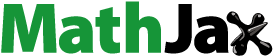
Abstract
Composite materials used in biomedical applications based on bacterial cellulose (BC) have attracted considerable scientific interest due to their specific and excellent properties. This work reports the fabrication of silver nanoparticles (AgNPs) deposited on nanofibrillated BC (BC-AgNPs nanocomposite) for antimicrobial applications. BC, AgNPs, as well as BC-AgNPs nanocomposite formation were fully characterized using various techniques such as X-ray diffraction (XRD), field emission scanning electron microscopy (FE-SEM) analyses, Fourier transform infrared (FTIR) spectoscopy, transmission electron microscopy (TEM) and small-angle X-ray scattering (SAXS). Furthermore, the antimicrobial effects of produced BC-AgNP composites were evaluated on two pathogenic microorganisms, a gram-negative (Escherichia coli) and a gram-positive (Staphylococcus aureus) by determining their minimum inhibitory concentrations (MIC), and minimum bactericidal concentrations (MBC) using an agar diffusion assay. The results show that the BC-AgNPs composite has good antimicrobial activity against Escherichia coli but less against Staphylococcus aureus.
Graphical Abstract
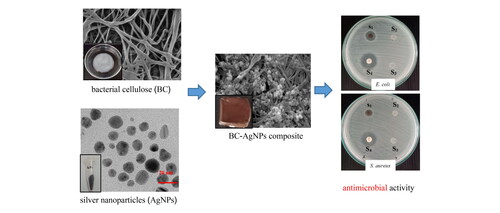
Introduction
Nanocomposites containing various functional nanomaterials have attracted considerable scientific interest due to the requirement for materials with improved physical and chemical properties. Recently, there has been growing interest in the production of cellulose-based composite scaffolds compounded with other ingredients, such as metal nanoparticles (NPs) for antimicrobial applications [Citation1–4]. Cellulose can be produced by bacteria, and in this case, it is known as bacterial cellulose (BC). BC naturally exists as very pure nanosized fibrils and does not require additional purification prior to its use. Based on these benefits, along with its high crystallinity and stiffness, BC has recently gained much interest in developing techniques to produce BC for various industrial applications [Citation5, Citation6]. One of these is the combination of BC with silver nanoparticles (AgNPs) [Citation7, Citation8]. AgNPs are of interest due to their optical properties arising from localized surface plasmon resonance (LSPR) [Citation9–11]. Several successful methods have been reported to prepare AgNPs, including photochemical, microwave-assisted, chemical reduction and plant-ne synthesis [Citation12]. Previously published work presented the combination of AgNPs with BC by impregnating AgNPs into BC through the chemical reduction by immersing BC in the silver nitrate solution [Citation8]. Sodium borohydride was used to reduce the absorbed silver ion inside BC to metallic AgNPs. However, this work demonstrated green synthesis of AgNPs using aqueous leaf extract of Moringa oleifera Lam. as reducing agent. Leaf extract can be used to synthesize AgNPs at such a low concentration. Also, there is no dangerous chemical or harmful physical technique in the process. This method applied here is easy to perform, cost-effective, and sustainable. These particles are not toxic, while the high efficiency in eliminating bacterial infections is observed [Citation13]. The obtained AgNPs is next composited with BC which produced in out Biomolecular laboratory. BC was produced by G. xylinus BKNC 19 bacteria that isolated and identified with high BC production from fermented plant beverages [Citation14] using the banana extract as the alternative agricultural waste as nutrient sources. Cellulose is a requirement in various industries, but it also has a high production cost. Therefore, the development of cost-effective media derived from agricultural waste and solid waste has been compromising to improve the cost competitiveness of BC production. Subsequently, the AgNPs were combined with BC (BC-AgNPs composite) by immersing BC in the aqueous AgNPs through a green, cost-effective and simple method. The prepared BC-AgNPs were characterized using scanning electron microscopy (FE-SEM), energy dispersive X-ray spectroscopy (EDX), X-ray diffraction (XRD), Fourier transform infrared spectroscopy (FTIR) analysis and transmission electron microscopy (TEM). Antimicrobial activity of the synthesized BC-AgNPs composite was evaluated against S. aureus and E. coli bacteria using disk diffusion method on an agar plate. The minimum inhibitory concentration (MIC), minimum bactericidal concentration (MBC) and time kill curve studies were also determined.
Materials and methods
Chemicals and materials
The silver nitrate (AgNO3) used in the current study was of analytical reagent grade and obtained from Sigma-Aldrich chemicals. It was used as received with no further purification. Fresh leaves of Moringa oleifera Lam. were collected from farms in Nong Khai Province, Northeastern Thailand. They were immediately processed in a lab for further studies. S. aureus and E. coli were obtained from the Biomolecular Laboratory, Faculty of Interdisciplinary Studies, Khon Kaen University, Nong Khai Campus, Thailand. Deionized water was utilized throughout the experiments.
Synthesis of AgNPs
To synthesize of AgNPs, 5 g of dried Moringa oleifera Lam. leaf fine-cut leaves were extracted with 100 ml of distilled water to prepare a leaf extract solution (MLE) for the reduction of Ag ions (Ag+) to Ag NPs (Ag0). Subsequently, 1.50 mL of MLE was taken to react with a fixed concentration of 50 mL of AgNO3 volume and the reaction time of 50 min at 60 °C in a water bath. Reduction of AgNPs was observed by change in their color of the reaction solution during temperature treatment. Their UV–visible spectra were recorded for investigating of the development of AgNPs. After complete reaction, the colloidal solution was collected by centrifugation at 10,000 rpm for 15 min. and washed twice with distilled water to remove the unreacted materials and impurities. Finally, the collected powder was dried, characterized and stored for further use.
