ABSTRACT
The development of colorectal cancer typically involves the accumulated influences of genetic alterations, medical issues, lifestyle, and diet. Dietary fatty acids appear to affect the tumorigenesis and progression of colorectal cancer. Despite conflicting results, the current consensus on the effects of very long-chain polyunsaturated fatty acids on colorectal cancer is that low levels of eicosapentaenoic acid and docosahexaenoic acid, and high levels of arachidonic acid are associated with an increased risk of colorectal cancer. Altered levels of arachidonic acid in membrane phospholipids can change the levels of prostaglandin E2, which affect the biological activities of cancer cells in multiple stages. Arachidonic acid and other very long-chain polyunsaturated fatty acids can affect tumorigenesis in prostaglandin E2-independent manners as well, including stabilization of β-catenine, ferroptosis, ROS generation, regulation of transcription factors, and de novo lipogenesis. Recent studies have revealed an association between the activities of enzymes synthesizing very long-chain polyunsaturated fatty acids and tumorigenesis and cancer progression, although the mechanisms are still unknown. In this study, PUFA effects on tumorigenesis, the endogenous very long-chain polyunsaturated fatty acid synthesis pathway, metabolites of arachidonic acid and their effects on tumorigenesis and progression of CRC, and current knowledge that supports the association of the enzymes involved in the polyunsaturated fatty acid synthesis pathway with colorectal cancer tumorigenesis and progression are reviewed.
Introduction
Colorectal cancer (CRC) is the third most common cancer and second most common cause of cancer-related death globally (WHO Citation2022). Accumulation of various factors accounts for most cases of CRC. These factors include genetic alterations; medical issues, such as a family history of CRC, inflammatory bowel disease, obesity, and diabetes. Smoking, excessive alcohol consumption, physical activity, and dietary factors that include meat, vegetables, dietary fiber, and fish consumption are also critical factors in the development of CRC (Thanikachalam and Khan Citation2019; Li et al. Citation2021).
Dietary fats, especially dietary fatty acids, appear to affect he tumorigenesis and progression of CRC. Epidemiological studies have suggested that saturated fatty acids and ω-6 very long chain (VL)-polyunsaturated fatty acids (PUFAs) may enhance colorectal carcinogenesis, whereas ω-3 VL-PUFAs may have protective effects (Van Blarigan et al. Citation2018), although more studies are still needed to establish their effects and the mechanisms involved. Over the last decade, whole genome analysis and transcriptomic studies using CRC tissues and cell lines have revealed alterations in gene expression related to VL-PUFA synthesis and metabolism during tumorigenesis and progression of CRC, implicating these genes as therapeutic targets for cancer treatment, as well as markers to assess the severity of cancer or prognosis.
This review overviews PUFA effects on tumorigenesis, the endogenous VL-PUFA synthesis pathway and enzymes, metabolites of arachidonic acid and their effects on tumorigenesis and progression of CRC, expression changes of genes involved in VL-PUFA synthesis and metabolism, and epigenetic regulation of elongase of very long fatty acid 5 (ELOVL5) in cancer.
Effects of PUFAs on tumorigenesis
Linoleic (C18:2, ω-6), α-linolenic acid (C18:3, ω-3), arachidonic acid (C20:4, ω-6), eicosapentaenoic acid (EPA, C20:5, ω-3), and docosahexaenoic acid (DHA, C22:6, ω-3) are the major components of membrane phospholipids. VL-PUFAs with more than 20 carbons (C20) also have various biological activities. They are ligands for nuclear receptors, including peroxisome proliferator-activated receptors and liver X receptors (LXRs) (Chambrier et al. Citation2002; Yoshikawa et al. Citation2002; Bordoni et al. Citation2006). VL-PUFAs are also regulators of transcription factors, such as sterol regulatory element binding protein-1 (SREBP-1) and carbohydrate element binding protein (ChREBP) (Hannah et al. Citation2001; Dentin et al. Citation2006). Their metabolites, which include eicosanoids and resolvins, act as signaling molecules in various physiological and pathological processes. Because of these essential roles, deficiencies in PUFAs result in disease conditions (Spector and Kim Citation2015). Conversely, many clinical and epidemiologic studies have revealed that VL-PUFAs, especially in the form of ω-3, which is rich in fish oil, have beneficial effects on the prevention and improvement of cardiovascular and metabolic diseases (Burns et al. Citation2018). The effects of VL-PUFAs on inflammation and cancer have not yet been clearly defined, and various studies have reported both pro-/anti-inflammatory and pro-/anti-tumorigenic roles (Hardman Citation2004; Hall et al. Citation2008; Calder Citation2017; Burns et al. Citation2018; Innes and Calder Citation2018; Bae et al. Citation2020; Dierge et al. Citation2021).
Population-based studies designed to determine the association between FA intake and CRC have demonstrated conflicting results, including a lack of association (Sasazuki et al. Citation2011; Sakai et al. Citation2012; Hodge et al. Citation2015). The influence of genetic variations in genes related to prostanoid synthesis, such as prostaglandin-endoperoxide synthase (PTGS) 1, PTGS2, arachidonate lipoxygenase (ALOX) 12, ALOX5, ALOX15, and 5-lipoxygenase activating protein, has been proposed as a reason for the inconsistent results of dietary VL-PUFAs on CRC risk (Habermann et al. Citation2013). Currently, the consensus on VL-PUFA on CRC seems to be that low levels of EPA and DHA, and high levels of arachidonic acid are associated with an increased risk of CRC (Pot et al. Citation2008). Similarly, dietary supplementation with ω-3 PUFA and ω-6 PUFA suppresses and enhances, respectively, the risk of CRC (Hardman Citation2004; Hall et al. Citation2008; Sasazuki et al. Citation2011; May-Wilson et al. Citation2017). Arachidonic acid seems to increase colon cancer cell growth, whereas DHA acts in another way (Habbel et al. Citation2009). Therefore, supplementation of ω-3 PUFA has been used in cancer treatment to minimize resistance and improve the efficacy of radiotherapy and chemotherapy (Hardman Citation2004; Dupertuis et al. Citation2007). One of the ways in which ω-6 arachidonic acid affects colon cancer cells may be mediated by the production of prostaglandins (PGs), whereas ω-3 PUFAs inhibit PG production from ω-6 PUFA (Dupertuis et al. Citation2007; Habbel et al. Citation2009). Independent of PG production, arachidonic acid could be pro-tumorigenic by stabilizing β-catenin through direct interaction with Fas-associated factor 1 (FAF1), thus stimulating tumor growth (Kim et al. Citation2015). The peroxidation of PUFAs induces oxidative stress in cells, which can be pro-inflammatory and pro-carcinogenic (Cai et al. Citation2012). In contrast, peroxidation of PUFAs can be cytotoxic and tumor-suppressive in CRC cells (Dupertuis et al. Citation2007). VL-PUFAs of both ω-6 and ω-3 can mediate anti-cancer effects through ferroptosis, an iron-dependent form of programed cell death (Dixon et al. Citation2012) characterized by the accumulation of lipid peroxides (Dierge et al. Citation2021). The conditions and mechanisms of the effects of ω-6 and ω-3 PUFAs on CRC are unclear, and further studies are required.
