ABSTRACT
Candida spp. are the fourth leading cause of nosocomial blood stream infections in North America. Candida glabrata is the second most frequently isolated species, and rapid development of antifungal resistance has made treatment a challenge. In this study, we investigate the therapeutic potential of metformin, a biguanide with well-established action for diabetes, as an antifungal agent against C. glabrata. Both wild type and antifungal-resistant isolates of C. glabrata were subjected to biguanide and biguanide-antifungal combination treatment. Metformin, as well as other members of the biguanide family, were found to have antifungal activity against C. glabrata, with MIC50 of 9.34 ± 0.16 mg/mL, 2.09 ± 0.04 mg/mL and 1.87 ± 0.05 mg/mL for metformin, phenformin and buformin, respectively. We demonstrate that biguanides enhance the activity of several antifungal drugs, including voriconazole, fluconazole, and amphotericin, but not micafungin. The biguanide-antifungal combinations allowed for additional antifungal effects, with fraction inhibition concentration indexes ranging from 0.5 to 1. Furthermore, metformin was able to lower antifungal MIC50 in voriconazole and fluconazole-resistant clinical isolates of C. glabrata. We also observed growth reduction of C. glabrata with rapamycin and an FIC of 0.84 ± 0.09 when combined with metformin, suggesting biguanide action in C. glabrata may be related to inhibition of the mTOR complex. We conclude that the biguanide class has direct antifungal therapeutic potential and enhances the activity of select antifungals in the treatment of resistant C. glabrata isolates. These data support the further investigation of biguanides in the combination treatment of serious fungal infections.
Introduction
Invasive fungal infections due to non-Candida albicans species have been on the rise for the past two decades [Citation1,Citation2]. Candida glabrata, the most frequently isolated non-C. albicans species, accounts for around 21% of all Candida-related systemic bloodstream infections in North America [Citation3]. The rise in number of C. glabrata infections is particularly concerning, due to the high antifungal resistance of this species. Unlike most other Candida bloodstream infections, C. glabrata is intrinsically less susceptible to antifungal agents [Citation4]. This feature is especially true for the most widely used antifungal agent, fluconazole, with more than 10% of bloodstream infection isolates of C. glabrata showing high fluconazole resistance (MIC ≥ 64 μg/mL) [Citation5]. C. glabrata also demonstrates rapid secondary antifungal resistance, which is suspected to be related to C. glabrata haploid genome [Citation6].
A strategy to counter fluconazole-resistant infections is to use advanced and more potent synthetic derivative azole compounds. Voriconazole, for instance, is an azole whose antifungal spectrum is broader than fluconazole, with a potency 10 to 100 times greater against Candida species [Citation7,Citation8]. However, C. glabrata has rapidly developed resistance to voriconazole and other later generation azoles, presenting with higher MICs than those seen for most C. albicans isolates [Citation6]. The current first-line treatment for candidemia due to C. glabrata are echinocandin agents, such as micafungin, which are potent inhibitors of beta-glucan synthase, a critical component of the fungal cell wall [Citation1,Citation9]. Echinocandins are efficacious against fluconazole-resistant Candida species, however, during prolonged therapy, C. glabrata can develop reduced susceptibility even to this class [Citation10]. C. glabrata echinocandin resistance has increased from 4.9 to 12.3% between 2001 and 2010, and among the fluconazole-resistant isolates tested, 14.1% were fluconazole/echinocandin double-resistant [Citation11]. The prevalence of drug resistance in C. glabrata is projected to increase with rise in susceptible patient populations, as use of immunosuppressive therapy and invasive surgical procedures expand, while older and more complex patient populations are becoming increasingly common [Citation12–Citation15]. Additionally, the use of broad spectrum antibiotics and suboptimal dosing practices are significant contributors to the rise of drug resistance [Citation16]. These observations underscore the urgency to develop new antifungal strategies that may counter C. glabrata drug resistance.
Members of the biguanide drug class are defined by the common chemical feature of two guanidinium molecules joined by a single nitrogen, and include metformin, phenformin and buformin [Citation17]. All three compounds exhibit anti-diabetic properties by reducing circulating glucose levels. Metformin, being the most well-established oral medication for management of type II diabetes, is prescribed as mono-therapy or in combination with other anti-hyperglycemic medicines [Citation18–Citation21]. The global usage of metformin is estimated to be 150 million individuals worldwide [Citation22]. Recently, there has been interest surrounding the expanded therapeutic potential of metformin to include treatment and prevention of various types of cancer. Numerous clinical and preclinical studies suggest that metformin has anticancer properties, with a role in cancer stem cell suppression, epithelial-to-mesenchymal transition inhibition, and interference in cancer cell metabolism [Citation23–Citation25]. In addition, metformin is an effective adjuvant to several chemotherapy agents. A meta-analysis of clinical trials using metformin as an adjuvant demonstrated improved survival outcomes as compared with standard of care, especially in colorectal and prostate cancer patients [Citation25].
Metformin is thought to exert its anticancer effects through inhibition of mammalian target of rapamycin complex 1 (mTORC1), which has crucial functions in metabolism, growth, and proliferation [Citation26]. This metformin response pathway through mTORC1 is shown to be conserved across many organisms, from the nematode worms such as Caenorhabditis elegans to humans [Citation27]. Despite these observations, there are no known observational studies suggesting that patients using metformin have lower fungal infections. We hypothesized that the same growth-inhibition pathway may also exist for fungal species and sought to test the potential of metformin as an antifungal agent.
