. (Citation2017) and Li et al. (Citation2018) formulated NSPs using the same polymer (hydroxypropyl-β-cyclodextrin) with folic acid, soybean lecithin, ACGs (bullatacin) and a dispersion method of low energy. The authors reported an average particle size of 119.7–199.5 nm and a drug payload of 49.68%, concluding that the particle size and stability depend on the type and quantity of ingredients. However, if NSps-loaded bullatacin is formulated with different block polymers, the particle size can vary from 120–180 nm (Hong et al., Citation2016b). Han et al. (Citation2021) developed NSps-loaded bullatacin (43%) using poloxamer 188 as a stabilizer, achieving a mean particle size of 115.9 nm.
However, to obtain stable and cheap NSps, it is necessary to evaluate different experimental conditions such as dispersion methods, the amount and type of active ingredient, wall materials, and/or surfactant to get NSps with homogeneous nanometric particle size, good stability, and the highest encapsulation efficiency (Aguilar-Hernández et al., Citation2023b). It has been demonstrated that a smaller particle size allows intravenous administration of poorly soluble drugs without blockage of blood capillaries and increases bioavailability and permeability (Han et al., Citation2021; Shetab-Boushehri et al., Citation2020).
In this study, the optimization to develop NSps loaded with an ACGs mixture containing 60% pseudoannonacin and 6% annonacin was performed. Moreover, different spectroscopic techniques were used to characterize the optimized NSps and their antimicrobial effect.
2. Materials and methods
2.1. Materials
The ACGs used in this study were obtained previously by López-Romero et al. (Citation2022). Briefly, ACGs were extracted from the A. muricata seeds using methanol as solvent and thermosonication as extraction method (50°C, 50 min, 100% amplitude, and 0.5 s pulse cycles). The extract was dried with a rotary evaporator (Yamato RE300, Tokyo, Japan) and subjected to purification by open-column chromatography (López-Romero et al., Citation2022).
2.2. Bacterial strains
Enterococcus faecalis (ATCC 51,575) and Listeria monocytogenes (ATCC 15,313) were provided by the University of Guadalajara and handled according to the manufacturer’s instructions (Microbiologics®, Saint Cloud, MN, U.S.A.). Before use, all instruments and reagents for the various microbiological assays were sterilized for 15 minutes at 121°C.
2.3. Preparation of acetogenin-loaded nanosuspensions using Box-Behnken design
A Box-Behnken design (BBD) with three levels for each factor was employed to develop NSps loaded with ACGs (βCDSL-ACGs-NSps). The individual and interaction effect of the concentration of hydroxypropyl-β-cyclodextrin (XCD 0.05, 0.1, and 0.2%), soy lecithin (XSL 5, 10, and 15 mg/mL) and the dispersion method (XPM low agitation 500 rpm, middle agitation 10,000 rpm, and ultrasound bath with 50 W potency, 40 kHz frequency, (Cole-Parmer, Vernon Hills, IL, U.S.A.) on the particle size of the βCDSL-ACGs-NSps were evaluated.
βCDSL-ACGs-NSps were developed according to Hong et al. (Citation2016a). Briefly, βCD (0.05, 0.1 or 0.2% w/v) was dissolved in distilled water (10 mL), and SL (5, 10 or 15 mg/mL) was dissolved in methanol. SL solution (800 µL) was mixed with βCD solution, adding drop by drop by applying the dispersion method according to BBD for 5 min to obtain the amphiphilic complex (βCD-SL complex). Methanol was evaporated from the βCD-SL complex using a rotary evaporator (Yamato RE300, Tokyo, Japan). ACGs were dissolved in methanol (4.16 mg/mL), and 800 µL of this solution was added drop by drop into the βCD-SL complex, applying the dispersion method established in the BBD for 5 min. Then methanol evaporated from the last mixture, obtaining βCDSL-ACGs-NSps with 320 µg/mL of ACGs and NSPs were used for various analyses.
2.4. Particle size and polydispersity index (PDI)
All βCDSL-ACGs-NSps were evaluated by measuring particle size and PDI using classic dynamic light scattering at 90° in an equipment ZN90 nanosizer (Malvern Instruments, Cambridge, UK). Each NSps was analyzed in triplicate (20 scans) at 25°C.
2.5. Optimization of the experimental conditions for the development of βCDSL-ACGs-NSps employing the response surface methodology (RSM)
RSM was performed with particle size data to obtain the optimal experimental conditions for preparing βCDSL-ACGs-NSps. A second-order polynomial equation was obtained and used to calculate the predicted values (EquationEquation (1)(1)
(1) )
Where Y is the expected particle size, Xi is the coded or uncoded value for the independent variables (XPEG, XSL, and XPM), β0 is a constant, βi are the principal effect coefficients for each variable, and βij are the interaction effect coefficients.
The adequacy of the model was assessed by analysis of variance (ANOVA) to determine the significant effect of the independent variables and correlation coefficients (R2 squared) at a significance level of 5%. In addition, the data were adjusted (adjusted R2) with the second-order polynomial equation by multiple regression using Statistic software (v. 10 StatSoft, Tulsa, OK, U.S.A.).
2.6. Validation of the optimal experimental conditions to develop the optimized βCDSL-ACGs-NSps (OβCDSL-ACGs-NSps)
OβCDSL-ACGs-NSps were prepared under the optimal conditions provided by RSM. They were analyzed with particle size, PDI, zeta potential (Z-potential), entrapment, and encapsulation efficiency to verify the model’s reliability.