Bacterial cellulose production
Bacterial cellulose was produced by G. xylinus BKNC 19, cultured in agricultural waste medium (83.24 ml/L banana extract, 5 g/L yeast extract, and 5 g/L peptone) The sugar content of banana extract was analyzed using high performance liquid chromatography (HPLC). The culture media were prepared with total sugar contents matching the level of glucose in standard medium (Hestrin and Schramm’s medium) and incubated at 30 °C for 7 days under static conditions. After incubated, BC pellicle were formed on culture medium surfaces and were collected to washed followed by boil with a 0.5 N NaOH solution for 10 min and rinsed until the pH is neutral. The pellicle was stored in distilled water for further use.
Production of BC-AgNP nanocomposites
BC materials and powdered AgNPs were prepared separately. Then, the purified BC pellicles were cut into small pieces, placed in flasks with 0.05, 0.1 and 0.2%w/v of AgNPs powder, and stirred using a magnetic stirrer for 24 h. The antimicrobial activities of BC-AgNPs composite were evaluated and then they were freeze-dried to retain their 3 D network structure before determination of their characteristics and properties.
Characterization
The optical absorption properties of the synthesized AgNPs were first investigated using ultraviolet-visible (UV-Vis) spectrophotometry (Shimadzu UV-1800 spectrophotometer) over the wavelength range of 300–750 nm with a resolution of 1 nm. TEM was used to identify the morphology, size and shape of these nanoparticles. Their crystalline nature was determined using selected area electron diffraction (SAED), examined in the high resolution mode of TEM. The structure and crystallinity of AgNPs, BC and BC-AgNPs composite were analyzed using an XRD apparatus equipped with a Cu () (
Å) radiation source. XRD spectra were recorded over 2θ angles ranging from 10 to
and a step size of 0.2°. FTIR spectra of the MLE, AgNPs, BC and BC-AgNPs composite were analyzed to determine the organic functional groups present in the samples. FTIR studies were carried out using a Shimadzu FTIR spectrometer in an attenuated total reflection mode over the spectral range of 4000–500 cm−1 and with a resolution of 4 cm−1. Small/Wide angle X-ray scattering (SAXS/WAXS) measurements were performed at the Beamline 1.3 W: SAXS/WAXS at the Synchrotron Light Research Institute (Public Organization), Nakhon Ratchasima, Thailand. The X-ray energy of 9.0 keV were extracted from 1.2 GeV synchrotron light source [Citation15]. For SAXS experiments, the 1510.40 and 4560.35 mm sample to detector distances were set and calibrated. The two-dimensional SAXS patterns were recorded using and SX-165 Mar CCD detector. Background subtraction was performed on all data and SAXS profiles from 2 SDDs were merged using in-house software, SAXSIT. In order to analyze the particle size and size distribution, the unified exponential-power law model of Beaucage [Citation16] was used to fit the experimental data. The fitting was carried out with a least-squares minimization procedure using the software package SASfit [Citation17].
Antimicrobial properties of BC-AgNP nanocomposite materials
Antimicrobial activities were investigated against two different pathogenic microorganisms. The model bacteria were a gram-negative, E. coli, and a gram-positive, S. aureus. Testing was done by means of disc diffusion in agar plates containing nutrient agar (NA). Fresh bacterial cultures were transferred onto NA plates and gently spread over their surfaces. Then, sample pellicles of BC-AgNPs nanocomposites were cut into discs and sterilized under UV radiation for 2 h. They were placed on the surface of NA sheets onto which the pathogenic bacteria were spread. The NA plates were incubated at 37 ̊C to permit better diffusion of BC-AgNPs nanocomposites to the surfaces of bacterial cells. After 18 h of incubation, inhibition zones around the discs were observed and their widths measured using Vernier calipers.
The MIC and MBC of BC-AgNPs nanocomposite materials
The MIC and MBC of BC-AgNPs nanocomposite materials were determined using a microdilution method. BC-AgNP nanocomposites were diluted in a nutrient broth from 2000 to 3.906 µg/mL and the dilutions were transferred into each well of 96-well plates. Then, 10 µL of bacterial suspension was added to each well and the plates incubated at 37 ̊C for 18 h. The lowest concentration inhibiting visual growth of microorganisms, indicated by increased turbidity, is considered the MIC value. To determine the MBC of a sample, the contents of all wells with no visible bacterial growth were spread on NA plates and incubated for 18 h at 37 ̊C. After incubation, the agar plate with no bacterial growth was designated as the MBC.
The prolonged antimicrobial performance of BC-AgNPs nanocomposite
The prolonged antimicrobial performance of BC-AgNPs nanocomposite was examined by the determination of the growth curve of E. coli and S. aureus in the NA culture medium. The concentration of BC-AgNPs nanocomposite was prepared equal to the MIC and MBC values in 15 of the culture medium, cultured with 0.1 mL of bacterial suspension and incubated at 37 °C. One milliliter of cell suspension was transfused at intervals of every 2 h, and analyzed by spectrophotometric with a light source of 600 nm to determine the optical density (OD600) that indicates the amount of microbial growth in relation to turbidity. The growth of E. coli and S. aureus in NB culture medium were compared with the control group without BC-AgNPs nanocomposite.