Here, we describe the therapeutic potential of metformin against the fungal pathogen C. glabrata and demonstrate that multiple members of the biguanide family exhibit an antifungal effect. We show that in biguanide-antifungal combination therapies, biguanides enhance efficacy of antifungal agents across multiple classes. The antifungal properties of metformin extend to drug-resistant isolates of C. glabrata, and, importantly, can augment the therapeutic efficacy of antifungal drugs toward these difficult to treat infections.
Results
Metformin exhibits antifungal properties
To determine if metformin has antifungal properties towards C. glabrata, we inoculated C. glabrata into different concentrations of metformin, incubated for 20–24 h, and measured yeast viability using Prestoblue viability dye conversion as well as by CFU on YPD plates. We observed a decreased growth of C. glabrata in the presence of metformin with a MIC50 of 9.34 ± 0.16 mg/mL, using the Prestoblue viability dye conversion assay (). Similarly, there was a significant reduction in CFU at metformin concentrations of 10 mg/mL and higher ()). Metformin is also effective against several other Candida species, including C. albicans, C. krusei. C. tropicalis, and C. parapsilosis, but not C. auris, although metformin sensitivity varies among the species ().
Table 1. MIC50 of various Candida species.
Figure 1. Metformin demonstrates antifungal activity against C. glabrata. (A) Prestoblue assay. Wild type C. glabrata were treated with a range of metformin concentrations, with Prestoblue fluorescence read at 18hrs. Arbitrary units (AU) for fluorescence plotted on the y-axis. Data points were fitted to a four-parameter logistic curve. (B) CFU assay. Wild type C. glabrata was treated with metformin for 18hrs, resuspended and plated onto YPD agar plates for 24-48hrs at 30°C. Data are plotted as fold population change compared to initial inoculum. ** denotes p ≤ 0.01, ns = not significant. Data represent 3 independent experiments.
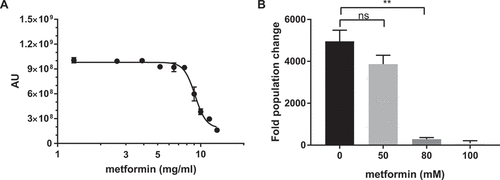
To determine whether this antifungal activity was unique to metformin or was a class effect of biguanides, we tested two additional commercially available biguanides. Phenformin and buformin, both more potent biguanides than metformin, also inhibited C. glabrata growth (). The MIC50 for phenformin was 2.09 ± 0.04 mg/mL, and for buformin 1.87 ± 0.05 mg/mL. To control for the high biguanide concentrations required in this study, we used the antibacterial agent ampicillin as a chemical concentration control for non-specific drug effect. At concentrations that the biguanides, including metformin, were effective in reducing C. glabrata growth, ampicillin did not exhibit any effect ()).
Figure 2. Antifungal activity is a shared feature of the biguanide family. Wild type C. glabrata were incubated with phenformin (A) or buformin (B) in the presence of Prestoblue for 18h at 30°C and arbitrary units for fluorescence determined (AU). (C) As a drug concentration control, C. glabrata was incubated with ampicillin. Data points were fitted to a four-parameter logistic curve and represent 3 independent experiments.
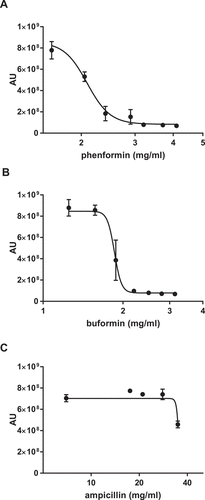
Previous studies have linked biguanide activity to mTOR, an essential regulator for growth and survival that is highly conserved among mammalian cell types. To assess if the antifungal effects of biguanides may also be mTOR related, chemical inhibition of mTORC1 with rapamycin was examined. Rapamycin exhibited a dose-dependent growth inhibition against C. glabrata with an MIC50 of 5–10 µg/mL (). Fractional inhibitory concentration index (ƩFIC) for rapamycin and metformin combinations was determined as a measurement of drug interaction between the two compounds. The combination is considered synergistic when the ΣFIC is ≤ 0.5, non-synergistic when the ΣFIC is ≥ 0.5 and ≤ 4, and antagonistic when ΣFIC is ≥ 4 [Citation28,Citation29]. Rapamycin and metformin ΣFIC was 0.84 ± 0.09, suggesting non-synergistic interaction between the two compounds. We then explored other upstream regulators of mTORC1, such as adenosine monophosphate-activated protein kinase (AMPK), an mTORC1 inhibitor, and alterations in cellular ATP levels. There was no effect on C. glabrata growth observed following treatment with the mitochondria complex I inhibitor rotenone ()), but there was a small but statistically significant dose dependent decrease in C. glabrata growth following treatment with the AMPK agonist 5-Aminoimidazole-4-Carboxamide-1-Beta-D-Ribofuranoside (AICAR) ()).
Figure 3. Inhibition of mTORC1 complex and AMPK, but not complex I, demonstrates anti-C. glabrata activity. Wild type C. glabrata were incubated with mTORC1 inhibitor rapamycin (A), mitochondrial complex I inhibitor rotenone (B), and AMPK agonist AICAR (C), in the presence of Prestoblue for 18h at 30°C. Yeast growth was expressed as arbitrary units for Prestoblue fluorescence (AU). *** denotes p ≤ 0.001, **** p ≤ 0.0001, ns = not significant. Data represent 3 independent experiments.