The entrapment and entrapment efficiency of ACGs inside nanoparticles were determined using the methodology proposed by Venugopal et al. (Citation2016). Briefly, 10 mL of OβCDSL-ACGs-NSps was added to a dialysis membrane (Sigma Aldrich, St. Louis, MO, U.S.A.) which was immersed in phosphate buffer saline (PBS) (pH 7.4) with stirring (500 rpm) at 37 ± 2°C. Aliquots of NSPs (500 µL) were taken after 15 min and 24 h of dialysis from inside the membrane. The ACGs content in aliquots after these times of dialysis was determined. The aliquots were shaken at 5000 rpm with a tissue tearor homogenizer (Model 985370, Biospec, Xiamen, China) for 5 min. Subsequently, ACGs were separated from the amphiphilic complex with 500 µL mL of chloroform. The chloroform was evaporated, and ACGs were resuspended in methanol (1 mL) and mixed with 2 mL of Kedde’s reagent. The absorbances at 505 nm were measured in a spectrophotometer (Jenway 6705, Dunmow, UK). The ACGs content was calculated using a calibration curve of an annonacin standard (López-Romero et al., Citation2022). The encapsulation efficiency was determined according to EquationEquation (2)(2)
(2) .
E1 is the concentration of ACGs in optimized NSPs after 15 min of dialysis, and E2 is the concentration of released ACGs after 24 h of dialysis.
The entrapment efficiency was evaluated after exposing the NSPs under the conditions mentioned above with EquationEquation (3)(3)
(3) :
C1 is the concentration of ACGs in optimized NSPs (320 μg/mL), and C2 is the amount on the surface and no-entrapped ACGs in the nanosuspension. C2 is the concentration of released ACGs after 15 min starting dialysis.
2.7. Spectroscopic characterization of isolated ACGs and the OβCDSL-ACGs-NSps
2.7.1. Fourier transform infrared spectroscopy (FTIR)
Isolated ACGs, βCD-SL complex, and optimized NSps were performed by FTIR (iS10 FTIR, Thermo Scientific, Waltham, MA, U.S.A.). The spectra were recorded at room temperature (25 ± 2°C) in the range of 4000 to 500 cm−1 with 24 scans and a resolution of 4 cm−1 (Anaya-Esparza et al., Citation2021).
2.7.2. X-ray diffraction (XRD)
The crystallinity of ACGs, βCD-SL complex and OβCDSL-ACGs-NSps was obtained by measuring X-ray diffraction patterns (XRD Empyrean, Malvern Panalytical, Almelo, The Netherlands) equipped with Cu-Kα radiation (λ = 0.154 nm) a 40 kV voltage generator and a tube current of 30 mA.
2.7.3. UV-VIS diffuse reflectance spectroscopy
Diffuse reflectance spectra of the samples were obtained by a UV-Vis spectrometer (Shimadzu UV-2600, Tokyo, Japan) with an integrating sphere reflectance accessory. UV-VIS absorption spectra were measured in the spectral range of 190 to 600 nm (Anaya-Esparza et al., Citation2021).
2.7.4. Particle size distribution and morphology by atomic force microscopy (AFM) in OβCDSL-ACGs-NSps
The particle size distribution was measured using dynamic light scattering (DLS) equipment (Malvern Instruments, Cambridge, UK) with Zetasizer software. The morphology of OβCDSL-ACGs-NSps was determined by atomic force microscopy (AFM) (Nanosurf, EasyScan2, Gräubernstrasse, Switzerland) using the static mode (contact mode) and a 10 and 70-micron head.
2.7.5. Identification of ACGs from OβCDSL-ACGs-NSps by HPLC-DAD
It was carried out employing a high-performance liquid chromatography coupled to a diode array detector (HPLC-DAD) (Agilent Technologies 1260 Infinity, Waldbronn, Germany) and an Agilent Zorbax Extend C18 reversed-phase column (250 mm x 4.6 mm, 5 µm) at 20°C. To release the ACGs present in the NSPs, 3 mL of the NSPs were shaken at 5000 rpm with a tissue tearor homogenizer (Model 985370, Biospec, Xiamen, China) for 10 min, at 2 min intervals. Subsequently, the ACGs were extracted using chloroform (5 mL). The samples were centrifuged at 3400 xg for 10 min at 4°C. The organic phase was separated, and the solvent evaporated; ACGs were resuspended in 1 mL of methanol, filtered using 0.22 µm nylon filters, and injected (10 µl) into HPLC-DAD. The separation was done using a gradient of methanol (eluent A) and water (eluent B): 0–40 min (85% A) and 40–60 min (85–95% A) with a flow rate of 1 mL/min. The ACGs were identified according to the retention times at 220 nm reported by López-Romero et al. (Citation2022) and Yang et al. (Citation2009). In addition, annonacin and pseudoannonacin were quantified using calibration curves of these standards.