Generation of endogenous VL-PUFAs
Linoleic and α-linolenic acids are essential fatty acids with 18 carbons. They cannot be synthesized in mammalian cells and must be supplied in the diet (Spector and Kim Citation2015). Longer chain PUFAs with more double bonds than linoleic and α-linolenic acid in the ω-6 and ω-3 series can be generated through reactions that are performed by FA elongation and desaturation systems on the endoplasmic reticulum (ER) membrane in cells (Cinti et al. Citation1992). Arachidonic acid, EPA, and DHA are representative VL-PUFAs generated from linoleic and α-linolenic acid through these systems (Spector and Kim Citation2015).
FAs longer than 18 carbons are generated by the FA elongation system on the ER membrane () (Cinti et al. Citation1992). This system adds two carbons to preexisting fatty acyl-CoA through a cycle of four consecutive reactions of condensation, reduction, dehydration, and reduction. Each reaction is catalyzed by a separate enzyme. Condensation between malonyl-CoA and preexisting fatty acyl-CoA to generate β-ketoacyl-CoA is catalyzed by ELOVLs. Next, β-ketoacyl reductase, also known as 17-beta hydroxysteroid dehydrogenase 12 (HSD17B12), catalyzes the generation of β-hydroxyacyl-CoA from β-ketoacyl-CoA using NADPH as a reducing cofactor. Next, trans-2,3-enoly-CoA is generated by the catalytic action of dehydratase, followed by another reduction catalyzed by trans-2,3-enoly-CoA reductase (TECR) using NADPH to generate the final 2-carbon elongated fatty acyl-CoA (Cinti et al. Citation1992). In this cycle, the condensation step catalyzed by ELOVLs is the rate-limiting step. Seven ELOVLs (ELOVL1, 2, 3, 4, 5, 6, and 7) exist in mammals (Jump Citation2009). Each ELOVL exhibits substrate specificity for fatty acyl-CoA with different chain lengths and numbers of double bonds, and is expressed in a tissue-specific manner. However, other enzymes that comprise the elongation system seem to possess no substrate specificity and are ubiquitously expressed in all tissues (Moon and Horton Citation2003).
Figure 1. Fatty acid elongation system. Fatty acids longer than C18 are elongated through the fatty acid elongation system on the endoplasmic reticulum membrane. R represents the fatty acyl chain.
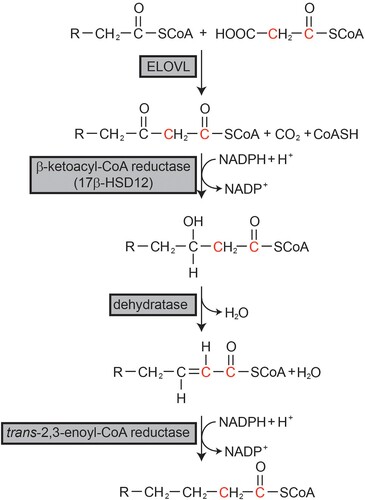
To generate arachidonic acid, EPA, and DHA from linoleic and α-linolenic acid, ELOVL2, ELOVL5, fatty acid desaturase 1 (Δ5 desaturase; FADS1), and FADS2 (Δ6 desaturase) involve the reactions (). Linoleic and α-linolenic acids are reduced to γ-linolenic acid and stearidonic acid by FADS2, followed by elongation to dihomo- γ-linolenic acid (C20:3, ω-6) and ETA (C20:4, ω-3) by ELOVL5. Following reduction by FADS1, arachidonic acid and EPA are generated. ELOVL2 is involved in the elongation of PUFAs longer than C20 and C22, and peroxisomal degradation may additionally occur to generate DHA (Jakobsson et al. Citation2006) (). The importance of ELOVL5 in maintaining VL-PUFA in the cell was demonstrated in Elovl5 knock out mice. The lack of ELOVL5 resulted in a reduction in cellular VL-PUFA content, and the phospholipid class displayed the greatest change (Moon et al. Citation2009).
Metabolites of arachidonic acid and effects on cancer
Arachidonic acid is a precursor of C20 eicosanoids. Arachidonic acid at the sn-2 position of membrane phospholipids is released by phospholipase A2 and can be oxidized by the catalytic actions of cyclooxygenase (COX, also known as prostaglandin-endoperoxide synthase [PTGS]), P-450 epoxygenase, and lipoxygenase (LOX) (Patel et al. Citation2008). The COX pathway generates five primary prostanoids that include prostaglandin (PG) D2, PGE2, PGF2α, PGI2, and thromboxane A2, which are signaling molecules with short half-lives that act in a paracrine or autocrine manner (Patel et al. Citation2008). Prostanoids play important roles in general physiological and pathological processes, including inflammation, fever, smooth muscle constriction and relaxation, vascular constriction and relaxation, platelet aggregation, ovulation, and labor. The two major isoforms of COX (COX-1 and COX-2) act in the same fashion to generate prostanoids, but with different expression patterns in humans. COX-1 is constitutively expressed in most tissues, whereas COX-2 expression is induced in certain pathological conditions. Selective inhibition of COX-2 can reduce the side effects generated by inhibiting the physiological processes performed by COX-1 (Patel et al. Citation2008).