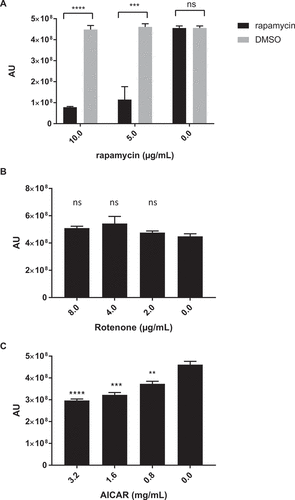
Metformin enhances activity of multiple classes of antifungals
We further explored the ability of biguanides to augment the activity of FDA-approved, existing antifungal therapies used for the treatment of C. glabrata infection. We examined combinations of three biguanides: metformin, phenformin, and buformin, with four antifungals: voriconazole, fluconazole, amphotericin B deoxycholate, and micafungin, and we determined ƩFIC for all 12 possible combinations (). All three biguanides had ƩFICs in the range of 0.5 < ƩFIC < 2 with voriconazole, fluconazole, and amphotericin. Biguanide combinations with micafungin, however, was not as effective, with ƩFICs consistently > 2.
Table 2. ƩFIC of biguanides and antifungals.
By introducing 6.5 mg/mL of metformin, a concentration without effect alone, to wild type C. glabrata, we were able to control yeast growth with lower concentrations of specific antifungal agents (). 6.5 mg/mL of metformin significantly lowered C. glabrata MIC50 to antifungals when combined with voriconazole, fluconazole, and amphotericin ()). In contrast, combination of metformin with micafungin had no influence on the micafungin MIC50. The enhanced antifungal activity of metformin-voriconazole combination was confirmed by CFU assay ()). Treatment with 6.5 mg/mL metformin and 40 ng/mL voriconazole reduced CFUs by 88% compared to C. glabrata treated with voriconazole alone.
Figure 4. Combination therapy of metformin with common antifungal agents reduces C. glabrata activity. (A) Wild type C. glabrata treated with 6.5 mg/mL metformin in combination with voriconazole, fluconazole, amphotericin or micafungin in the presence of Prestoblue for 18hrs at 30°C. AU represent Prestoblue fluorescence unit. Data points were fitted to a four-parameter logistic curve. (B) MIC50 were determined from four-parameter logistic curves comparing control antifungal drug with metformin combination. (C) C. glabrata was treated with voriconazole, metformin, or the combination for 18hrs, resuspended and plated onto YPD agar plates for 24-48hrs at 30°C. Data are plotted as fold population change compared to initial inoculum. * denotes p ≤ 0.05, ** p ≤ 0.01. Data represent 3 independent experiments.
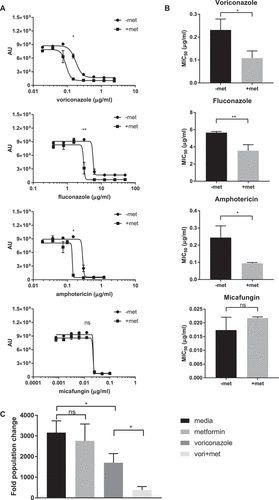
Metformin slows C. glabrata growth by prolonging yeast doubling time
To deepen our understanding of the process by which metformin affects C. glabrata growth overtime, we performed time-lapse microscopy with live C. glabrata dividing in the presence of metformin, voriconazole, or metformin-voriconazole combination treatment. While 6.5 mg/mL of metformin or 40 ng/mL voriconazole alone did not significantly change the rate of C. glabrata growth, combination treatment of metformin and voriconazole led to a drastic decrease in C. glabrata growth rate (Supplemental movie 1). Compared to C. glabrata that was untreated or treated with either metformin or voriconazole alone, C. glabrata grown in the combination of metformin-voriconazole exhibited slowed growth and smaller yeast clusters (). Our results suggest that the antifungal activity of metformin may be due to decreased rate of yeast division.
To further quantify these microscopic observations, we used a CFSE assay determine C. glabrata doubling time. Log phase C. glabrata were stained with CFSE as a fluorescent tracer of division. Yeast cells directly stained with CFSE are high in fluorescence intensity. During each cell division, percent of the undivided cell population decreases as additionally divided yeast cell numbers increase. Cells that have undergone division can be easily distinguished from undivided cells based upon CFSE fluorescence intensity. Using metformin-voriconazole combination treatment, we observed a higher percentage of bright undivided C. glabrata cells consistent with inhibition of yeast cell division as compared to no drug control (,)). The doubling time of C. glabrata increased significantly in the presence of metformin. Metformin alone significantly prolonged C. glabrata doubling time to 2.3h compared to untreated yeast at 2h, while metformin-voriconazole combination treatment prolonged doubling time to 3.5h ()).
Figure 5. Metformin reduces C. glabrata proliferation by slowing division rate. (A) Time lapse microscopy of live wild type C. glabrata over 20hrs in a humidified microscopy chamber at 30°C. Images show frame at 8hrs (supplemental video displays entire time course). Scale bar represents 50µm. (B) Flow cytometry division measurement of CFSE-labeled C. glabrata. CFSE-bright undivided yeast population shown at t = 0 is then incubated in media, metformin (6.5mg/ml), voriconazole (40µg/mL) or combination. Percent (scatter plot inset) of undivided CFSE-bright population was determined over time indicated. Representative scatter flow plots shown at 8hrs, and (C) percent undivided population shown over time. (D) C. glabrata doubling time was determined from flow cytometry CFSE measurements for yeast incubated in media, metformin, voriconazole and combination treatment. * denotes p ≤ 0.05, **** p ≤ 0.0001. Data represent a minimum of 3 independent experiments.
