ABSTRACT
Hypoxia-inducible factor 1 (HIF-1) is a transcription factor that plays critical roles during the cellular response to hypoxia. Under normoxic conditions, its function is tightly regulated by the degradation of its alpha subunit (HIF-1α), which impairs the formation of an active heterodimer in the nucleus that otherwise regulates the expression of numerous genes. Importantly, HIF-1 participates in both cancer and infectious diseases unveiling new therapeutic targets for those ailments. Here, we discuss aspects related to the activation of HIF-1, the effects of this transcription factor over immune system components, as well as the involvement of HIF-1 activity in response to viral infections in humans. Although HIF-1 is currently being assessed in numerous clinical settings as a potential therapy for different diseases, up to date, there are no clinical studies evaluating the pharmacological modulation of this transcription factor as a possible new antiviral treatment. However, based on the available evidence, clinical trials targeting this molecule are likely to occur soon. In this review we discuss the role of HIF-1 in viral immunity, the modulation of HIF-1 by different types of viruses, as well as the effects of HIF-1 over their life cycle and the potential use of HIF-1 as a new target for the treatment of viral infections.
Introduction
Hypoxia-inducible factor 1 (HIF-1) was described for the first time in 1992 as a critical regulator of oxygen tension levels in human hepatocellular carcinoma cells (Hep3B) [Citation1]. Later, HIF-1 was characterized as a heterodimeric DNA-binding protein complex belonging to the PER-ARNT-SIM (PAS) family related to circadian rhythmicity in diverse organisms, voltage-activated potassium channels, and hydrogen sensing, among others [Citation2]. HIF-1 consists of two polypeptide subunits with helix-loop-helix motifs/domains that are constitutively expressed in the cell [Citation3]. This heterodimer is composed of an oxygen-regulated α subunit and a β subunit [Citation4]. Currently, there are three known isoforms of the α subunit, namely HIF-1α, HIF-2α, and HIF-3α, with all three encoded in different loci and regulating distinct cellular functions, which give rise to three different proteins: HIF-1, HIF-2 and HIF-3, respectively [Citation3, Citation5, Citation6]. Furthermore, the α subunits have different isoforms, with eight alternative splicing variants of the HIF-1α subunit, and 10 known isoforms of the HIF-3α subunit [Citation7, Citation6]. Up to date, splice variants of the HIF-2α subunit have not been described. While HIF-1 mainly regulates glycolysis and angiogenesis, HIF-2 stimulates erythropoiesis [Citation4], promotes the growth and metastasis of neuroblastomas, and activates the expression of target genes that modulate vascular function and angiogenesis in embryonic vascular endothelial cells, among other functions [Citation5] . On the other hand, the role of HIF-3 has remained somewhat poorly described. Interestingly, a new study has suggested a possible role for HIF-3α, isoform 2 as a transcriptional activator that regulates erythropoietin expression [Citation8] . Also, it has been described that HIF-3 may inhibit the activity of both, HIF-1 and HIF-2 [Citation6] . Under hypoxic conditions (<~1% O2), the HIF-1α subunit is activated and translocated to the nucleus, where it forms a heterodimer with the HIF-1β subunit and binds to the hypoxia response element (HRE) sequence in the regulatory regions of target genes. Combined with transcriptional coactivators recruited to neighboring sites, HIF-1 can induce the transcription of numerous genes [Citation4,Citation9].