The eicosanoids produced are secreted into the extracellular microenvironment and bind to their specific receptors on the plasma membrane. PGD2 binds to prostaglandin D2 receptor (DP), PGE2 to prostaglandin E2 receptors (Eps), PGF2α to prostaglandin F2α receptor (FP), PGI2 to prostacyclin receptors (IPs), and TXA2 to thromboxane receptor (TBXA2R). Generally, PG pathways contribute to tumorigenesis by mediating cell proliferation, growth, apoptosis, invasion, migration, metastasis, and angiogenesis. Thus, PGs are critical mediators of cancer (Wang et al. Citation2015).
PGE2 is one of the major PGs produced by COX protein and the most abundant PG found in CRC (Cai et al. Citation2006; Wang et al. Citation2015). PGE2 binds to its specific receptors, EP1–EP4. These are G-protein-coupled receptors that transfer their signals via different signaling pathways. G proteins coupled with EPs give rise to second messengers that include cAMP, Ca2+, and inositol triphosphate (IP3) to initiate downstream signaling often associated with tumor growth and metastasis. The signaling includes the phosphoinositide 3-kinase (PI3K), mitogen-activated protein kinase (MAPK) pathway, NF-κB, and β-catenin/T-cell factor signaling (Wang et al. Citation2022). PGE2 effects on cancer cell proliferation and metastasis have been studied in cultured cells and in various types of human cancers, including CRC, breast cancer, lung cancer, liver cancer, gastrointestinal cancer, pancreatic cancer, and renal and urinary cancer (Jara-Gutiérrez and Baladrón Citation2021). PGE2 affects multiple stages of cancer, including carcinogenesis, tumor cell proliferation, invasion, and interaction between tumor and immune cells (Wang et al. Citation2015).
Chronic inflammation is an important risk factor for the development of CRC (Ogino et al. Citation2011; Cai et al. Citation2012). COX-2 expression is upregulated during inflammation and PGE2 synthesis is increased, which can alter cytokine balance and expression of PG receptors, as well as activate cell proliferation (Mutoh et al. Citation2006). PGE2 levels and expression of COX-2 are also increased in colon tumors compared with the surrounding normal tissue in mouse models and human patients (Sano et al. Citation1995). In the colon, PGE2 has been associated with polyp formation and tumorigenesis (Mutoh et al. Citation2006). In a rat colon tumor model induced by azoxymethane, the addition of PGE2 for 25 weeks significantly increased the incidence of tumors, suggesting that PGE2 is involved in tumorigenesis and that inhibition of the receptors or PGE2 synthesis can be targets for the prevention and treatment of CRC (Wang et al. Citation2005; Mutoh et al. Citation2006). Experimental evidence of the involvement of PGE2 in tumorigenesis and cancer metastasis has led to the use of COX inhibitors, including aspirin and nonsteroidal anti-inflammatory drugs (NSAIDs), to reduce PGE2 and produce anti-cancer effects (Dannenberg et al. Citation2001). Randomized controlled trials demonstrated that regular use of aspirin for >20 years could reduce the long-term incidence and mortality of CRC (Rothwell et al. Citation2010). Regular use of nonselective NSAIDs and selective COX-2 inhibitors over 10–15 years reduced the development of colorectal cancer by 40%–50%, and reduced the number and size of adenomas in patients with familial adenomatous polyposis (Wang et al. Citation2005). While NSAIDs reduced the risk of CRC associated with COX-2 overexpression, no effect was observed in CRC with weak or absent expression of COX-2 (Chan et al. Citation2007). Selective COX-2 inhibitors have been included in therapeutic strategies, either as a prophylactic or adjuvant treatment for chemotherapy or radiotherapy (Hashemi Goradel et al. Citation2019). These results suggest that PGE2 is important in CRC, and that the level of precursor arachidonic acid might affect PGE2 production.
Enzymes involved in VL-PUPA synthesis in CRC
Reprograming of cellular metabolic processes is a feature of cancer cells that leads to changes in enzymes involved in de novo lipogenesis and FA profiles (Peck and Schulze Citation2016). Cellular changes occurring upon alteration in arachidonic acid content in membrane phospholipids have been demonstrated in endothelial cells. In the study, arachidonic acid content affected the viscosity of the cell membrane and thus cellular motion, and regulated cell adhesion and migration (Rossen et al. Citation2011). The increased arachidonic acid content in phosphatidylinositol at the cancer cell/stromal cell interface in CRC patients may imply the influence of arachidonic acid content in cancer progression (Hiraide et al. Citation2016). Moreover, arachidonic acid in membrane phospholipids is a source of PGE2, which affects multiple stages of cancer. As described earlier, VL-PUFAs other than arachidonic acid can also affect CRC. Therefore, changes in the activities of enzyme components in the VL-PUFA synthesis pathway could influence CRC cells in various ways, including PGE2-dependent and -independent manners. An inclusive list of published data of the association of ELOVL5, FADS2, and HSD17B12 with CRC and other types of cancers are shown in , respectively.
Table 1. Association of genes in VL-PUFA synthesis with human colorectal cancer (CRC).
Table 2. Association of genes in VL-PUFA synthesis with human cancers other than CRC.