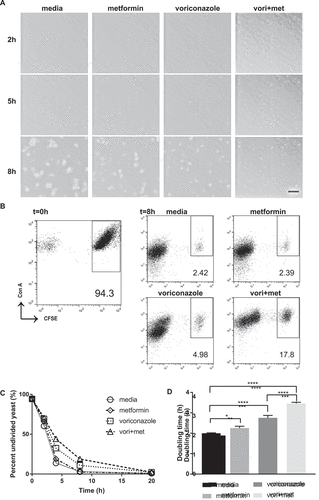
Metformin decreases antifungal MIC50 in drug-resistant C. glabrata strains
Since combining metformin with antifungals enhanced drug efficacy, we sought to determine if metformin can increase the antifungal susceptibility of drug-resistant strains of C. glabrata. Seven strains of resistant C. glabrata clinical isolates were subjected to combinations of metformin and antifungal agents. Of the isolated strains used, 3 isolates (FR-1, FR-2, FR-3) are fluconazole-resistant with moderate voriconazole resistance, 3 isolates (MR-1, MR-2, MR-3) are micafungin-resistant, and a single isolate, FR/MR, is fluconazole and micafungin double resistant. We established that all seven isolates are susceptible to metformin, with metformin MIC50 around 6.5 mg/mL, similar to wild type control. Isolate susceptibility to voriconazole, fluconazole, and micafungin was tested in the presence and absence of metformin. With metformin treatment, all 4 azole-resistant clinical isolates demonstrated a lowered antifungal MIC50 to voriconazole and fluconazole (). For the micafungin-resistant isolates as well as the double resistant FR/MR isolate, addition of metformin had no influence on micafungin MIC50 ()).
Figure 6. Metformin enhances antifungal activity against drug resistant C. glabrata isolates. Fluconazole-resistant clinical C. glabrata isolates were incubated with 3.9 mg/mL of metformin and voriconazole (A) or fluconazole (B). The MIC50 to each antifungal agent was determine with and without metformin. (C) Echinocandin and fluconazole double-resistant strain FR/MR was incubated with metformin and increasing amounts of micafungin. Micafungin MIC50 was determined for strain FR/MR with and without metformin. * denotes p ≤ 0.05, ** p ≤ 0.01. Data represent a minimum of 3 independent experiments.
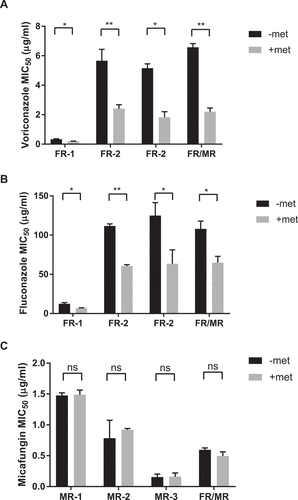
Discussion
The metformin-induced growth inhibition pathway is conserved across a wide variety of organisms [Citation27]. Despite the broad number of species studied, the effect of biguanides on fungal species has not previously been examined. In this study, we evaluated the antifungal properties of biguanides, both alone, and in combination with common antifungal agents. Our studies show that three biguanides – metformin, phenformin, and buformin – exhibit dose-dependent inhibition of C. glabrata as well as many other Candida species. In biguanide-antifungal combination therapies, the three biguanides combine favorably with voriconazole, fluconazole, and amphotericin, with ƩFICs ranging from 0.6 to 1. That these three biguanides demonstrate the same growth-inhibition and the same combination effect point to a likely common antifungal mechanism.
A significant limitation of these data is that the effective biguanide concentrations are beyond those normally achieved in vivo, in patient plasma [Citation30,Citation31]. The maximal approved total daily dose of metformin for treatment of diabetes is 35 mg/kg body weight, which results in a plasma concentration of 5–9 mg/L [Citation32]. Our studies utilize concentrations significantly higher, although use of control drug in this concentration range did not show toxicity, suggesting that the antifungal response exerted by biguanides are class specific. Limited biguanide entry into C. glabrata as compared to mammalian cells is one possible factor leading to the need for high drug levels. Even among human cells, intracellular metformin concentrations differ widely, preferentially accumulating in liver tissues as a result of high expression of the organic cation transporter 1 (OCT1), the main plasma-membrane metformin transporter [Citation33]. Overexpression of OCT1 can increase metformin uptake drastically [Citation34]. On the other hand, studies investigating the role of metformin in cancer cells, that lack OCT1 expression, often require concentrations similar to those used in our study [Citation23,Citation33]. The lack of appropriate membrane transporters in C. glabrata may hinder metformin uptake, thereby requiring the extreme concentrations used in these studies. These observations highlight the need for structural modifications of biguanides that may allow for heightened antifungal activity.
Our studies using time-lapse microscopy and flow cytometric yeast division analysis demonstrate a continuous slowing of cell division in metformin-treated C. glabrata. This reduction in growth rate points towards modulation of cellular metabolism or cell cycle activity as possible targets of the antifungal activity of metformin. Multiple possible molecular mechanisms of actions may contribute to biguanide antifungal activity. Of particular interest are mTORC1 pathways. Biguanides can limit mitochondrial complex I activity, lowering intracellular ATP and in turn elevating AMP [Citation35]. AMP accumulation prompts (AMPK) to deactivate mTORC1, while ATP deprivation causes changes in the nuclear pore complex conformation, restricting localization of mTORC1-activating Rag proteins [Citation27,Citation36]. Both pathways result in inhibition of mTORC1, which is a highly conserved signaling hub shared between fungi and mammalian cells [Citation37]. The mTORC1 complex is involved in regulation of a vast range of cellular protein target, having downstream effects in growth, proliferation, oxidative stress, and lipid metabolism [Citation38–Citation40]. The pathway has also been previously linked to antifungal activity with anti-Mucorales activity [Citation41]. We demonstrate mTOR inhibition also leads to reduction in C. glabrata metabolic activity, and that rapamycin-metformin combinations show non-synergistic drug interactions. Similarly, in nematode growth studies, such as C. elegans, biguanide-dependent inhibition of mTORC1 resulted in limited growth [Citation27]. In contrast, the mitochondria complex I inhibitor, rotenone, was effective in limiting C. elegans body size, but exerts no effect in C. glabrata. The AMPK agonist AICAR had no effect on C. elegans, but demonstrated a small, but statistically significant reduction in metabolic activity in C. glabrata, suggesting AMPK-dependent mTOR inhibition contributes to growth reduction in yeast. Additionally, the non-synergistic drug interactions between rapamycin and metformin suggest alternate metabolic pathways to mTOR may also be involved in biguanide activity. Both observations require further studies to delineate the precise biguanide-dependent molecular mechanisms in Candida.