2.7.6. In vitro study of the ACGs release from the OβCDSL-ACGs-NSps
The analysis was developed following the methodology employed by Hong et al. (Citation2016a) with some modifications. Quickly, 10 mL of OβCDSL-ACGs-NSps were mixed with 10 mL of a hydrochloric acid-potassium chloride buffer (0.05 M, pH 1.5) and 0.2 mL of pepsin (300 mg/mL), and the mixture was stirred and incubated for 1 h at 37 ± 1°C in a heating plate (IKA® C-MAG HS10, Wilmington, NC, U.S.A.). Subsequently, 4.5 mL of PBS (0.05 M, pH 6. 9) and 1 mL of pancreatic α-amylase (120 mg/mL) were added to the mixture. Then, the sample was maintained at 37°C in the same heating plate with continuous stirring (500 rpm) for 3 h. The mixture was transferred to a cellulose membrane for dialysis (Sigma Aldrich, St. Louis, MO, U.S.A.) and adjusted to 30 mL with PBS (0.05 M, pH 6.9). After, aliquots (500 µL) of the dialyzed samples (inside the membrane) were taken at 0, 2, 3, 4, 8, 16, 24, 28, 32 and 36 h. Aliquots were treated as mentioned before and were mixed with 2 mL of Kedde’s reagent. The absorbance was measured at 505 nm (López-Romero et al., Citation2022). The release plot was realized with the accumulative release (%) results of EquationEquation (4)(4)
(4) :
TACi is the concentration of released ACGs at the initial time (0 h), and TACt is the concentration of released ACGs at a specific time (Hong et al., Citation2016a).
2.7.7. Modeling of the ACGs release from the OβCDSL-ACGs-NSps
The ACGs release data were modeled using the zero-order, Higuchi and Korsmeyer-Peppas models (Donbrow & Samuelov, Citation1980; Higuchi, Citation1961; Korsmeyer et al., Citation1983). The mathematical interpretation of the experimental release data was performed using the DDSolver® software program (Zhang et al., Citation2010), where the results of the best-fitting kinetic model, the regression coefficient, and the release constant were obtained.
2.8. Antibacterial activity of the OβCDSL-ACGs-NSps against Enterococcus faecalis and listeria monocytogenes
The antibacterial activity was determined by the microdilution plate method employed by Pérez-Pérez et al. (Citation2020) with some modifications. Nutrient broth (100 µL) was placed in each well, and 125 µL of the OβCDSL-ACGs-NSps (98.7, 177 and 320 µg/mL) or βCD-SL complex were added in each well. Finally, 25 µL of the bacterial suspension equivalent to 1 × 106 CFU/mL (compared to McFarland standard 0.5 OD) were added to each well. The plate was incubated at 37°C, and the absorbance (545 nm) was taken each hour for 6 hours and until 12 hours. The standard antibiotic trimethoprim with sulfamethoxazole (3.2 mg/mL) was used as a positive control, while the nutrient broth was used as a negative control. The percentage of bacterial inhibition (% BI) was calculated with EquationEquation (5)(5)
(5) .
Where ACN represents the absorbance of the negative control, and AS is the absorbance of the tested sample.
2.9 Statistical analysis
The experimental design was realized in three replicates. All studies were realized in triplicate, and data were reported as mean ± standard deviation. The results of the Box-Behnken design were analyzed with RSM. The other experiments were conducted with a single-factorial experimental design. Data were analyzed by ANOVA (p < .05) with Statistic software (v.10 StatSoft, Tulsa, OK, U.S.A.). Fisher LSD test determined mean differences between treatments (p < .05).
3. Results and discussion
3.1. Particle size and polydispersity index of the βCDSL-ACGs-NSps using BBD
Statistical differences (p < .05) were observed between the treatments (). The particle size and PDI depended on each factor and their interactions. The values were within the range of 144.67 at 272.36 nm, while the PDI parameter was 0.21–0.36. The largest particle size (272.36 nm) was exhibited in NSps with 10 mg/mL XSL, 0.05% XCD and 500 rpm XPM. In contrast, the smallest particle size (144.67 nm) was obtained by adding 10 mg/mL XSL, 0.2% XCD and ultrasound XPM as a dispersion method.
Table 1. The Box-Behnken experimental design to formulate nanosuspensions (NSps) as carriers of acetogenins (βCDSL-ACGs-NSps) with the testing and predicted response variables.
Stabilizers are paramount in preparing NSps due to their relationship with the stability and final particle size of the NSps (Hong et al., Citation2016a, Citation2016b). However, the smallest particle size and PDI value are attributed to ultrasound as a dispersion method. Jakubowska et al. (Citation2021) mention that ultrasound is an effective tool for reducing the particle size and, at the same time, preserving the stability of NSps due to the generation of a significant amount of energy produced in cavitation, making a more homogeneous dispersion in the medium. It could be confirmed with the PDI values, indicating a homogeneous particle size distribution. It is essential because if this were not so, physical instability would be caused by Ostwald ripening (Gulsun et al., Citation2018).
Hong et al. (Citation2016a, Citation2017) highlight the importance of the previous formation of the amphiphilic complex because, in previous trials, they formed a complex only of hydroxypropyl-β-cyclodextrin with the bullatacin, observing larger particle sizes (230 nm). Therefore, the optimal concentrations of polymer and surfactant (as was evaluated in this work), as well as the dispersion method, help reduce the particle size and PDI that potentially increase the stability of ACGs-NSps.
3.2. Optimization of the experimental conditions to develop an βCDSL-ACGs-NSps by the response surface methodology and their validation
The effects of the independent variables (XPM, XSL, XCD) and the regression coefficients of the experimental model are shown in . The individual components exhibited a significant effect (p < .01) on particle size, while the interaction of XSL2 and XPM presented a not significant effect (p > .01). At the same time, ANOVA showed that the experimental data had a high correlation (R2 = 0.90 and adjusted R = 0.89) and an excellent fit to the models (Lack of fit p > .01), suggesting an approximation to the natural system.