ELOVL5: ELOVL5 activity is essential for maintaining VL-PUFA levels in phospholipids, as shown in a study involving Elovl5−/− mice (Moon et al. Citation2009). Therefore, changes in the expression of ELOVL5 in cancer cells would change the VL-PUFA profile and affect the proliferation and progression of cancer cells. According to a Gene Expression Omnibus (GEO) profile (GDS4296) using various CRC cell lines, COLO205, HCC 2998, HCT116, KM12, and SW 620 cells exhibited high expression of ELOVL5, whereas ELOVL5 expression was negligible in HCT 15 and HT 29 cells ((A)). ELOVL5 expression in CRC is inversely associated with the prognosis of patients with CRC. The Cancer Genome Atlas (TCGA) survival data that linked ELOVL5 expression level with overall survival of patients with colon adenocarcinoma revealed better survival rates for patients with low expression of ELOVL5 (<10th percentile) compared to patients with high expression of ELOVL5 (>50th percentile) ((B)). Genome analysis has demonstrated increased expression of ELOVL5 in human CRC and other types of cancers, including breast, prostate, and kidney cancers. The findings suggest the involvement of ELOVL5 in cancer cell proliferation and invasion, although its effects and mechanisms remain unclear. Study data on the association between ELOVL5 expression and cancers are presented in .
Figure 3. Association of ELOVL5 with colorectal cancer (CRC). (A) ELOVL5 expression in the indicated CRC cell lines (GEO profile GDS4296). (B) TCGA survival data that link ELOVL5 expression level with overall survival of the patients of colon adenocarcinoma shows that the patients with low expression of ELOVL5 (<10th percentile) showed a better survival rate than those with high expression of ELOVL5 (>50th percentile).
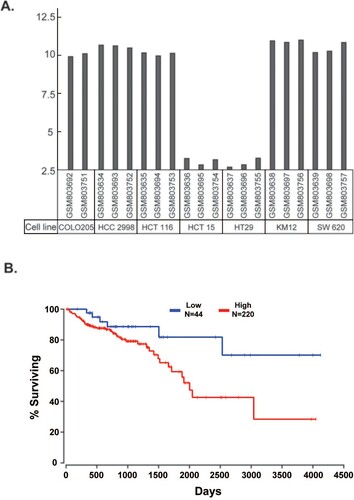
Gene expression analysis of CRC using GEO dataset GSE20931 showed that the expression of ELOVL5, FADS2, HSD17B12, and trans-2,3-enoyl-CoA reductase (TECR), the enzymes involved in PUFA elongation and desaturation, were significantly increased in colon cancer samples compared to the normal tissue. The findings suggest that changes in PUFA contents could affect cancer cell behavior (Mokhtari et al. Citation2022). In another study, changes in the expression of genes involved in de novo FA and VL-PUFA synthesis were detected in cultured colon cancer cells. Increased expression of fatty acid synthase, stearoyl-CoA desaturase, FADS2, and ELOVL5 in tumor epithelial cells was correlated with changes in fatty acid contents of cellular phospholipids, which reflected the increased activities of ELOVL5/2 and FADS2 in these tumor cells (Hofmanová et al. Citation2021). One of the mechanisms that regulates ELOVL5 activity may be related to genetic variation. A genome-wide analysis found an association between the single nucleotide polymorphisms (SNP) near 6p12.1/ELOVL5 gene and survival outcomes in patients with distant metastatic CRC. The SNP, rs2309489, exhibited the strongest association with poor survival rate. However, the role of genetic variation in CRC has not been elucidated, and the use of the SNP as a prognostic marker has not yet been determined (Phipps et al. Citation2015). Upregulation of enzymes involved in PUFA elongation has also been reported in other types of cancer. In a study that compared gene expression in 74 breast cancer tissues with that in normal breast tissues, ELOVL1, 5, and 6 were detected as genes that were upregulated in tumors (Yamashita et al. Citation2017).
Mechanisms of how ELOVL5 activity affects cancer cells have been suggested in a few studies performed in prostate cancer, renal cell cancer, and gastric cancer cells. In prostate cancer cells, ELOVL5 expression was induced by androgen, and increased expression of ELOVL5 in prostate cancer was demonstrated in cultured cells, xenografts, and clinical tumors of prostate cancer (Centenera et al. Citation2021). Upon depletion of ELOVL5 in prostate cancer cells, the cells exhibited morphological and functional changes in the mitochondria, resulting in the excess generation of ROS to kill the cells (Centenera et al. Citation2021). In renal cell cancer, higher levels of ELOVL5 correlate with poor clinical prognosis, and ELOVL5 seems to lead to cancer cell proliferation and invasion (Nitta et al. Citation2022). FA changes due to the increased activity of ELOVL5 have been related to increased cell proliferation and invasion (Nitta et al. Citation2022). Protein kinase B-mammalian target of rapamycin-signal transducer and activator of transcription 3 (AKT-mTOR-STAT3) signaling through AKT Ser473 phosphorylation has been suggested as a mechanism. However, more studies are needed to determine how these lipid changes affect signaling pathways.
Recently, ferroptosis has emerged as a mechanism of programed cell death. Ferroptosis is characterized by the accumulation of peroxided VL-PUFAs. Some gastric cancer cells exhibit differential expression of ELOVL5 and FADS1 through changes in DNA methylation of their promoter regions. ELOVL5 expression is associated with the levels of cellular arachidonic acid and adrenic acid (C22:4, ω-6) in cancer cells, which are subjected to lipid peroxidation and ferroptosis (Lee et al. Citation2020). As the ferroptosis pathway is a possible target for cancer treatment (Dierge et al. Citation2021), ELOVL5 could be a marker for determining whether ferroptosis-mediated treatment is applicable (Lee et al. Citation2020). The association between ferroptosis sensitization and differential expression of ELOVL5 needs to be determined to indicate its’ therapeutic feasibility in CRC.
Contrary to the results presented above, a study related the low expression of ELOVL5 and insulin-like growth factor binding protein 6 (IGFBP6) with pronounced metastasis in breast cancer (Nikulin et al. Citation2021). Inhibition of the ELOVL5 and IGFBP6 genes in breast cancer cells results in the increased expression of matrix metalloproteinase 1 and reduction of intercellular contacts. These changes in turn result in a more efficient invasion of tumor cells and higher probability of increased metastasis (Nikulin et al. Citation2021).