Our data also suggest augmentation of current antifungals by metformin. Given that azole drugs function by inhibition of lanosterol 14α-demethylase in the ergosterol biosynthesis pathway [Citation42,Citation43] and polyenes by binding to ergosterol, these data raise sterol synthesis as a possible mechanism of metformin-induced antifungal activity. Interestingly, the lack of biguanide activity with echinocandins, potent inhibitors of beta-glucan synthase [Citation9], may either stem from the involvement of two distinct and independent pathways, or from influencing the same precise biochemical process. Biguanides can also alter ATP concentration, in turn, affecting ATP-binding cassette (ABC) transporter activity [Citation44]. ABC transporters are a major etiology of azole resistance in C. glabrata isolates [Citation44] and disruption of azole efflux could allow for more concentrated intracellular azoles. Micafungin, on the other hand, is not an efflux pump substrate [Citation45], potentially offering another explanation for the lack of effect with biguanide-micafungin combinations.
In summary, our findings suggest that in vitro, metformin, as well as other biguanides, demonstrate antifungal activity against C. glabrata. Biguanides possess antifungal alone but can also enhance antifungal efficacy of azole and polyene agents. Importantly, from a therapeutic perspective, biguanides lower the resistance of antifungal-resistant isolates to fungal drugs, specifically polyene and azole resistant isolates, suggesting combinatorial therapy may assist with clinical drug resistance. We have yet to explore the antifungal properties of biguanides in vivo as the high biguanide concentrations are currently unachievable. Intriguingly, in models of Mycobacteria tuberculosis infection, metformin enhanced host leukocyte activity, including metformin-associated reactive oxygen species production and autophagy promotion enhancing pathogen elimination [Citation46,Citation47]. Whether metformin could enhance the antifungal activity of human leukocytes is not known, however it is possible that in addition to the direct antifungal effects of metformin, there may be an effect on antifungal immunity. While the molecular mechanisms of biguanide antifungal activity require closer examination, these data suggest that biguanides offer a potential new therapeutic modality alone or in combination antifungal therapy.
Materials and methods
Reagents
Metformin hydrochloride was purchased from Cayman Chemicals. Fluconazole, ampicillin sodium, rapamycin, and other biguanide hydrochlorides including phenformin and buformin were purchased from Sigma. Clinical grade formulations of the antifungal agents voriconazole, amphotericin B deoxycholate, and micafungin were purchased from the Massachusetts General Hospital (MGH) clinical pharmacy. All reagents were dissolved in distilled water or DMSO according to the manufacturer’s recommendation, and stored at −20°C. Cell culture media include liquid YPD (1% yeast extract, 2% peptone, 2% dextrose); YPD agar (1% yeast extract, 1% peptone, 2% dextrose, 2% agar); RPMI-MOPS (RPMI 1640 containing 2% glucose and 0.165M MOPS, buffered at pH7); and complete RPMI (RPMI 1640 w/2mM L-glutamine, 10% heat-inactivated fetal bovine serum, 1% penicillin-streptomycin).
Candida strains and culture conditions
Wild type C. glabrata and wild type SC5314 C. albicans were purchased from the American Type Culture Collection (ATCC, Manassas, VA). Clinical strains of other Candida species were obtained from the MGH microbiology lab or Dr. Dimitrios Kontonyanis (MDAnderson Cancer Center). Drug resistant isolates included one dose-dependent fluconazole resistant strain (Strain FR-1), two fluconazole-resistant strains (FR-2, FR-3), three micafungin-resistant strains (MR-1, MR-2, MR-3), and one fluconazole-micafungin double-resistant strain (FR/MR), as determined by Clinical Laboratory and Standards Institute (CSLI) criteria [Citation48]. Antifungal susceptibility testing was confirmed by the MGH microbiology lab using microplate dilution and summarized in Supplemental . Yeast were grown overnight in liquid YPD at 30°C with shaking, washed three times in PBS after collection, counted with a Luna automated cell counter (Logos Biosystems), and resuspended in PBS at the desired inoculum.
Drug susceptibility testing
Biguanide drug testing was adopted from CLSI microdilution antifungal testing [Citation48]. Candida strains were incubated in RPMI-MOPS media at an inoculum of 200 yeasts per 100µl of media, and the indicated concentration of test drug. Prestoblue Cell Viability Reagent (Thermo Fisher) was added to 10% of the final volume. Cells were incubated at 35°C. After 20-24h of incubation, the MIC was determined based on fluorescence reading at 560/590nm using a SpectraMax i3x reader (Molecular Devices) [Citation49]. The MIC50 was determined using GraphPad Prism 7 (GraphPad Software) non-linear curve fit equation. Log-transformed concentration values and Prestoblue dye fluorescence data were fitted to a four-parameter logistic equation, from which the concentration at 50% maximal value was extracted as the MIC50. To determine yeast viability after drug exposure and confirm Prestoblue measurements, colony forming unit (CFU) assays were performed. After incubation as above, yeasts were serially diluted in distilled water and plated on YPD plates. CFU were determined manually after 48 hours of incubation at 30°C. Initial inoculum was also plated to confirm the starting number of yeast cells.