Results and discussion
Synthesis and properties of AgNPs
MLE after reaction with AgNO3 shows a yellowish-brown color, which is regarded as a preliminary indication of AgNPs (see inset in ). The formation of colloidal AgNPs was identified from the UV-Visible spectrum, shown in . An absorbance peak was observed at around 440 nm, which is due to collective oscillations of the conduction electrons of the AgNPs, also known as the LSPR. TEM images of the synthesized AgNPs (see ) show that the shape of the synthesized AgNPs was nearly spherical, which is in agreement with data in the UV-Visible spectrum. The diameters of the AgNPs were found to be 5-35 as presented in a histogram of particle size distribution (see ). The bright circular spots in the SAED pattern (see ) show circular rings that can reveal the crystalline nature of the AgNPs formed. The effects of various phytochemicals of the MLE as well as their role in the synthesis and stability of AgNPs were investigated using a FTIR technique, the results of which are shown in (curve a). The FTIR spectrum of the MLE shows various vibration bands, such as a strong band of
(ascribed to the stretching vibrations of hydroxyl (–OH) groups), at
and
(related to aliphatic C–H stretching vibrations),
and
(corresponding to asymmetric and symmetric stretching vibrations of C = O in carboxylic groups),
(symmetric bending of CH3),
(C–H bending),
(–SO3 stretching) and a prominent peak at
(C-O stretching from alcohol, carboxylic acid, ester and ethers and C-N stretching vibrations of amines) [Citation18, Citation19]. After the MLE reacted with AgNO3, these characteristic peaks were shifted into new positions, disappeared or remained unchanged (see (curve c)). For example, the shift from
to
to
or the disappearance the peaks of
and
after reaction with AgNO3 suggests that the biomolecules of M. oleifera Lam may be responsible for reduction and stabilization of AgNPs [Citation18, Citation19]. Four characteristic diffraction peaks of the synthesized AgNPs by XRD ( (curve b)) were observed at
values of
and
which are indexed to (111), (200), (220) and (311) planes of a face-centered cubic structure of Ag, respectively [Citation18, Citation19]. Some additional peaks were observed at
and
corresponding to the organic groups present in M. oleifera Lam. responsible for the reduction of Ag ions and formation of the AgNPs [Citation20]. For making a database, the synthesis and properties of the obtained AgNPs were compared with the AgNPs synthesized with the other natural product extracts as shown in .
Figure 1. UV–visible spectra of reaction mixtures containing 1.5 mM AgNO3 and MLE mixed at a volume ratio 50 mL:1.5 mL at optimum reaction times of 50 min. Inset shows imagery of M. Oleifera Lam. leaves, dried fine-cut leaves and mixtures containing 1.5 mM AgNO3 and MLE.
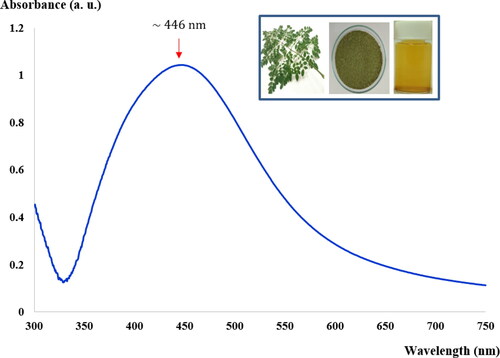
Figure 2. TEM micrographs of the synthesized AgNPs (a)– (b) at various magnifications, (c) is the SAED pattern and (d) size distribution histogram of AgNPs.
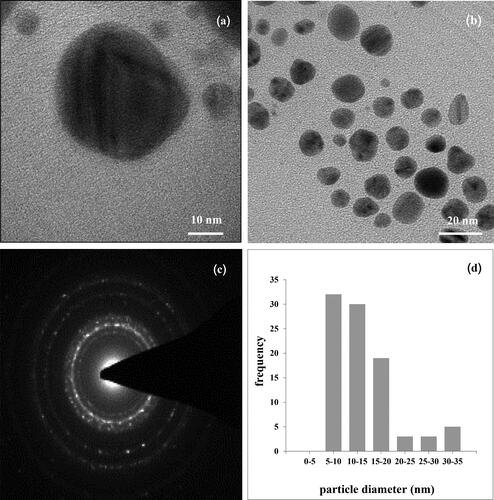
Figure 3. FTIR spectra of the (a) MLE, (b) pure BC, (c) synthesized AgNPs and (d) BC-AgNP nanocomposites.
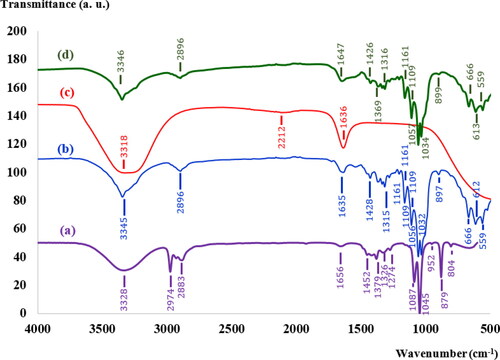
Figure 4. XRD pattern of (a) freeze-dried BC (b) synthesized AgNPs powders and (c) the BC-AgNP nanocomposites with AgNPs at 0.2%w/v. The Bragg reflection planes of the respective crystallites are shown.