Table 2. Analysis of variance and regression coefficients of polynomial quadratic models predicted with the formulation conditions of acetogenin-loaded nanosuspensions on particle size.
The resulting second-order polynomial equation describes the individual and combined effects of all independent variables on the particle size in βCDSL-ACGs-NSps, and predicted values can be calculated (EquationEquation (6)(6)
(6) ) at a confidence level of 95%.
XSL is soy lecithin, XCD is hydroxypropyl-β-cyclodextrin, and XPM is the dispersion method.
On the other hand, the response surface plots () show the effect of significant interactions (p < .05) between different polymer and surfactant concentrations on particle size. The elliptical shape of the graphs shows the interactions between the independent variables. The particle size was 138 and 181 nm, using low () or high agitation (), respectively. However, the smallest particle size (133 nm) was obtained when 10 mg/mL of SL, 0.16% of βCD () and ultrasound were applied. The Pareto diagram () shows the most significant effects on the response variables in hierarchical order, considering the absolute value of each interaction on particle size at a 95% confidence level. The main significant effects of linear and quadratic parameters were XSL2 × XPM > XPM2 >XCD × XPM.
Figure 1. Response surface plots of the particle size from nanosuspension loaded with acetogenins obtained with low agitation (500 rpm) (a), High agitation (1000 rpm) (b), ultrasound, (c) and pareto plot (d). XCD, hydroxypropyl-β-cyclodextrin (%); XSL, soy lecithin (mg/mL); XPM, dispersion method.
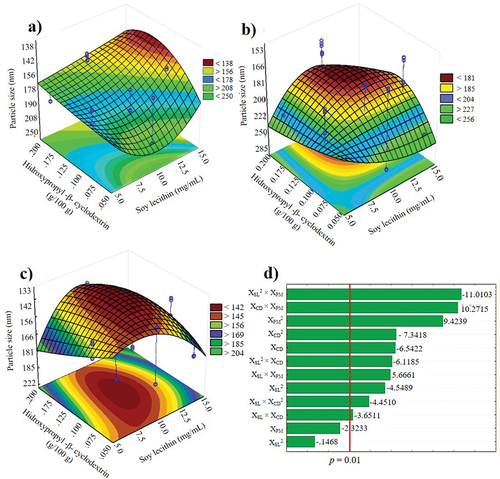
According to the optimization (), the expected particle size was 135.67 nm in an NSps prepared with 10 mg/mL soybean lecithin, 0.16% w/v βCD and employing ultrasound as the dispersion method. Furthermore, the predicted response on the particle size can range from 123.67–150.22 nm with a 95% confidence level.
Table 3. Optimal experimental conditions to formulate nanosuspensions as carriers of acetogenins (βCDSL-ACGs-NSps) and experimental validation.
3.3. Validation of the optimal conditions on physical characteristics of the OβCDSL-ACGs-NSps
The validated OβCDSL-ACGs-NSps presented a particle size of 133.37 nm (), PDI of 0.20, a zeta potential of −37 mV, 85.33% entrapment and 56.09% encapsulation efficiency. The percentage of ACGs that can bind or be trapped in the first layers or the surface of nanoparticles is known as entrapment efficiency. On the other hand, encapsulation efficiency measures the percentage of successfully encapsulated drugs in the interior or core of nanoparticles (Razura-Carmona et al., Citation2019). In this sense, the efficiency of entrapment and encapsulation are crucial to confirm the effectiveness of the NSP preparation technique. The results obtained in this research differ from those reported by Hong et al. (Citation2016a, Citation2016b, Citation2017), preparing bullatacin-NSps with stirring at 500 rpm for 15 min. The authors obtained a particle size of 144.4 nm and a zeta potential of −22.9 mV. Therefore, optimizing conditions to prepare NSPs helped to improve the particle size and stability.
3.4. Spectroscopic characterization of isolated ACGs and the OβCDSL-ACGs-NSps
3.4.1. FTIR
The FTIR spectra (4000–400 cm−1) of the ACGs, the βCD-SL complex and the OβCDSL-ACGs-NSps are shown in . The FTIR spectrum revealed the presence of deformations relative to the ketone group (C=O) at 1739 cm−1 indicating the lactone ring of the ACGs. The axial deformation of the carbon-hydrogen (−CH) bond was also observed at 1462 cm−1 and 1062 cm−1; it shows in the portion connected to the tetrahydrofuran ring, while the deformations 3318 cm−1 is assigned for O-H. The bands found at 2915 cm−1 and 2849 cm−1 (C-H) indicated the presence of long fatty acid chains characteristic of ACGs. The peaks for ACGs from FTIR spectra agree with those reported in the literature (Aguilar-Villalva et al., Citation2021; Ferreira et al., Citation2023). These results showed that the chemical structure of the ACGs was preserved in the OβCDSL-ACGs-NSps; thus, the βCD-SL complex and the dispersion method did not affect the chemical stability of these compounds.
Figure 2. Fourier-transform infrared spectroscopy (a), X-ray diffraction (b), and ultraviolet-visible diffuse reflectance spectroscopy (c) of isolated acetogenins (ACGs) and the optimized nanosuspension loaded with acetogenins (OβCDSL-ACGs-NSps) optimized nanosuspension loaded with acetogenins. βCD-SL complex, hydroxypropyl-β-cyclodextrin complexed with soy lecithin.