The studies presented above suggest that the cellular VL-PUFA composition and the activities of the synthesis pathway could play important roles in the development and progression of cancer. Although the functions of ELOVL5 and its mechanisms in tumorigenesis are still unknown, ELOVL5 could be a possible diagnostic and prognostic marker and a targetable molecule for CRC treatment.
FADS2: FADS2, a Δ-6 desaturase, performs the first rate-limiting step in the endogenous pathway to synthesize arachidonic acid from C18 PUFA () (Tang et al. Citation2003). The possible involvement of FADS2 in the pathogenesis of breast cancer has been suggested. Along with increased FDAS2 activity, levels of metabolites from linoleic acid, such as C18:3, ω-6, C20:3, ω-6, and arachidonic acid, as well as PGE2 levels, are reportedly increased in cancerous tissue compared to adjacent noncancerous tissue in breast tumors (Pender-Cudlip et al. Citation2013). These results may lead to similar effects of increased ELOVL5 expression, which leads to increased arachidonic acid production due to the greater conversion from linoleic acid. The correlation between FADS2 expression and PGE2 levels suggests that the enzyme activity toward arachidonic acid synthesis could affect PGE2 levels and, thus, tumorigenesis (Pender-Cudlip et al. Citation2013).
HSD17B12: HSD17B12, or β-ketoacyl-CoA reductase, is the enzyme involved in the second step of the FA elongation cycle () (Moon and Horton Citation2003). Therefore, overall FA elongation and cellular FA profiles can be affected by HSD17B12 activity. However, the enzyme’s mechanism in tumorigenesis is unknown; several studies have reported an association between HSD17B12 expression and cancer outcomes.
Higher expression of HSD17B12 was detected in colorectal tumor tissues, suggesting a possible correlation between its activity and CRC. Functional genetic variants of HSD17B12 are correlated with the outcome of CRC (Lin et al. Citation2020). A Cox regression model that evaluated the genetic effects on CRC overall survival and progression-free survival revealed that rs10838164 C>T in HSD17B12 was significantly associated with an increased risk of CRC progression and death. The T allele can increase HSD17B12 expression by enhancing the binding affinity of transcription factors to promote the transcriptional activity of the HSD17B12 gene (Lin et al. Citation2020).
Changes in the expression of HSD17B12 have been reported in ovarian and breast cancers (Kemiläinen et al. Citation2018; Tsachaki et al. Citation2020). High expression of HSD17B12, along with increased COX-2 expression, is associated with high-grade epithelial ovarian cancer (Kemiläinen et al. Citation2018). The expression level of HSD17B12 correlated with the severity of ovarian cancer, and its expression mimicked COX-2 expression, indicating its role in increased arachidonic acid and PGE2 production during ovarian cancer progression (Kemiläinen et al. Citation2018). High immunoreactivity in breast cancer has been significantly associated with poor prognosis of patients (Nagasaki et al. Citation2009). When HSD17B12 was knocked down in cancer cells with high expression of HSD17B12, cell growth was significantly inhibited with reduced total amounts of FAs and arachidonic acid. These changes were completely reversed by the addition of arachidonic acid. HSD17B12 activity may be correlated with PG production (Nagasaki et al. Citation2009). The biological significance and function of 17BHSD12 in human cancer remain unknown, and further studies are needed to elucidate its mechanism.
Epigenetic regulation of ELOVL5 in CRC
Epigenetic regulation is one of the mechanisms that regulate downstream gene expression. Approximately 70% of genes contain CpG islands in their promoters (Saxonov et al. Citation2006; Deaton and Bird Citation2011). While unmethylated regions usually serve as transcriptional initiation sites, their methylation can form heterochromatin that inhibits the interaction with transcription factors or chromatin remodelers, leading to the inhibition of downstream gene expression (Saxonov et al. Citation2006; Deaton and Bird Citation2011). Many tumors exhibit changes in the methylation status of CpG islands during tumorigenesis (Paweł and Maria Małgorzata Citation2022). In a subset of colorectal tumors, an exceptionally high frequency of methylation of some CpG islands has been described and categorized as a ‘CpG island methylator phenotype (CIMP)’, where BRAF mutations are present in most cases (Ogino et al. Citation2011; Boot et al. Citation2017). CIMP is one of the mechanisms that lead to chromatin instability and microsatellite instability during tumorigenesis of CRC (Ogino et al. Citation2011).
Methylation-associated transcriptional repression has emerged as a mechanism that inhibits the expression of ELOVL5 in CRC cell lines (Boot et al. Citation2017). When DNA methylation and gene expression profiles were generated in CRC cell lines and cell lines with BRAF mutations, one of the subsets was reportedly associated with CIMP. ELOVL5 was hypermethylated along with family with sequence similarity 127 member B (FAM127B), mitochondrial transcription termination factor 1 (MTERF1), and zinc finger protein 606 (ZNF606) genes whose expressions were low (Boot et al. Citation2017). These findings suggest that ELOVL5 expression can be epigenetically regulated in CRC cells. A survival analysis using TCGA data showed that ELOVL5 hypermethylation was associated with improved overall survival, suggesting that low expression of ELOVL5 by DNA hypermethylation is protective in the progression of CRC progression (Boot et al. Citation2017). The authors also described the correlation of ELOVL5 expression with tumor stage and relapse-free survival. However, a mechanism that can explain this correlation has yet to be elucidated; changes in lipogenesis, the downstream transcriptional effect of the MAPK pathway, and its effects on apoptosis have been proposed.
Conclusion
The roles of PGE2 and the responsible enzyme (COX-2) in the stages of CRC have been actively studied. The enzymes in the VL-PUFA synthesis pathway, including ELOVL5, FADS2, and HSD17B12, can affect cellular arachidonic acid level and PGE2 level, and thus could change the biological activities of cancer cells in multiple stages. Arachidonic acid and other VL-PUFAs can affect tumorigenesis in PGE2-independent manners as well, including stabilization of β-catenine, ferroptosis, ROS generation, regulation of transcription factors, and de novo lipogenesis. Recent studies have revealed an association between the activities of enzymes synthesizing VL-PUFA and tumorigenesis and cancer progression, although the mechanisms are still unknown. More studies are needed to elucidate the role of these enzymes and further their use as diagnostic and prognostic markers, and as therapeutic targets for CRC.