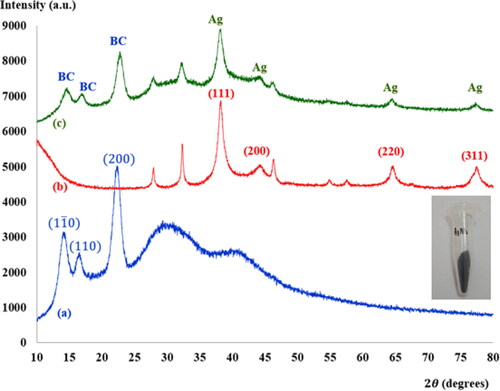
Table 1. Comparison of silver nanoparticles using different plant extracts.
Production and properties of BC
shows BC produced by G. xylinus BKNC 19 using an agricultural waste product, banana juice with added nitrogen source as culture medium. The colors of BC gels before and after treatment in NaOH were respectively yellowish and white (see ). The yellowish color of BC may be due to the color of culture media. The pellicles of all BC samples were treated in NaOH to remove G. xylinus and associated biomass, resulting in purified white pellicles [Citation26] (see ). shows the surface morphology of a freeze-dried BC sample analyzed using FE-SEM at magnifications of (),
() and
(). The FE-SEM images indicate that the three-dimensional network of ribbon-like microfibrils formed a highly fibrous structure. Most of the microfibrils were approximately 40-70 nm wide and interwoven in a nano-sized structure. Microfibril lengths could not be determined since their ends were not visible, even at low magnification (
). XRD spectra of freeze-dried BC, collected from
to
) are shown in . The
(110) and (200) crystal planes have been reported for BC [Citation27–29], which can be observed at
and
respectively, in this work. These diffraction peaks are characteristic of cellulose [Citation27]. Moreover, it can be clearly seen in that BC is not a completely crystalline material and its diffraction patterns from the BC films show broad peaks [Citation28, Citation29]. The FTIR spectrum of freeze-dried BC (see ) shows characteristic BC peaks, such as the strong band at
(stretching of O-H bonds,
and asymmetric angular deformation of C-H bonds),
(symmetric angular deformation of C-H bonds),
(asymmetrical stretching of C-O-C glycoside bonds),
and
(stretching of C-OH and C-C-OH bonds in secondary and primary alcohols, respectively) and at
corresponding to angular deformation of C-H bonds [Citation28, Citation29].
Production and properties of BC-AgNPs nanocomposites
BC-AgNP nanocomposite formation from Ag-impregnated pure BC was accomplished using a wet process [Citation30]. An image of a BC-AgNP nanocomposite sheet is shown in . The addition of AgNPs makes the BC color darker. The yellowish brown color of the films was due to incorporation of AgNPs into the BC matrix. shows a set of selected FE-SEM images of the surfaces of BC and BC-AgNPs nanocomposite sheets. The results indicate that in the absence of AgNPs, BC nanofibers formed highly interconnected and reasonably dense films with irregularly shaped pores (). show FE-SEM analysis of BC-AgNP nanocomposite sheets that were used to investigate the distribution of AgNPs on the BC fibrils and the particle size distribution of the AgNPs. Clearly, as the Ag NP content was progressively increased, there were more AgNPs on the composite surfaces. The spaces/voids between BC nano-fibrils were filled with AgNPs. High AgNP contents result in formation of large BC-AgNPs clusters, which remained intact since no nanofibers were seen between the clusters (). Individual spherical particles were observed entrapped between the BC nanofibrils. These aggregates were uniformly distributed in the nanofiber matrix. Above certain AgNPs content, the aggregates became larger than the inter-fiber pores and thus became embedded into the nanofiber matrix causing the nanofibers to be pushed apart. This disrupted the structure the nanofiber matrix in the packed bed structure. Hence, AgNP contents between 0.05%w/v and 0.10%w/v (low loading) represent one regime, where the role of AgNPs is to fill gaps in the nanofiber network. An AgNP content of 0.20%w/v (high loading) represents another regime, where AgNPs clusters form a much tighter, controlled pore structure (). Analysis by EDX of BC-AgNPs nanocomposite confirms the presence of the elemental metal signal of Ag, as shown in . The specific functional groups in the BC-AgNP nanocomposite material were investigated using FTIR in the range of with a resolution of
They correspond to crystalline BC and AgNPs, shown in (curve d). The absorbed peaks, such as the strong bands at
and
and
are related to BC composition. Moreover, the peaks observed at around
and
were shifted due to treatment in an aqueous solution of AgNO3 with MLE during a reduction process, followed by capping and stabilization of the AgNPs [Citation18]. (curve c) shows XRD spectra of BC-AgNPs nanocomposites, indicating the main diffraction peaks correspond to crystalline BC and Ag. The characteristic peaks observed at
and
are related to the (111), (200), (220) and (311) crystal planes of the face centered cubic structure of Ag [Citation18, Citation19]. Diffraction peaks at
and
corresponded to the
and
planes, respectively, where the crystal planes of BC. This pattern was in agreement with those of earlier studies [Citation31]. It was found that there was no change in the position of diffraction peaks after addition of AgNPs to the BC. This indicated that there were no chemical interactions formed between AgNPs and BC. However, it is confirmed that no chemical interactions formed between AgNPs and BC. Consequently, the long-term loss of AgNPs during use of Ag should be considered as an important factor of the Ag-contained antimicrobial materials from the viewpoint of practical applications [Citation7]. Due to the Ag particles located in the surface region of the BC matrix was ready to loss when rinsed in physiological saline solution. The dispersion of AgNPs into the inner part of the BC matrix is important way to be achieved a prolonged antibacterial effect.