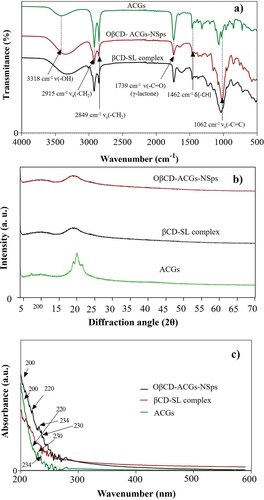
3.4.2. X-ray diffraction
ACGs showed diffraction peaks at 2θ from 19° to 21° (). On the other hand, the βCD-SL complex presented a broad peak at 2θ in the range of 15°-25°, confirming the amorphous character of these compounds, as was reported previously (Kim, Citation2020). Moreover, the XRD patterns of OβCDSL-ACGs-NSps showed only the peak belonging to the βCD-SL complex, indicating a correct encapsulation of ACGs.
3.4.3. UV-Vis diffuse reflectance spectroscopy
shows the UV-Vis spectra obtained for ACGs, βCD-SL complex and OβCDSL-ACGs-NSps. An intense band is observed around 200 nm. Studies conducted by Hidalgo et al. (Citation2019) and Aguilar-Villalva et al. (Citation2021) report that the bands found between 200–205 nm are associated with ππ* transitions due to the C=C double bond corresponding to the lactone ring of ACGs. Both peaks are observed in the spectra of ACGs and OβCDSL-ACGs-NSps. On the other hand, a well-defined peak was presented at 220 nm corresponding to hydroxypropyl-β-cyclodextrin in the spectrum of the βCD-SL complex and OβCDSL-ACGs-NSps (Hong et al., Citation2017).
3.4.4. Particle size distribution and morphology by atomic force microscopy (AFM) in OβCDSL-ACGs-NSps
The particle size distribution in the OβCDSL-ACGs-NSps is shown in with an average value of 133.37 nm. This nanometric size was corroborated in , where AFM analysis showed an average particle size of 132.4–142.2 nm. Moreover, the AFM analysis confirmed that the particles presented a spherical shape with an average height of 13 nm ().
3.4.5. Identification of ACGs in OβCDSL-ACGs-NSPs by HPLC-DAD
The chromatogram of extracted ACGs from OβCDSL-ACGs-NSps () demonstrated the existence of ACGs inside the optimized NSPs. It can be confirmed by comparing it with the ACGs chromatogram previously reported by López-Romero et al. (Citation2022). Also, the pseudoannonacin was conserved in 283 μg/mL and annonacin in 11 μg/mL.
3.5. In vitro study of the ACGs release from the OβCDSL-ACGs-NSps
The cumulative release is presented in , where ACGs were rapidly released in the first three hours, reaching 35.36% release. Then, the release was slow and constant until 16 h (45.11%) and increased again from 24 h to 36 h with 84.15% of released ACGs at the end of the kinetics. Hong et al. (Citation2016a) found the highest release of bullatacin in the first hours. The authors concluded that this occurs because a significant amount of ACGs is located in the surface layer of nanoparticles, resulting in a rapid initial release. Afterward, there is a slow release of ACGs. It is attributed to β-cyclodextrin increased the residence time of these compounds, as reported by Ahmad et al. (Citation2018), who observed that β-cyclodextrin prevents the rapid release of bioactive compounds under simulated gastric conditions, resulting in an initial quick release that subsequently slows down because this process is concentration gradient dependent.
Figure 5. Cumulative release of ACGs from the optimized nanosuspension (OβCD-ACGs-NSps) and prediction of the overall release behavior according to the Korsmeyer-Peppas model (a), bacterial inhibition of E. faecalis (b) and L. monocytogenes (c) of OβCD-ACGs-NSps. k, release constant; n, release exponent; R2, coefficient of determination; DMSO, Dimethylsulfoxide; βCD-SL, hydroxypropyl-β-cyclodextrin complexed with soy lecithin; antibiotic, trimethoprim with sulfamethoxazole (3200 μg/mL).
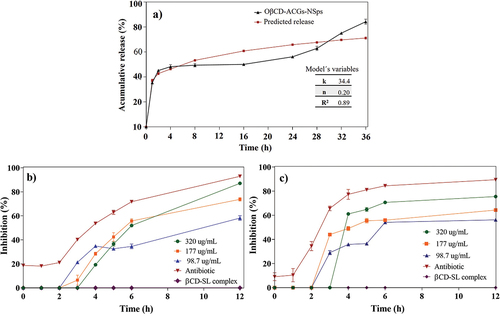
3.5.1. Modeling of the ACGs release from the OβCDSL-ACGs-NSps
The results of the correlation analysis (R2) indicate that there was no good fit with the zero-order (R2 = -0.14) and the Higuchi (R2 = 0.59) models. However, the data fit the Korsmeyer Peppas model (R2 = 0.89) (). The Korsmeyer Peppas model allows us to determine the mechanism that governs the system release. If the mechanism is by diffusion, the model corresponds to a Fickian behavior where the n value is ≤ 0.5. On the other hand, if the n value is between 0.5 and 1, it is defined as an anomalous or non-Fickian diffusion mechanism because, during the diffusion process, a viscoelastic relaxation of the polymeric chains occurred (Alhamhoom et al., Citation2023). In this study, the n value was 0.20, which indicates a Fickian diffusion by the concentration gradient.