Disclosure statement
No potential conflict of interest was reported by the author(s).
Additional information
Funding
References
- Bae S, Kim MK, Kim HS, Moon YA. 2020. Arachidonic acid induces ER stress and apoptosis in HT-29 human colon cancer cells. Anim Cells Syst. 24(5):260–266.
- Boot A, Oosting J, van Eendenburg JDH, Kuppen PJK, Morreau H, van Wezel T. 2017. Methylation associated transcriptional repression of ELOVL5 in novel colorectal cancer cell lines. PLoS One. 12(9):e0184900.
- Bordoni A, Nunzio D, Danesi M, Biagi F, L P. 2006. Polyunsaturated fatty acids: from diet to binding to PPARs and other nuclear receptors. Genes Nutr. 1(2):95–106.
- Burns JL, Nakamura MT, Ma DWL. 2018. Differentiating the biological effects of linoleic acid from arachidonic acid in health and disease. Prostaglandins Leukot Essent Fatty Acids. 135:1–4.
- Cai F, Dupertuis YM, Pichard C. 2012. Role of polyunsaturated fatty acids and lipid peroxidation on colorectal cancer risk and treatments. Curr Opin Clin Nutr Metab Care. 15(2):99–106.
- Cai Q, Gao YT, Chow WH, Shu XO, Yang G, Ji BT, Wen W, Rothman N, Li HL, Morrow JD, et al. 2006. Prospective study of urinary prostaglandin E2 metabolite and colorectal cancer risk. J Clin Oncol. 24(31):5010–5016.
- Calder PC. 2017. Omega-3 fatty acids and inflammatory processes: from molecules to man. Biochem Soc Trans. 45(5):1105–1115.
- Centenera MM, Scott JS, Machiels J, Nassar ZD, Miller DC, Zinonos I, Dehairs J, Burvenich IJG, Zadra G, Chetta PM, et al. 2021. ELOVL5 is a critical and targetable fatty acid elongase in prostate cancer. Cancer Res. 81(7):1704–1718.
- Chambrier C, Bastard J-P, Rieusset J, Chevillotte E, Bonnefont-Rousselot D, Therond P, Hainque B, Riou J-P, Laville M, Vidal H. 2002. Eicosapentaenoic acid induces mRNA expression of peroxisome proliferator-activated receptor γ. Obes Res. 10(6):518–525.
- Chan AT, Ogino S, Fuchs CS. 2007. Aspirin and the risk of colorectal cancer in relation to the expression of COX-2. N Engl J Med. 356(21):2131–2142.
- Cinti DL, Cook L, Nagi MN, Suneja SK. 1992. The fatty acid chain elongation system of mammalian endoplasmic reticulum. Prog Lipid Res. 31:1–51.
- Dannenberg AJ, Altorki NK, Boyle JO, Dang C, Howe LR, Weksler BB, Subbaramaiah K. 2001. Cyclooxygenase 2: a pharmacological target for the prevention of cancer. Lancet Oncol. 2(9):544–551.
- Deaton AM, Bird A. 2011. Cpg islands and the regulation of transcription. Genes Dev. 25(10):1010–1022.
- Dentin R, Denechaud P-D, Benhamed F, Girard J, Postic C. 2006. Hepatic gene regulation by glucose and polyunsaturated fatty acids: a role for ChREBP. J Nutr. 136(5):1145–1149.
- Dierge E, Debock E, Guilbaud C, Corbet C, Mignolet E, Mignard L, Bastien E, Dessy C, Larondelle Y, Feron O. 2021. Peroxidation of n-3 and n-6 polyunsaturated fatty acids in the acidic tumor environment leads to ferroptosis-mediated anticancer effects. Cell Metab. 33(8):1701–1715.e1705.
- Dixon SJ, Lemberg KM, Lamprecht MR, Skouta R, Zaitsev EM, Gleason CE, Patel DN, Bauer AJ, Cantley AM, Yang WS, et al. 2012. Ferroptosis: An iron-dependent form of nonapoptotic cell death. Cell. 149(5):1060–1072.
- Dupertuis YM, Meguid MM, Pichard C. 2007. Colon cancer therapy: new perspectives of nutritional manipulations using polyunsaturated fatty acids. Curr Opin Clin Nutr Metab Care. 10(4):427–432.
- Habbel P, Weylandt KH, Lichopoj K, Nowak J, Purschke M, Wang JD, He CW, Baumgart DC, Kang JX. 2009. Docosahexaenoic acid suppresses arachidonic acid-induced proliferation of LS-174 T human colon carcinoma cells. World J Gastroenterol. 15(9):1079–1084.
- Habermann N, Ulrich CM, Lundgreen A, Makar KW, Poole EM, Caan B, Kulmacz R, Whitton J, Galbraith R, Potter JD, et al. 2013. PTGS1, PTGS2, ALOX5, ALOX12, ALOX15, and FLAP SNPs: interaction with fatty acids in colon cancer and rectal cancer. Genes Nutr. 8(1):115–126.
- Hall MN, Chavarro JE, Lee I-M, Willett WC, Ma J. 2008. A 22-year prospective study of fish, n-3 fatty acid intake, and colorectal cancer risk in men. Cancer Epidemiol Biomarkers Prev. 17(5):1136–1143.
- Hannah VC, Ou J, Luong A, Goldstein JL, Brown MS. 2001. Unsaturated fatty acids down-regulate SREBP isoforms 1a and 1c by two mechanisms in HEK-293 cells. J Biol Chem. 276(6):4365–4372.
- Hardman WE. 2004. (n-3) fatty acids and cancer therapy. J Nutr. 134(12 Suppl):3427s–3430s.
- Hashemi Goradel N, Najafi M, Salehi E, Farhood B, Mortezaee K. 2019. Cyclooxygenase-2 in cancer: A review. J Cell Physiol. 234(5):5683–5699.