Figure 7. FE-SEM micrographs of (a) a BC nanofiber sheet alone and BC nanofiber composite with (b) 0.05%w/v, (c) 0.1%w/v, (d) 0.2%w/v at high magnification (), and (e) 0.1%w/v and (f) 0.2%w/v Ag NPs at lower magnification (
).
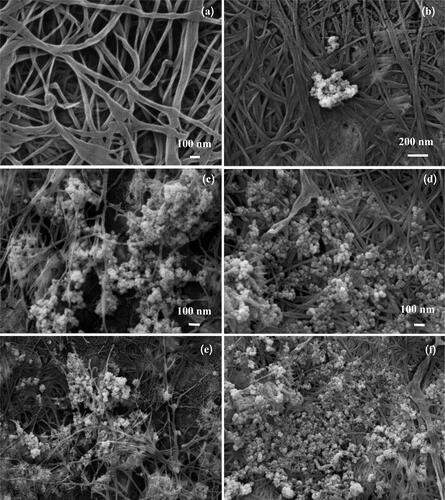
Figure 8. FE-SEM and EDX analyses of BC-AgNP nanocomposites. Figure 8 (a) shows FE-SEM imagery of BC-AgNP nanocomposites. Figure 8 (b) gives the Energy Dispersive X-ray spectrum of BC-AgNP nanocomposites and depicts the elemental components in the BC-AgNP nanocomposites.
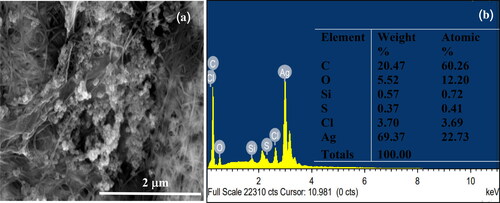
Additionally, the size of AgNPs embedded in BC was investigated using SAXS. The SAXS intensity I(q) as function of the scattering vector q obtained from each sample. To obtain the size of AgNPs in BC-AgNPs, the curves were fitted using the SAXS fit code [Citation19]. The morphological parameters of AgNPs and BC-AgNPs nanocomposite samples determined by SAXS are summarized in . It was found that the synthesized AgNP size was around 19 nm, in agreement with the TEM results in . The SAXS technique tends to give more statistically reliable estimates of nanoparticle sizes than electron microscopy. This is because the particle size distribution obtained by the SAXS technique is typically estimated over a large number of AgNPs, while the size distribution obtained using a TEM technique is based on many fewer measurements [Citation32].
Table 2. Morphological parameters of powdered AgNPs and BC-AgNP nanocomposites from SAXS data.
Assessment of antimicrobial activity
The disc diffusion method
AgNPs are known to be more effective against gram-negative than gram positive bacteria. In the current study, assessment of antimicrobial activities of BC-AgNP nanocomposite composite materials were investigated against pathogenic gram negative E. coli and gram positive S. aureus bacteria using a disc diffusion method, as depicted in and . It can be seen that BC-AgNP nanocomposite materials show clear zones, indicating their good antimicrobial behavior. The maximal zones of inhibition of BC-AgNP nanocomposite materials against S. aureus and E. coli were found to be 9.50 ± 0.50 and 13.17 ± 0.58 mm, respectively. This was visualized using photographs of the S1 sample showing clear zones against the E. coli and S. aureus bacteria in , respectively. Inhibition of E. coli was greater than that of S. aureus. This may be attributed to the characteristics of their bacterial species, i.e. differences in thickness and constituents of their cell walls and membranes structures [Citation32]. Additionally, the BC matrix (S3 sample) and composite material of BC and MLE (S2 sample) showed no inhibition zones at the concentration levels used in this study. This result indicates that BC-AgNP nanocomposite materials have considerable antimicrobial effects.
Figure 9. Antimicrobial activity against (a) E. coli and (b) S. aureus. (S1) BC-AgNP nanocomposites, (S2) BC-MLE, (S3) BC and (S4) a standard antibiotic, streptomycin.
“-” there is no growth, “+” there is growth
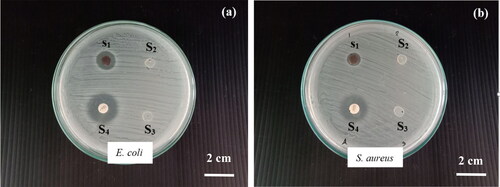
Table 3. Inhibition zone width (IZD) of synthesized BC-AgNP nanocomposites, BC-MLE, BC, and a standard antibiotic.
MIC and MBC
The potential antimicrobial activity of BC-AgNP nanocomposites were also analyzed by means of MIC and MBC tests. The MIC and MBC values of BC-AgNP nanocomposite materials required to inhibit the growth of E. coli and S. aureus bacteria are presented in and . After incubation of those samples and bacteria at 37 °C for 18 h, no turbidity was observed in-vitro at concentrations ranging from 250-2000 µg/ml, indicating inhibition of bacterial growth (see ). The MIC value of both gram negative E. coli and gram positive S. aureus bacteria were found to be 250 µg/ml. This results differ from Moodley et al.(2018) [Citation33] that reported MIC values of AgNPs synthesized from M. oleifera leaf extract had stronger inhibition of gram-negative bacteria than gram-positive bacteria. While the MBC value (see and ), the bactericidal activity against E. coli and S. aureus were evident at 500 and 2000 ug/ml, respectively. The results indicated that BC-AgNP nanocomposites had a more pronounced effect against E. coli comparing to S. aureus pathogens. These results agree with Erjaee et al. (2017) [Citation34] have been reported that gram negative bacteria E. coli had more sensitive to AgNPs than gram positive bacteria S. aureus. This was thought to be due to the difference in bacterial cell walls that affected to bactericidal activity. It is well known that gram negative bacteria contain a thin layer of peptidoglycan and outer membrane composed with lipid and lipoproteins. Therefore, it was possible that nanoparticles could bind to cell surface and diffused within the cytoplasmic membrane [Citation35]. Whereas gram positive bacteria contain a thick peptidoglycan layer along with teichoic acid and lipoteichoic acids make a strong negative charge on their cell surface that may act as a barrier to reach the cytoplasmic membrane of sliver ions [Citation36].