Table 4. Acgs release kinetics for zero-order, Higuchi and Korsmeyer-Peppas model and n-value.
3.6. Antibacterial activity of OβCDSL-ACGs-NSps against Enterococcus faecalis and listeria monocytogenes
ACGs in NSps showed bacterial inhibition, which could be corroborated because the βCD-SL complex did not inhibit bacteria (). However, the inhibition depended on bacteria and the concentration of ACGs in NSPs. E. faecalis () and L. monocytogenes () were inhibited by 87% and 75% at 12 h exposition with the highest ACGs concentration. Both bacteria were inhibited to a significant percentage by NSPs using a lower concentration than positive control (3200 μg/mL).
The antibacterial activity of ACGs was reported by Aguilar-Hernández et al. (Citation2023a). The authors evaluated ACGs against E. faecalis and L. monocytogenes, finding an inhibition of 95%, using a 2000 µg/mL concentration. Therefore, it should be noted that OβCDSL-ACGs-NSps inhibited these bacteria at the lowest concentration (320 µg/mL). It is due to the nanometer size of the particle in NSPs because the active compound increases the permeability to the interior of the membrane (Omolo et al., Citation2018).
The antimicrobial mechanism of ACGs is mainly related to inhibiting mitochondrial complex I and programmed cell death (Pinto et al., Citation2017). In addition, bacterial inhibition is associated with the calcium chelation mechanism of ACGs, which affects the viability of bacteria due to the formation of pores that modify the permeability structure and functionality of the cell membrane, making it susceptible to external damage (Pinto et al., Citation2017).
4. Conclusions
This research developed a new OβCDSL-ACGs-NSps with nanometer size, uniform polydispersity index, good stability and high encapsulation percentage using optimal experimental conditions (0.16% hydroxypropyl-β-cyclodextrin, 10 mg/mL soybean lecithin, and ultrasound 40 kHz). Spectroscopic characterization ensured the presence of ACGS in the NSps with spherical nanoparticles. Moreover, the highest release of ACGs from the NSps occurred at 36 h. The OβCDSL-ACGs-NSps showed a potent antibacterial effect against E. faecalis and L. monocytogenes. These NSps could be a cost-effective alternative to encapsulate ACGs and use them in drug treatments.
Acknowledgments
The authors thank CONACYT-Mexico for the scholarship (No. 794251) awarded to Brandon A. López-Romero to carry out this project and for the postdoctoral granted to Gabriela Aguilar-Hernández (No. 590664).
Disclosure statement
No potential conflict of interest was reported by the author(s).
Additional information
Funding
References
- Aguilar-Hernández, G., López-Romero, B. A., Pérez-Larios, A., Ruvalcaba-Gómez, J. M., Castellanos-Huerta, I., Tellez-Isaias, G., Petrone-García, V. M., Anaya-Esparza, L. M., & Montalvo-González, E. (2023a). Antibacterial activity of crude extract and purified acetogenins from Annona muricata seeds. Applied Sciences, 13(1), 558. https://doi.org/10.3390/app13010558
- Aguilar-Hernández, G., López-Romero, B. A., Nicolás-García, M., Nolasco-González, Y., García-Galindo, H. S., & Montalvo-González, E. (2023b). Nanosuspensions as carriers of active ingredients: Chemical composition, development methods, and their biological activities. Food Research International, 174, 113583. https://doi.org/10.1016/j.foodres.2023.113583
- Aguilar-Hernández, G., Zepeda-Vallejo, L. G., García-Magaña, M. D. L., López-García, U. M., Aguilera-Aguirre, S., & Montalvo-González, E. (2022). Contenido de acetogeninas a partir de semillas del fruto de guanábana (Annona muricata L.): Evaluación de diferentes condiciones de extracción. Biotecnia, 24(2), 12–19. https://doi.org/10.18633/biotecnia.v24i2.1453
- Aguilar-Villalva, R., Molina, G. A., España-Sánchez, B. L., Díaz-Peña, L. F., Elizalde Mata, A., Valerio, E., Azanza-Ricardo, C., & Estevez, M. (2021). Antioxidant capacity and antibacterial activity from Annona cherimola phytochemicals by ultrasound-assisted extraction and its comparison to conventional methods. Arabian Journal Chemistry, 14(7), 103239. https://doi.org/10.1016/j.arabjc.2021.103239
- Ahmad, M., Ashraf, B., Gani, A., & Gani, A. (2018). Microencapsulation of saffron anthocyanins using β glucan and β cyclodextrin: Microcapsule characterization, release behavior & antioxidant potential during in-vitro digestion. International Journal of Biological Macromolecules, 109, 435–442. https://doi.org/10.1016/j.ijbiomac.2017.11.122