- Hiraide T, Ikegami K, Sakaguchi T, Morita Y, Hayasaka T, Masaki N, Waki M, Sugiyama E, Shinriki S, Takeda M, et al. 2016. Accumulation of arachidonic acid-containing phosphatidylinositol at the outer edge of colorectal cancer. Sci Rep. 6:29935.
- Hodge AM, Williamson EJ, Bassett JK, MacInnis RJ, Giles GG, English DR. 2015. Dietary and biomarker estimates of fatty acids and risk of colorectal cancer. Int J Cancer. 137(5):1224–1234.
- Hofmanová J, Slavík J, Ciganek M, Ovesná P, Tylichová Z, Karasová M, Zapletal O, Straková N, Procházková J, Bouchal J, et al. 2021. Complex alterations of fatty acid metabolism and phospholipidome uncovered in isolated colon cancer epithelial cells. Int J Mol Sci. 22(13):6650.
- Innes JK, Calder PC. 2018. Omega-6 fatty acids and inflammation. Prostaglandins Leukot Essent Fatty Acids. 132:41–48.
- Jakobsson A, Westerberg R, Jacobsson A. 2006. Fatty acid elongases in mammals: their regulation and roles in metabolism. Prog Lipid Res. 45(3):237–249.
- Jara-Gutiérrez Á, Baladrón V. 2021. The role of prostaglandins in different types of cancer. Cells. 10(6):1487.
- Jump DB. 2009. Mammalian fatty acid elongases. Methods Mol Biol. 579:375–389.
- Kemiläinen H, Huhtinen K, Auranen A, Carpén O, Strauss L, Poutanen M. 2018. The expression of HSD17B12 is associated with COX-2 expression and is increased in high-grade epithelial ovarian cancer. Oncology. 94(4):233–242.
- Kim H, Rodriguez-Navas C, Kollipara RK, Kapur P, Pedrosa I, Brugarolas J, Kittler R, Ye J. 2015. Unsaturated fatty acids stimulate tumor growth through stabilization of β-catenin. Cell Rep. 13(3):495–503.
- Lee J-Y, Nam M, Son HY, Hyun K, Jang SY, Kim JW, Kim MW, Jung Y, Jang E, Yoon S-J, et al. 2020. Polyunsaturated fatty acid biosynthesis pathway determines ferroptosis sensitivity in gastric cancer. Proc Natl Acad Sci U S A. 117(51):32433–32442.
- Li J, Ma X, Chakravarti D, Shalapour S, DePinho RA. 2021. Genetic and biological hallmarks of colorectal cancer. Genes Dev. 35(11-12):787–820.
- Lin Y, Meng Y, Zhang J, Ma L, Jiang L, Zhang Y, Yuan M, Ren A, Zhu W, Li S, et al. 2020. Functional genetic variant of HSD17B12 in the fatty acid biosynthesis pathway predicts the outcome of colorectal cancer. J Cell Mol Med. 24(24):14160–14170.
- May-Wilson S, Sud A, Law PJ, Palin K, Tuupanen S, Gylfe A, Hänninen UA, Cajuso T, Tanskanen T, Kondelin J, et al. 2017. Pro-inflammatory fatty acid profile and colorectal cancer risk: A Mendelian randomisation analysis. Eur J Cancer. 84:228–238.
- Mokhtari K, Mahdevar M, Hajipour M, Esmaeili M, Peymani M, Mirzaei S, Nasr-Esfahani MH, Hashemi M, Hushmandi K, Ghaedi K. 2022. Title: involvement of unsaturated fatty acid biosynthesis in CRC progression based on in vitro and in silico studies. Biomed Pharmacother. 153:113338.
- Moon YA, Hammer RE, Horton JD. 2009. Deletion of ELOVL5 leads to fatty liver through activation of SREBP-1c in mice. J Lipid Res. 50(3):412–423.
- Moon YA, Horton JD. 2003. Identification of two mammalian reductases involved in the two-carbon fatty acyl elongation cascade. J Biol Chem. 278(9):7335–7343.
- Mutoh M, Takahashi M, Wakabayashi K. 2006. Roles of prostanoids in colon carcinogenesis and their potential targeting for cancer chemoprevention. Curr Pharm Des. 12(19):2375–2382.
- Nagasaki S, Suzuki T, Miki Y, Akahira J-i, Kitada K, Ishida T, Handa H, Ohuchi N, Sasano H. 2009. 17β-Hydroxysteroid dehydrogenase type 12 in human breast carcinoma: A prognostic factor via potential regulation of fatty acid synthesis. Cancer Res. 69(4):1392–1399.
- Nikulin S, Zakharova G, Poloznikov A, Raigorodskaya M, Wicklein D, Schumacher U, Nersisyan S, Bergquist J, Bakalkin G, Astakhova L, et al. 2021. Effect of the expression of ELOVL5 and IGFBP6 genes on the metastatic potential of breast cancer cells. Front Genet. 12:662843.
- Nitta S, Kandori S, Tanaka K, Sakka S, Siga M, Nagumo Y, Negoro H, Kojima T, Mathis BJ, Shimazui T, et al. 2022. ELOVL5-mediated fatty acid elongation promotes cellular proliferation and invasion in renal cell carcinoma. Cancer Sci. 113(8):2738–2752.
- Ogino S, Chan AT, Fuchs CS, Giovannucci E. 2011. Molecular pathological epidemiology of colorectal neoplasia: an emerging transdisciplinary and interdisciplinary field. Gut. 60(3):397–411.
- Patel MI, Kurek C, Dong Q. 2008. The arachidonic acid pathway and its role in prostate cancer development and progression. J Urol. 179(5):1668–1675.
- Paweł K, Maria Małgorzata S. 2022. Cpg island methylator phenotype- A hope for the future or a road to nowhere? Int J Mol Sci. 23(2):830.
- Peck B, Schulze A. 2016. Lipid desaturation – the next step in targeting lipogenesis in cancer? The FEBS J. 283(15):2767–2778.