Fractional Inhibitory Concentration (FIC) index determination
Mode of drug interaction in combinations of biguanides and antifungals were determined by assessing the fractional inhibitory concentration index (ΣFIC) through the checkerboard method [Citation28]. Following inoculation, checkerboard plates were incubated at 35°C and read at 560/590nm fluorescence after 18 hours. MIC was defined as the lowest concentration of drug or drug combination that completely inhibited growth of the organism as determined by fluorescence reading. FIC and FIC indexes (ΣFIC) were determined as described previously, where the combination is considered synergistic when the ΣFIC is ≤ 0.5, non-synergistic when the ΣFIC is ≥ 0.5 and ≤ 4, and antagonistic when ΣFIC is ≥ 4 [Citation28,Citation29].
Flow cytometric analysis of yeast doubling time
Wild type C. glabrata proliferation was determined by carboxyfluorescein diacetate succinimidyl ester (CFSE) labeling. Similar to cell wall labeling with FITC [Citation50], CFSE allows for tracing of multiple generations of yeast division by dye dilution, giving rise to highly fluorescent undivided cells and divided cell populations with lower fluorescence intensity. Wild type C. glabrata were grown to log phase in liquid YPD, washed in PBS, and stained with 25µg/mL CFSE (Sigma) for 30 min at 30°C on a vertical rotator. Yeasts were washed in PBS containing 2% bovine serum albumin (BSA) to remove excess CFSE. Stained yeast cells were passed through a 25G 7/8-inch needle to dissociate clumped cells.
CFSE stained C. glabrata was inoculated in MOPS-RPMI media with desired concentrations of biguanide and antifungal agents. At the desired time points, yeast cells were washed and fixed in 3.7% formalin and labeled with 3µg/mL concanavalin A conjugated to Alexa Fluor 647 (Thermo Fisher). Flow acquisition was done with a FACS Calibur flow cytometer (Becton-Dickinson), using CellQuest software (Becton-Dickinson). After expressing ConA-647 on the y-axis and CFSE on the x-axis, the percentage of CFSE bright undivided population was determined using FlowJo 10 software (FlowJo, Ashland, OR). To determine yeast doubling time, percentage of undivided population over time was fitted to a non-linear one phase exponential decay curve fit equation in GraphPad Prism 7.
Microscopy
Wild type C. glabrata was plated onto 8-chambered coverslip slides (LabTek, Thermo Scientific) and allowed to proliferate in MOPS-RPMI in the presence of metformin, voriconazole, or metformin-voriconazole combined treatment. Slides were mounted on a Nikon Ti-E inverted microscope equipped with an EM-CCD camera (Hamamatsu, C9100-13). For live cell imaging, the microscopy chamber, housed in an environmental chamber, was humidified and set to 30°C [Citation51]. Images were acquired using MetaMorph software (Molecular Devices).
Statistics
Statistical calculations were performed using GraphPad Prism 7 software. Data were analyzed by two-tailed unpaired t test, or one-way ANOVA test, where appropriate, and were considered significant when p ≤ 0.05.
Supplemental Material
Download MS Word (13.4 KB)Disclosure statement
No potential conflict of interest was reported by the authors.
Supplementary material
Supplemental data for this article can be accessed here.
Additional information
Funding
Notes on contributors
Michael K. Mansour
S.X., M.K.M., D.B.S., A.A.S., J.L.R. contributed to experimental design. S.X., P.E.N., Z.D.M., M.K.M. executed the experiments. S.X., M.K.M., D.B.S., A.A.S., J.L.R., N.K., P.E.N., M.B.F., A.L., D.L., M.F., J.M.T performed analysis and interpretation of experiments. S.X., M.K.M., Z.D.M. contributed to writing the manuscript.
References
- Rodrigues CF, Silva S, Henriques M. Candida glabrata: a review of its features and resistance. Eur J Clin Microbiol. 2014 May;33(5):673–688. PubMed PMID: 24249283.
- Silva S, Henriques M, Hayes A, et al. Candida glabrata and Candida albicans co-infection of an in vitro oral epithelium. J Oral Pathol Med. 2011 May;40(5):421–427. PubMed PMID: 21158929.
- Sardi JC, Scorzoni L, Bernardi T, et al. Candida species: current epidemiology, pathogenicity, biofilm formation, natural antifungal products and new therapeutic options. J Med Microbiol. 2013 Jan;62(Pt 1):10–24. PubMed PMID: 23180477.
- Henry KW, Nickels JT, Edlind TD. Upregulation of ERG genes in Candida species by azoles and other sterol biosynthesis inhibitors. Antimicrob Agents Chemother. 2000 Oct;44(10):2693–2700. PubMed PMID: 10991846; PubMed Central PMCID: PMC90137.
- Pfaller MA, Messer SA, Hollis RJ, et al. Variation in susceptibility of bloodstream isolates of Candida glabrata to fluconazole according to patient age and geographic location in the United States in 2001 to 2007. J Clin Microbiol. 2009 Oct;47(10):3185–3190. PubMed PMID: 19656983; PubMed Central PMCID: PMC2756923.
- Fidel PL Jr., Vazquez JA, Sobel JD. Candida glabrata: review of epidemiology, pathogenesis, and clinical disease with comparison to C. albicans. Clin Microbiol Rev. 1999 Jan;12(1):80–96. PubMed PMID: 9880475; PubMed Central PMCID: PMC88907.
- Masia Canuto M, Gutierrez Rodero F. Antifungal drug resistance to azoles and polyenes. Lancet Infect Dis. 2002 Sep;2(9):550–563. PubMed PMID: 12206971.