- Akhmad, E. Z. H., Heddy, J., Nurliani, B., Eny, I. R., & Fahru, R. A. (2022). Soursop leaves (Annona muricata L.) endophytic fungi anticancer activity against HeLa cells. Saudi Journal of Biological Sciences, 29(8), 103354. https://doi.org/10.1016/j.sjbs.2022.103354
- Alhamhoom, Y., Honmane, S. M., Hani, U., Osmani, R. A. M., Kandasamy, G., Vasudevan, R., Paramshetti, S. R., Dudhal, R. K., Kengar, N., & Charde, M. S. (2023). Study of formulation and process variables for optimization of piroxicam nanosuspension using 32 factorial design to improve solubility and in vitro bioavailability. Polymers, 15(3), 483. https://doi.org/10.3390/polym15030483
- Anaya-Esparza, L. M., Ruvalcaba-Gómez, J. M., Romero-Toledo, R., Sánchez-Burgos, J. A., Montalvo-González, E., & Pérez-Larios, A. (2021). Investigating structural changes of Chitosan-TiO2 and Chitosan-Ti-ZnO-MgO hybrid films during storage by FTIR spectroscopy. Macedonian Journal of Chemistry and Chemical Engineering, 40(2), 197–211. https://doi.org/10.20450/mjcce.2021.2396
- Bermejo, A., Figadère, B., Zafra-Polo, M. C., Barrachina, I., Estornell, E., & Cortes, D. (2005). Acetogenins from Annonaceae: Recent progress in isolation, synthesis and mechanisms of action. Natural Product Reports, 22(2), 269–303. https://doi.org/10.1039/B500186M
- Chamundeeswari, M., Jeslin, J., & Verma, M. L. (2018). Nanocarriers for drug delivery applications. Environmental Chemistry Letters, 17(2), 849–865. https://doi.org/10.1007/s10311-018-00841-1
- Coria-Téllez, A. V., Montalvo-González, E., Yahia, E. M., & Obledo-Vázquez, E. N. (2018). Annona muricata: A comprehensive review on its traditional medicinal uses, phytochemicals, pharmacological activities, mechanisms of action and toxicity. Arabian Journal Chemistry, 11(5), 662–691. https://doi.org/10.1016/j.arabjc.2016.01.004
- Donbrow, M., & Samuelov, Y. (1980). Zero order drug delivery from double-layered porous films: Release rate profiles from ethyl cellulose, hydroxypropyl cellulose and polyethylene glycol mixtures. Journal of Pharmacy and Pharmacology, 32(1), 463–470. https://doi.org/10.1111/j.2042-7158.1980.tb12970.x
- Ferreira, G. G., Quaresma, A. C. S., Brandão, D. L. D. N., Marinho, A. M. D. R., Siqueira, J. E. D. S., Correa, K. L., Silva-Júnior, J. O. C., Percario, S., & Dolabela, M. F. (2023). Evaluation of genotoxicity and toxicity of Annona muricata L. seeds and in silico studies. Molecules, 28(1), 231. https://doi.org/10.3390/molecules28010231
- Gulsun, T., Borna, S. E., Vural, I., & Sahin, S. (2018). Preparation and characterization of furosemide nanosuspensions. Journal of Drug Delivery Science and Technology, 45, 93–100. https://doi.org/10.1016/j.jddst.2018.03.005
- Gutiérrez, M. T., Durán, A. G., Mejías, F. J. R., Molinillo, J. M. G., Megias, D., Valdivia, M. M., & Macías, F. A. (2020). Bio-guided isolation of acetogenins from Annona cherimola deciduous leaves: Production of nanocarriers to boost the bioavailability properties. Molecules, 25(20), 4861. https://doi.org/10.3390/molecules25204861
- Han, J., Zhou, X., Fu, J., Gao, G., Zou, C., Guo, Y., Han, M., & Wang, Y. (2021). Annonaceous acetogenin nanosuspensions stabilized by poloxamer 188: Preparation, properties and in vivo evaluation. Journal of Drug Delivery Science and Technology, 66, 102676. https://doi.org/10.1016/j.jddst.2021.102676
- Hidalgo, J. R., Neske, A., Iramain, M. A., Alvarez, P. E., Bongiorno, P. L., & Brandán, S. A. (2019). FT-IR, FT-Raman and UV-visible spectra of motrilin acetogenin isolated from annona cherimolia. Journal of Molecular Structure, 1196, 508–517. https://doi.org/10.1016/j.molstruc.2019.06.107
- Higuchi, T. (1961). Rate of release of medicaments from ointment bases containing drugs in suspension. Journal of Pharmaceutical Sciences, 50(10), 874–875. https://doi.org/10.1002/jps.2600501018
- Hong, J., Li, Y., Xiao, Y., Li, Y., Guo, Y., Kuang, H., & Wang, X. (2016a). Annonaceous acetogenins (ACGs) nanosuspensions based on a self-assembly stabilizer and the significantly improved anti-tumor efficacy. Colloids Surfaces B Biointerfaces, 145, 319–327. https://doi.org/10.1016/j.colsurfb.2016.05.012
- Hong, J., Li, Y., Li, Y., Xiao, Y., Kuang, H., & Wang, X. (2016b). Annonaceous acetogenins nanosuspensions stabilized by PCL-PEG block polymer: Significantly improved antitumor efficacy. International Journal of Nanomedicine, 11, 3239–3253. https://doi.org/10.2147/IJN.S108143
- Hong, J., Sun, Z., Li, Y., Guo, Y., Liao, Y., Liu, M., & Wang, X. (2017). Folate-modified Annonaceous acetogenins NSps and their improved antitumor efficacy. International Journal of Nanomedicine, 12, 5053–5067. https://doi.org/10.2147/ijn.s134284