Figure 10. MIC determination of the BC-AgNPs composite against E. coli and S. aureus (a), and (b) table of MBC shows bactericidal effect of the bacteria suspensions taken from the MIC test.
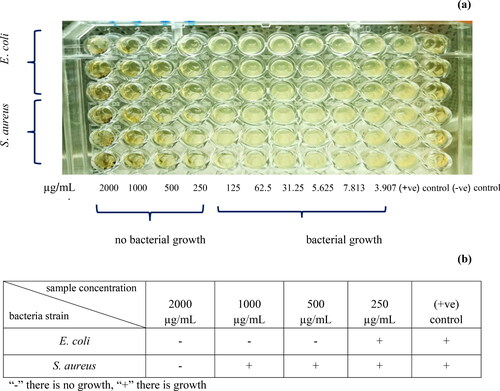
Table 4. MIC and MBC of BC-AgNP nanocomposites against E. coli and S. aureus bacteria.
The prolonged antimicrobial performance of BC-AgNPs nanocomposite
In order to represent the long-term antimicrobial effect of BC-AgNPs composite material on the growth of a gram positive S. aureus and a gram negative E. coli bacteria in the culture medium over period time of 48 h. The growth of bacteria was investigated by measuring optical density at 600 nm (OD600). As shown in , the growth curve of both E. coli () and S. aureus () without BC-AgNPs composite in culture medium were used as control, showed rapid growth in the first 24. Whereas no growth of E. coli and S. aureus were observed only in the first 16 h in culture medium contained the BC-AgNPs composite concentration 250 µg/ml after that both bacteria slightly began to grow. It was due to this concentration equal to MIC of both bacteria that only inhibited the growth of bacteria. This result corresponded to Erjaee et al. (2017) [Citation34] that showed growth curve of E. coli and S. aureus in culture medium contained AgNPs at concentration equal to MIC after 10 h incubation, bacterial growth was observed with increased the OD600 values . But the concentration of BC-AgNPs composite at 500 µg/ml and 2000 µg/ml equal to MBC of E. coli and S. aureus, respectively revealed a complete inhibition of both bacterial growth over period time of 48 h.
Figure 11. Growth curves of E. coli (a) and S. aureus (b) exposed to the BC-AgNPs composite material with different concentrations in culture medium for 48 h.
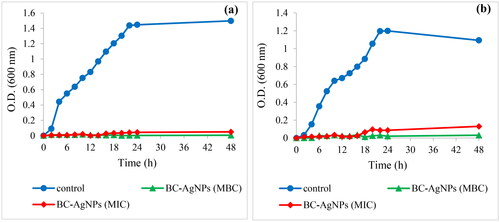
However, it is well known that several factors affect the antimicrobial activity of AgNPs, including particle size, shape, stabilizer and its dose, as well as treatment time [Citation31, Citation37]. The mechanism of inhibitory action caused by AgNPs on the bacterial activity is that when elemental AgNPs are in contact with bacterial cell wall, silver ion is released from the surface of nanoparticles [Citation38, Citation39]. The changing in the local electronic structure of the surface (electronic effects) due to nanostructured size of metal particle can enhance the reactivity of the nanoparticle surfaces [Citation38]. Subsequently, silver ions might either destroy or penetrate the cell membrane causing a critical decrease in enzymatic activity which might change microorganism metabolism and inhibit their growth, lead to the death of the cell [Citation37, Citation38]. Moreover, AgNP is a soft acid which has a natural tendency of an acid to react with a base. Consequently, it will tend to have a higher affinity to react with a soft base [Citation38]. The cell membrane of the bacteria is majorly made up of phosphorus, sulfur-containing proteins in the interior of the cell, as well as with phosphorus-containing compounds such as DNA which are soft bases [Citation38]. The action of AgNPs on the cell can cause the reaction to occur and subsequently lead to cell death. As a final, AgNP releases silver ions which will have an additional contribution to the bactericidal effect of the AgNP. In addition, the difference in antimicrobial activity of AgNPs towards E. coli was more than towards S. aureus. This can be attributed to the difference in sensitivity between them towards AgNPs i.e. thickness and components of cell membrane of bacteria.
Conclusions
This report describes fabrication of BC-AgNPs nanocomposites using a wet process. The production of BC-AgNPs nanocomposite formation were investigated using XRD, FTIR, TEM, FESEM and SAXS analyses. From the FE-SEM morphology, it is evident that the AgNPs, either as individual spherically shaped particles or aggregates, were uniformly distributed within the BC matrix. XRD confirms the presence of AgNPs in the BC-AgNP nanocomposites. The sizes of AgNPs embedded in BC characterized using SAXS was found to be around 19 nm, in agreement with TEM results. The BC-AgNP nanocomposite films showed good antimicrobial activity against S. aureus and E. coli bacteria. However, BC-AgNP nanocomposites were more effective at inhibiting the growth of E. coli than S. aureus bacteria. These results suggest that BC-AgNP nanocomposites can be of interest considered for use in packaging and biomedical applications.