- Pfaller MA, Diekema DJ. Epidemiology of invasive candidiasis: a persistent public health problem. Clin Microbiol Rev. 2007 Jan;20(1):133–163. PubMed PMID: 17223626; PubMed Central PMCID: PMC1797637.
- Cross SA, Scott LJ. Micafungin: a review of its use in adults for the treatment of invasive and oesophageal candidiasis, and as prophylaxis against Candida infections. Drugs. 2008;68(15):2225–2255. PubMed PMID: 18840009.
- Spreghini E, Orlando F, Sanguinetti M, et al. Comparative effects of micafungin, caspofungin, and anidulafungin against a difficult-to-treat fungal opportunistic pathogen, Candida glabrata. Antimicrob Agents Chemother. 2012 Mar;56(3):1215–1222. PubMed PMID: 22203604; PubMed Central PMCID: PMC3294882.
- Alexander BD, Johnson MD, Pfeiffer CD, et al. Increasing echinocandin resistance in Candida glabrata: clinical failure correlates with presence of FKS mutations and elevated minimum inhibitory concentrations. Clin Infect Dis. 2013 Jun;56(12):1724–1732. PubMed PMID: 23487382; PubMed Central PMCID: PMC3658363.
- Cruciani M, Serpelloni G. Management of Candida infections in the adult intensive care unit. Expert Opin Pharmacother. 2008 Feb;9(2):175–191. PubMed PMID: 18201143.
- Horn DL, Neofytos D, Anaissie EJ, et al. Epidemiology and outcomes of candidemia in 2019 patients: data from the prospective antifungal therapy alliance registry. Clin Infect Dis. 2009 Jun 15;48(12):1695–1703. PubMed PMID: 19441981.
- Kojic EM, Darouiche RO. Candida infections of medical devices. Clin Microbiol Rev. 2004 Apr;17(2):255–267. PubMed PMID: 15084500; PubMed Central PMCID: PMC387407.
- Shoham S, Marr KA. Invasive fungal infections in solid organ transplant recipients. Future Microbiol. 2012 May;7(5):639–655. PubMed PMID: 22568718; PubMed Central PMCID: PMC4222063.
- Bennett JE, Izumikawa K, Marr KA. Mechanism of increased fluconazole resistance in Candida glabrata during prophylaxis. Antimicrob Agents Chemother. 2004 May;48(5):1773–1777. PubMed PMID: 15105134; PubMed Central PMCID: PMC400565.
- Biguanides SG. A review of history, pharmacodynamics and therapy. Diabete Metab. 1983 May-Jun;9(2):148–163. PubMed PMID: 6352352.
- Romero R, Erez O, Huttemann M, et al. Metformin, the aspirin of the 21st century: its role in gestational diabetes mellitus, prevention of preeclampsia and cancer, and the promotion of longevity. Am J Obstet Gynecol. 2017 Sep;217(3):282–302. PubMed PMID: 28619690.
- Strack T. Metformin: a review. Drugs Today. 2008 Apr;44(4):303–314. PubMed PMID: 18536788.
- Bridges HR, Jones AJ, Pollak MN, et al. Effects of metformin and other biguanides on oxidative phosphorylation in mitochondria. Biochem J. 2014 Sep 15;462(3):475–487. PubMed PMID: 25017630; PubMed Central PMCID: PMC4148174.
- Viollet B, Guigas B, Sanz Garcia N, et al. Cellular and molecular mechanisms of metformin: an overview. Clin Sci. 2012 Mar;122(6):253–270. PubMed PMID: 22117616; PubMed Central PMCID: PMC3398862.
- Pryor R, Cabreiro F. Repurposing metformin: an old drug with new tricks in its binding pockets. Biochem J. 2015 Nov 1;471(3):307–322. PubMed PMID: 26475449; PubMed Central PMCID: PMC4613459.
- Gong J, Kelekar G, Shen J, et al. The expanding role of metformin in cancer: an update on antitumor mechanisms and clinical development. Target Oncol. 2016 Aug;11(4):447–467. 10.1007/s11523-016-0423-z. PubMed PMID: 26864078.
- Kasznicki J, Sliwinska A, Drzewoski J. Metformin in cancer prevention and therapy. Ann Transl Med. 2014 Jun;2(6):57. PubMed PMID: 25333032; PubMed Central PMCID: PMC4200668.
- Coyle C, Cafferty FH, Vale C, et al. Metformin as an adjuvant treatment for cancer: a systematic review and meta-analysis. Ann Oncol. 2016 Dec;27(12):2184–2195. PubMed PMID: 27681864; PubMed Central PMCID: PMC5178140.
- Chiang GG, Abraham RT. Targeting the mTOR signaling network in cancer. Trends Mol Med. 2007 Oct;13(10):433–442. PubMed PMID: 17905659.
- Wu L, Zhou B, Oshiro-Rapley N, et al. An ancient, unified mechanism for metformin growth inhibition in C. elegans and cancer. Cell. 2016 Dec 15;167(7):1705–1718 e13. PubMed PMID: 27984722; PubMed Central PMCID: PMC5390486.
- Orhan G, Bayram A, Zer Y, et al. Synergy tests by E test and checkerboard methods of antimicrobial combinations against Brucella melitensis. J Clin Microbiol. 2005 Jan;43(1):140–143. PubMed PMID: 15634962; PubMed Central PMCID: PMC540140.
- Meletiadis J, Pournaras S, Roilides E, et al. Defining fractional inhibitory concentration index cutoffs for additive interactions based on self-drug additive combinations, Monte Carlo simulation analysis, and in vitro-in vivo correlation data for antifungal drug combinations against Aspergillus fumigatus. Antimicrob Agents Chemother. 2010 Feb;54(2):602–609. PubMed PMID: 19995928; PubMed Central PMCID: PMC2812160.