- Jakubowska, E., Milanowski, B., & Lulek, J. A. (2021). A systematic approach to the development of cilostazol nanosuspension by liquid antisolvent precipitation (LASP) and its combination with ultrasound. International Journal of Molecular Sciences, 22(22), 12406. https://doi.org/10.3390/ijms222212406
- Kim, J. C. (2020). Study of flavonoid/hydroxypropyl-β-cyclodextrin inclusion complexes by UV-VIS, FT-IR, DSC, and X-ray diffraction analysis. Preventive Nutrition and Food Science, 25(4), 449–456. https://doi.org/10.3746/pnf.2020.25.4.449
- Kim, S. H., Lee, E. S., Lee, K. T., & Hong, S. T. (2021). Stability properties and antioxidant activity of curcumin nanosuspensions in emulsion systems. CyTA-Journal of Food, 19(1), 40–48. https://doi.org/10.1080/19476337.2020.1852315
- Korsmeyer, R. W., Gurny, R., Doelker, E., Buri, P., & Peppas, N. A. (1983). Mechanism of solute release from porous hydrophilic polymers. International Journal of Pharmaceutical Sciences, 15(1), 25–35. https://doi.org/10.1016/0378-5173(83)90064-9
- León-Fernández, A. E., Martínez, L., Zepeda-Vallejo, L. G., Arteaga-Garibay, R. I., Gutiérrez-Martínez, P., & Montalvo-González, E. (2019). Antibacterial, antifungal, antioxidant and toxic effect of fractioned extracts from soursop pulp. Revista Bio Ciencias, 6, 400. https://doi.org/10.15741/revbio.06e400
- Li, H., Li, Y., Ao, H., Bi, D., Han, M., Guo, Y., & Wang, X. (2018). Folate-targeting annonaceous acetogenins nanosuspensions: Significantly enhanced antitumor efficacy in HeLa tumor-bearing mice. Drug Delivery, 25(1), 880–887. https://doi.org/10.1080/10717544.2018.1455761
- López-Romero, B. A., Luna-Bárcenas, G., García-Magaña, M. D. L., Anaya-Esparza, L. M., Zepeda-Vallejo, L. G., López-García, U. M., Ortiz-Basurto, R. I., Aguilar-Hernández, G., Pérez-Larios, A., & Montalvo-González, E. (2022). Extraction of acetogenins using thermosonication-assisted extraction from Annona muricata seeds and their antifungal activity. Molecules, 27(18), 6045. https://doi.org/10.3390/molecules27186045
- Ma, Y., Cong, Z., Gao, P., & Wang, Y. (2023). Nanosuspensions technology as a master key for nature products drug delivery and in vivo fate. European Journal of Pharmaceutical Sciences, 185, 106425. https://doi.org/10.1016/j.ejps.2023.106425
- Omolo, C. A., Kalhapure, R. S., Agrawal, N., Rambharose, S., Mocktar, C., & Govender, T. (2018). Formulation and molecular dynamics simulations of a fusidic acid nanosuspension for simultaneously enhancing solubility and antibacterial activity. Molecular Pharmaceutics, 15(8), 3512–3526. https://doi.org/10.1021/acs.molpharmaceut.8b00505
- Pérez-Pérez, J. U., Guerra-Ramírez, D., Reyes-Trejo, B., Cuevas-Sánchez, J. A., & Guerra-Ramírez, P. (2020). Actividad antimicrobiana in vitro de extractos de Jatropha dioica Seseé contra bacterias fitopatógenas de tomate. Polibotánica, 49(49), 125–133. https://doi.org/10.18387/polibotanica.49.8
- Pinto, N. C. C., Campos, L. M., Evangelista, A. C. S., Lemos, A. S. O., Silva, T. P., Melo, R. C. N., & Fabri, R. L. (2017). Antimicrobial Annona muricata L. (soursop) extract targets the cell membranes of gram-positive and gram-negative bacteria. Industrial Crops and Products, 107, 332–340. https://doi.org/10.1016/j.indcrop.2017.05.054
- Razura-Carmona, F. F., Pérez-Larios, A., González-Silva, N., Herrera-Martínez, M., Medina-Torres, L., Sáyago-Ayerdi, S. S., & Sánchez-Burgos, J. A. (2019). Mangiferin-loaded polymeric nanoparticles: Optical characterization, effect of anti-topoisomerase I, and cytotoxicity. Cancers, 11(12), 1965. https://doi.org/10.3390/cánceres11121965
- Shetab-Boushehri, M. A., Dietrich, D., & Lamprecht, A. (2020). Nanotechnology as a platform for the development of injectable parenteral formulations: A comprehensive review of the know-how and state of the art. Pharmaceutics, 12(6), 510. https://doi.org/10.3390/pharmaceutics12060510
- Venugopal, V., Kumar, K. J., Muralidharan, S., Parasuraman, S., Raj, P. V., & Kumar, K. V. (2016). Optimization and in-vivo evaluation of isradipine nanoparticles using box-behnken design surface response methodology. OpenNano, 1, 1–15. https://doi.org/10.1016/j.onano.2016.03.002
- Yang, H. J., Zhang, N., Chen, J. W., & Wang, M. Y. (2009). Two new cytotoxic acetogenins from Annona squamosa. Journal of Asian Natural Products Research, 11(3), 250–256. https://doi.org/10.1080/10286020802682916
- Zhang, Y., Huo, M., Zhou, J., Zou, A., Li, W., Yao, C., & Xie, S. (2010). Ddsolver: An add-in program for modeling and comparison of drug dissolution profiles. The AAPS Journal, 12(3), 263–271. https://doi.org/10.1208/s12248-010-9185-1