Acknowledgments
The authors acknowledge use of the facilities and support of the Faculty of Interdisciplinary Studies, Khon Kaen University, Nong Khai Campus and SAXS beamline at the Siam Photon Laboratory of the Synchrotron Light Research Institute for SAXS measurements.
Disclosure statement
No potential conflict of interest was reported by the author(s).
Additional information
Funding
Notes on contributors
Sasiporn Audtarat
Sasiporn Audtarat is graduated student in the at the Faculty of Interdisciplinary Studies, Khon Kaen University, Nong Khai Campus. She has worked on characterization and antibacterial activity of bacterial cellulose nanofiber composites with silver nanoparticle.
Piyorot Hongsachart
Piyorot Hongsachart is an assistant professor in the department of applied science, Faculty of Interdisciplinary Studies at Khon Kaen University Nong Khai Campus. She has published mainly on lactic acid bacteria and acetic acid bacteria and their applications.
Thananchai Dasri
Thananchai Dasri is Associate Professor of physics and materials science at the faculty of interdisciplinary studies, Khon Kaen University, Nong Khai Campus. He has published more than 20 scientific papers on optical and magneto-optical of nanomaterials and he attention in the last years on polymeric nanocomposites from agriculture waste products.
Sirinart Chio-Srichan
Sirinart Chio-Srichan is a doctoral researcher at the SAXS beamline at the Siam Photon Laboratory (SPL) of the Synchrotron Light Research Institute (Public Organization), Nakhon Ratchasima, Thailand. She has published mainly on synchrotron light applications.
Siriwat Soontaranon
Siriwat Soontaranon is a doctoral researcher at the SAXS beamline at the Siam Photon Laboratory (SPL) of the Synchrotron Light Research Institute (Public Organization), Nakhon Ratchasima, Thailand. He has published mainly on synchrotron light applications.
Wullapa Wongsinlatam
Wullapa Wongsinlatam received her Ph.D. degree from Khon Kaen University in 2013 and is an assistance professor in Faculty of Interdisciplinary Studies of Khon Kaen University. Her current research interests are mainly focused on applied mathematics, mathematical modeling, mathematical optimization, artificial neural network, metaheuristic optimization and programming.
Supachai Sompech
Supachai Sompech is assistance professor of materials science at the faculty of interdisciplinary studies, Khon Kaen University, Nong Khai Campus. He has published more than 10 scientific papers on nanomaterials and he attention in the last years on polymeric nanocomposites from agriculture waste products.
References
- Dubey P, Bhushan B, Sachdev A, et al. Silver-nanoparticle-incorporated composite nanofibers for potential wound-dressing applications. J Appl Polym Sci. 2015;132:42473.
- Gopiraman M, Jatoi AW, Hiromichi S, et al. Silver coated anionic cellulose nanofiber composites for an efficient antimicrobial activity. Carbohydr Polym. 2016;149:51–59.
- Ito H, Sakata M, Hongo C, et al. Cellulose nanofiber nanocomposites with aligned silver nanoparticles. Nanocomposites. 2018;4(4):167–177.
- Zhang X, Shi X, Gautrot JE, et al. Nanoengineered electrospun fibers and their biomedical applications: a review. Nanocomposites. 2021;7(1):1–34.
- Soemphol W, Charee P, Audtarat S, et al. Characterization of a bacterial cellulose-silica nanocomposite prepared from agricultural waste products. Mater Res Express. 2020;7(1):015085.
- Menon MP, Selvakumar R, Kumar PS, et al. Extraction and modification of cellulose nanofibers derived from biomass for environmental application. RSC Adv. 2017;7(68):42750–42773.
- Yang G, Xie J, Deng Y, et al. Hydrothermal synthesis of bacterial cellulose/AgNPs composite: a “green” route for antibacterial application. Carbohydr Polym. 2012;87(4):2482–2487.
- Maneerung D, Tokura S, Rujiravanit R. Impregnation of silver nanoparticles into bacterial cellulose for antimicrobial wound dressing. Carbohydr Polym. 2008;72(1):43–51.
- Kelly KL, Coronado E, Zhao EL, et al. The optical properties of metal nanoparticles: the influence of size, shape, and dielectric environment. J Phys Chem B. 2003;107(3):668–677.
- Chaiyachate P, Dasri T, Chingsungnoen A. Theoretical calculation of the optical absorption property of nanoparticles composed of an Au core and Si shell embedded in silica. Mater Res Express. 2020;7(1):015072.
- Chaiyachate P, Dasri T. Optical absorption and scattering properties of the active layer of perovskite solar cells incorporated silver nanoparticles. Orient J Chem. 2017;33(2):807–813.
- Iravani S, Korbekandi H, Mirmohammadi SV, et al. Synthesis of silver nanoparticles: chemical, physical and biological methods. Res Pharm Sci. 2014;9(6):385–406.
- Ahmed S, Ahmad M, Swami BL, et al. Green synthesis of silver nanoparticles using Azadirachta indica aqueous leaf extract. J Radiat Res Appl. 2016;9:1–7.
- Soemphol W, Hongsachart P, Tanamool V. Production and characterization of bacterial cellulose produced from agricultural by-product by Gluconacetobacter strains. Mater Today: Proc. 2018;5(5):11159–11168.
- Soontaranon S, Rugmai S. Small angle X-ray scattering at Siam photon laboratory. Chin J Phys. 2012;5(2):204–210.