- Lalau JD, Lemaire-Hurtel AS, Lacroix C. Establishment of a database of metformin plasma concentrations and erythrocyte levels in normal and emergency situations. Clin Drug Investig. 2011;31(6):435–438. PubMed PMID: 21401215.
- Kajbaf F, De Broe ME, Lalau JD. Therapeutic concentrations of metformin: a systematic review. Clin Pharmacokinet. 2016 Apr;55(4):439–459. PubMed PMID: 26330026.
- He L, Wondisford FE. Metformin action: concentrations matter. Cell Metab. 2015 Feb 3;21(2):159–162. PubMed PMID: 25651170.
- Chandel NS, Avizonis D, Reczek CR, et al. Are metformin doses used in murine cancer models clinically relevant? Cell Metab. 2016 Apr 12;23(4):569–570. PubMed PMID: 27076070.
- Chien HC, Zur AA, Maurer TS, et al. Rapid method to determine intracellular drug concentrations in cellular uptake assays: application to metformin in organic cation transporter 1-transfected human embryonic kidney 293 cells. Drug Metab Dispos. 2016 Mar;44(3):356–364. PubMed PMID: 26700958.
- Owen MR, Doran E, Halestrap AP. Evidence that metformin exerts its anti-diabetic effects through inhibition of complex 1 of the mitochondrial respiratory chain. Biochem J. 2000 Jun 15;348(Pt 3):607–614. PubMed PMID: 10839993; PubMed Central PMCID: PMC1221104.
- Hinke SA, Martens GA, Cai Y, et al. Methyl succinate antagonises biguanide-induced AMPK-activation and death of pancreatic beta-cells through restoration of mitochondrial electron transfer. Br J Pharmacol. 2007 Apr;150(8):1031–1043. PubMed PMID: 17339833; PubMed Central PMCID: PMC2013909.
- Goberdhan DC, Wilson C, Harris AL. Amino acid sensing by mTORC1: intracellular transporters mark the spot. Cell Metab. 2016 Apr 12;23(4):580–589. PubMed PMID: 27076075; PubMed Central PMCID: PMC5067300.
- Dowling RJ, Topisirovic I, Fonseca BD, et al. Dissecting the role of mTOR: lessons from mTOR inhibitors. Biochim Biophys Acta. 2010 Mar;1804(3):433–439. PubMed PMID: 20005306.
- Hur KY, Lee MS. New mechanisms of metformin action: focusing on mitochondria and the gut. J Diabetes Investig. 2015 Nov;6(6):600–609. PubMed PMID: 26543531; PubMed Central PMCID: PMC4627534.
- Laplante M, Sabatini DM. mTOR signaling in growth control and disease. Cell. 2012 Apr 13;149(2):274–293. PubMed PMID: 22500797; PubMed Central PMCID: PMC3331679.
- Bastidas RJ, Shertz CA, Lee SC, et al. Rapamycin exerts antifungal activity in vitro and in vivo against Mucor circinelloides via FKBP12-dependent inhibition of Tor. Eukaryot Cell. 2012 Mar;11(3):270–281. PubMed PMID: 22210828; PubMed Central PMCID: PMC3294450.
- Johnson LB, Kauffman CA. Voriconazole: a new triazole antifungal agent. Clin Infect Dis. 2003 Mar 01;36(5):630–637. PubMed PMID: 12594645.
- Zervos M, Meunier F. Fluconazole (Diflucan): a review. Int J Antimicrob Agents. 1993;3(3):147–170. PubMed PMID: 18611557.
- Prasad R, Rawal MK. Efflux pump proteins in antifungal resistance. Front Pharmacol. 2014;5:202. PubMed PMID: 25221515; PubMed Central PMCID: PMC4148622.
- Cannon RD, Lamping E, Holmes AR, et al. Efflux-mediated antifungal drug resistance. Clin Microbiol Rev. 2009 Apr;22(2):291–321. Table of Contents. PubMed PMID: 19366916; PubMed Central PMCID: PMC2668233.
- Kaufmann SHE, Dorhoi A, Hotchkiss RS, et al. Host-directed therapies for bacterial and viral infections. Nat Rev Drug Discov. 2017 Sep 22;(17):35–56. PubMed PMID: 28935918.
- Singhal A, Jie L, Kumar P, et al. Metformin as adjunct antituberculosis therapy. Sci Transl Med. 2014 Nov 19;6(263):263ra159. PubMed PMID: 25411472.
- Alastruey-Izquierdo A, Melhem MS, Bonfietti LX, et al. Susceptibility test for fungi: clinical and laboratorial correlations in medical mycology. Rev Inst Med Trop Sao Paulo. 2015 Sep;57(Suppl 19):57–64. PubMed PMID: 26465371; PubMed Central PMCID: PMC4711191.
- Chapin KC, Musgnug MC. Evaluation of Sensititre automated reading and incubation system for automated reading of Sensititre broth microdilution susceptibility plates. J Clin Microbiol. 2004 Feb;42(2):909–911. PubMed PMID: 14766887; PubMed Central PMCID: PMC344475.
- Seider K, Brunke S, Schild L, et al. The facultative intracellular pathogen Candida glabrata subverts macrophage cytokine production and phagolysosome maturation. J Immunology. 2011 Sep 15;187(6):3072–3086. PubMed PMID: 21849684.
- Mansour MK, Tam JM, Khan NS, et al. Dectin-1 activation controls maturation of beta-1,3-glucan-containing phagosomes. J Biol Chem. 2013 May 31;288(22):16043–16054. PubMed PMID: 23609446; PubMed Central PMCID: PMC3668760.