- Pender-Cudlip MC, Krag KJ, Martini D, Yu J, Guidi A, Skinner SS, Zhang Y, Qu X, He C, Xu Y, et al. 2013. Delta-6-desaturase activity and arachidonic acid synthesis are increased in human breast cancer tissue. Cancer Sci. 104(6):760–764.
- Phipps AI, Passarelli MN, Chan AT, Harrison TA, Jeon J, Hutter CM, Berndt SI, Brenner H, Caan BJ, Campbell PT, et al. 2015. Common genetic variation and survival after colorectal cancer diagnosis: a genome-wide analysis. Carcinogenesis. 37(1):87–95.
- Pot GK, Geelen A, van Heijningen EM, Siezen CL, van Kranen HJ, Kampman E. 2008. Opposing associations of serum n-3 and n-6 polyunsaturated fatty acids with colorectal adenoma risk: an endoscopy-based case-control study. Int J Cancer. 123(8):1974–1977.
- Romanuik TL, Ueda T, Le N, Haile S, Yong TMK, Thomson T, Vessella RL, Sadar MD. 2009. Novel biomarkers for prostate cancer including noncoding transcripts. Am J Pathol. 175(6):2264–2276.
- Rossen NS, Hansen AJ, Selhuber-Unkel C, Oddershede LB. 2011. Arachidonic acid randomizes endothelial cell motion and regulates adhesion and migration. PLoS One. 6(9):e25196.
- Rothwell PM, Wilson M, Elwin CE, Norrving B, Algra A, Warlow CP, Meade TW. 2010. Long-term effect of aspirin on colorectal cancer incidence and mortality: 20-year follow-up of five randomised trials. Lancet. 376(9754):1741–1750.
- Sakai M, Kakutani S, Horikawa C, Tokuda H, Kawashima H, Shibata H, Okubo H, Sasaki S. 2012. Arachidonic acid and cancer risk: a systematic review of observational studies. BMC Cancer. 12:606.
- Sano H, Kawahito Y, Wilder RL, Hashiramoto A, Mukai S, Asai K, Kimura S, Kato H, Kondo M, Hla T. 1995. Expression of cyclooxygenase-1 and -2 in human colorectal cancer. Cancer Res. 55(17):3785–3789.
- Sasazuki S, Inoue M, Iwasaki M, Sawada N, Shimazu T, Yamaji T, Takachi R, Tsugane S, ftJPHCBPS G. 2011. Intake of n-3 and n-6 polyunsaturated fatty acids and development of colorectal cancer by subsite: Japan public health center–based prospective study. Int J Cancer. 129(7):1718–1729.
- Saxonov S, Berg P, Brutlag DL. 2006. A genome-wide analysis of CpG dinucleotides in the human genome distinguishes two distinct classes of promoters. Proc Natl Acad Sci U S A. 103(5):1412–1417.
- Shkurnikov MY, Poloznikov AA, Nikulin SV, Schumacher U, Wicklein D, Stürken C, Galatenko VV, BY A. 2019. Transcriptome guided drug combination suppresses proliferation of breast cancer cells. Bull Exp Biol Med. 166(5):656–660.
- Spector AA, Kim HY. 2015. Discovery of essential fatty acids. J Lipid Res. 56(1):11–21.
- Szajnik M, Szczepanski MJ, Elishaev E, Visus C, Lenzner D, Zabel M, Glura M, DeLeo AB, Whiteside TL. 2012. 17β hydroxysteroid dehydrogenase type 12 (HSD17B12) is a marker of poor prognosis in ovarian carcinoma. Gynecol Oncol. 127(3):587–594.
- Tang C, Cho HP, Nakamura MT, Clarke SD. 2003. Regulation of human Δ-6 desaturase gene transcription: identification of a functional direct repeat-1 element. J Lipid Res. 44(4):686–695.
- Thanikachalam K, Khan G. 2019. Colorectal cancer and nutrition. Nutrients. 11(1):164.
- Tsachaki M, Strauss P, Dunkel A, Navrátilová H, Mladenovic N, Odermatt A. 2020. Impact of 17β-HSD12, the 3-ketoacyl-CoA reductase of long-chain fatty acid synthesis, on breast cancer cell proliferation and migration. Cell Mol Life Sci. 77(6):1153–1175.
- Van Blarigan EL, Fuchs CS, Niedzwiecki D, Ye X, Zhang S, Song M, Saltz LB, Mayer RJ, Mowat RB, Whittom R, et al. 2018. Marine ω-3 polyunsaturated fatty acid and fish intake after colon cancer diagnosis and survival: CALGB 89803 (alliance). Cancer Epidemiol Biomarkers Prev. 27(4):438–445.
- Wang D, Fu L, Sun H, Guo L, DuBois RN. 2015. Prostaglandin E2 promotes colorectal cancer stem cell expansion and metastasis in mice. Gastroenterology. 149(7):1884–1895.
- Wang D, Mann JR, DuBois RN. 2005. The role of prostaglandins and other eicosanoids in the gastrointestinal tract. Gastroenterology. 128(5):1445–1461.
- Wang Q, Morris RJ, Bode AM, Zhang T. 2022. Prostaglandin pathways: opportunities for cancer prevention and therapy. Cancer Res. 82(6):949–965.
- WHO. 2022. Cancer. World Health Organizaion; [accessed 2022 2022 Nov 02]. https://www.who.int/news-room/fact-sheets/detail/cancer.
- Yamashita Y, Nishiumi S, Kono S, Takao S, Azuma T, Yoshida M. 2017. Differences in elongation of very long chain fatty acids and fatty acid metabolism between triple-negative and hormone receptor-positive breast cancer. BMC Cancer. 17(1):589.
- Yoshikawa T, Shimano H, Yahagi N, Ide T, Amemiya-Kudo M, Matsuzaka T, Nakakuki M, Tomita S, Okazaki H, Tamura Y, et al. 2002. Polyunsaturated fatty acids suppress sterol regulatory element-binding protein 1c promoter activity by inhibition of liver X receptor (LXR) binding to LXR response elements. J Biol Chem. 277(3):1705–1711.