- Beaucage G. Approximations leading to a unified exponential/Power-Law approach to small-angle scattering. J Appl Crystallogr. 1995;28(6):717–728.
- Breßler I, Kohlbrecher J, Thünemann AF. SASfit: a tool for small-angle scattering data analysis using a library of analytical expressions. J Appl Crystallogr. 2015;48(Pt 5):1587–1598.
- Ali K, Ahmed B, Dwivedi S, et al. Microwave accelerated green synthesis of stable silver nanoparticles with Eucalyptus globulus leaf extract and their antibacterial and antibiofilm activity on clinical isolates. PloS One. 2015;10(7):e0131178.
- Bakht Dalir SJ, Djahaniani H, Nabati F, et al. Characterization and the evaluation of antimicrobial activities of silver nanoparticles biosynthesized from Carya illinoinensis leaf extract. Heliyon. 2020;6(3):e03624.
- Roopan SM, Rohit Madhumitha G, et al. Low-cost and eco-friendly phyto-synthesis of silver nanoparticles using Cocos nucifera coir extract and its larvicidal activity. Ind Crops Prod. 2013;43:631–635.
- Sadeghi B, Gholamhoseinpoor F. A study on the stability and green synthesis of silver nanoparticles using Ziziphora tenuior (Zt) extract at room temperature. Spectrochim Acta Part A Mol Biomol Spectrosc. 2015;134:310–315.
- Sadeghi B, Rostami A, Momeni SS. Facile green synthesis of silver nanoparticles using seed aqueous extract of Pistacia atlantica and its antibacterial activity. Spectrochim Acta A Mol Biomol Spectrosc. 2015;134:326–332.
- Ulug B, Turkdemir MH, Cicek A, et al. Role of irradiation in the green synthesis of silver nanoparticles mediated by fig (Ficus carica) leaf extract. Spectrochim Acta A Mol Biomol Spectrosc. 2015;135:153–161.
- Ghosh S, Patil S, Ahire M, et al. Synthesis of silver nanoparticles using Dioscorea bulbifera tuber extract and evaluation of its synergistic potential in combination with antimicrobial agents. Int J Nanomedicine. 2012;7:483–496.
- Arunachalam KD, Annamalai SK. Chrysopogon zizanioides aqueous extract mediated synthesis, characterization of crystalline silver and gold nanoparticles for biomedical applications. Int J Nanomedicine. 2013;8:2375–2384.
- Gea S, Reynolds CT, Roohpour N, et al. Investigation into the structural, morphological, mechanical and thermal behaviour of bacterial cellulose after a two-step purification process. Bioresour Technol. 2011;102(19):9105–9110.
- Tokoh C, Takabe K, Sugiyama J, et al. Cp/mas 13c nmr and electron diffraction study of bacterial cellulose structure affected by cell wall polysaccharides. Cellulose. 2002;9(3/4):351–360.
- Castro C, Zuluaga R, Putaux JL, et al. Structural characterization of bacterial cellulose produced by Gluconacetobacter swingsii sp. from Colombian agroindustrial wastes. Carbohydr Polym. 2011;84(1):96–102.
- Vazquez A, Foresti ML, Cerrutti P, et al. Bacterial cellulose from simple and low cost production media by Gluconacetobacter xylinus. J Polym Environ. 2013;21(2):545–554.
- Xu Y, Li S, Yue X, et al. Review of silver nanoparticles(AgNPs)-cellulose antibacterial composites. BioRes. 2018;13(1):2150–2170.
- Santa MLC, Santos ALC, Oliveira PC, et al. Synthesis and characterization of silver nanoparticles impregnated into bacterial cellulose. Mater Chem. 2009;63(9–10):797–799.
- Agbabiaka A, Wiltfong M, Park C. Small angle X-Ray scattering technique for the particle size distribution of nonporous nanoparticles. J Nanopart. 2013;2013:1–11.
- Moodley JS, Krishna SBN, Pillay K, et al. Green synthesis of silver nanoparticles from Moringa oleifera leaf extracts and its antimicrobial potential. Adv Nat Sci: Nanosci Nanotechnol. 2018;9(1):015011.
- ErJaee H, Rajaian H, Nazifi S. Synthesis and characterization of novel silver nanoparticles using Chanmaemelum nobile extact for antibacterial application. Adv Nat Sci: Nanosci Nanotechnol. 2018;8(2):025004.
- Ebrahiminezhad A, Bagheri M, Taghizadeh SM, et al. Biomimetic synthesis of silver nanoparticles using microalgal secretory carbohydrates as a novel anticancer and antimicrobial. Adv Nat Sci: Nanosci Nanotechnol. 2016;7(1):015018.
- Shrivastava S, Bera T, Roy A, et al. Characterization of enhanced antibacterial effects of novel silver nanoparticles. Nanotechnology. 2007;18(22):225103.
- Feng QL, Wu J, Chen GQ, et al. A mechanistic study of the antibacterial effect of silver ions on Escherichia coli and Staphylococcus aureus. J Biomed Mater Res. 2000;52(4):662–668.
- Morones JR, Elechiguerra JL, Camacho A, et al. The bactericidal effect of silver nanoparticles. Nanotechnology. 2005;16(10):2346–2353.
- Ahmed S, Ahmad M, Swami BL, et al. A review on plants extract mediated synthesis of silver nanoparticles for antimicrobial applications: a green expertise. J Adv Res. 2016;7(1):17–28.