Figure 1. Schematic representation of HIF-regulated pathways
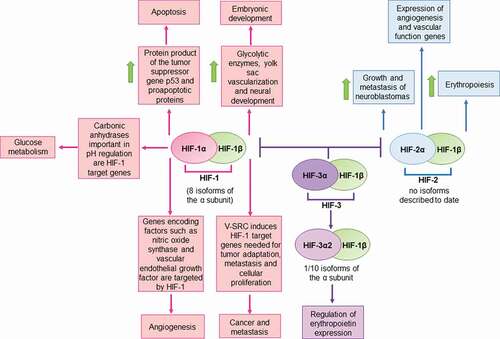
Different studies have reported a variety of genes with different functions that are targeted by HIF-1. A set of genes induced by HIF-1 are involved in embryonic development [Citation10, Citation11]. Importantly, a study reported that the loss of HIF-1α in embryonic stem cells (ESCs) led to a reduction in the expression of glycolytic enzymes, such as phosphoglycerate kinase 1 (PGK), lactate dehydrogenase A (LDH), aldolase A (ALDA) and glucose transporter 1 (GLUT-1). HIF-1α null embryos showed increased hypoxia and apoptosis, disorganized yolk sac vascularization, as well as abnormal neural development and cephalic vascularization [Citation11] . It has also been reported that transfection of cDNA encoding HIF-1α in murine ESCs promoted cardiogenesis, and that the activation of this transcription factor favored the differentiation and maturation of ESC-derived cardiomyocytes [Citation10]. Other studies report a role for HIF-1 in tumor growth and cancer metastasis. For instance, V-SRC which is a transforming oncogene found in the Rous sarcoma virus, induces signal transduction pathways that elicit HIF-1, and HIF-1-regulated gene expression, such as vascular endothelial growth factor (VEGF) and enolase 1 (ENO1). Such factors may promote tumor adaptation to an hypoxic environment, which seem to be necessary for maintaining cellular proliferation, metastasis and reducing cell death [Citation12, Citation13] . HIF-1 has also been described to be involved in apoptosis. Indeed, HIF-1α has been described as capable of increasing and stabilizing the product of the tumor suppressor gene p53 and upregulating proapoptotic proteins, such as BNIP3 which has a promoter containing an HRE [Citation14–16] . Another process modulated by HIF-1 is angiogenesis. Genes that encode factors that are involved in different steps of this process have been described to be targeted by HIF-1, such as: nitric oxide synthase which participates in vasodilatation, vascular endothelial growth factor which increases vascular permeability, and collagen prolyl-4-hydroxylase that is involved in the degradation of the extracellular matrix during angiogenesis [Citation17–20]. . An additional process modulated by HIF-1 is glucose metabolism. Under hypoxic conditions, anaerobic glycolysis becomes activated, which induces lactate production and consequently a reduction in pH [Citation21]. Importantly, transmembrane carbonic anhydrases, which play an important role in regulating pH have been shown to be HIF-1-target genes [Citation22], among others .
Importantly, a large amount of evidence suggests that HIF-1, and more specifically HIF-1α, plays a significant role in infectious and inflammatory diseases [Citation23, Citation24]. For example, this transcription factor plays a role in the outcome of infections by different human pathogens, such as bacteria (e.g., Staphylococcus aureus, Escherichia coli, and Acinetobacter baumannii), fungi (e.g., Tinea rubrum), parasites (e.g., Leishmania donovani), and viruses (discussed in detail below) [Citation25, Citation26, Citation24]. Numerous stimuli modulate this transcription factor’s expression during infections, which widely vary among pathogens [Citation27]. There is also evidence showing that HIF-1α may be directly involved in the host response to pathogens through the modulation of immune cell functions, as we will further discuss in the following sections [Citation28]. Considering all this, the transcription factor HIF-1 could be a potential target for novel therapies against some pathogens.
Here, we expand on previous studies and review articles that connect viral infections with HIF-1 [Citation29, Citation27], by discussing additional viruses and recently-available literature related to HIF-1α and viral infections. Furthermore, we discusses the role of HIF-1 in the immune responses to viruses and potential new antiviral treatments based on HIF-1 modulation.
Activation of HIF-1α
Both transcription and translation of HIF-1α mRNA are constitutive and are not affected by oxygen levels, overall remaining constant in the cell under normoxic (~21% O2) conditions [Citation4,Citation30]. However, the HIF-1α subunit has a half-life of approximately 5 minutes. Thus, due to its rapid degradation during normoxia, it is almost undetectable [Citation4]. Under these conditions, the von Hippel-Lindau (pVHL) ubiquitin E3 ligase complex tags HIF-1α with ubiquitin leading to its rapid degradation [Citation31]. Binding of pVHL with HIF-1α requires hydroxylation of two proline residue by the prolyl-hydroxylase domain proteins (PHDs). These proteins require oxygen, Fe2+, and ascorbate as cofactors for the hydroxylation process [Citation4]. When oxygen levels decrease, the degradation of HIF-1α no longer occurs, as PHDs are not active, and no proline residues in pVHL are modified. This degradation abrogates the interaction between pVHL and HIF-1α. Lack of this interaction results in the stabilization of HIF-1α and its translocation from the cytoplasm to the nucleus [Citation32,Citation33]. Under these circumstances, HIF-1α dimerizes with HIF-1β in the nucleus to form the HIF-1α/β heterodimer or HIF-1, which is transcriptionally active [Citation34, Citation30].
HIF-1α contains two transactivation domains: the N-terminal Transactivation Domain (N-TAD) and the C-Terminal Transactivation Domain (C-TAD). Under normoxic conditions, an asparagine residue in the C-TAD is hydroxylated by the asparagine hydroxylase factor inhibiting HIF-1 (FIH-1), which is also oxygen-dependent [Citation3,Citation4]. This hydroxylation prevents the recruitment of transcriptional coactivators that bind to this region of HIF-1α, such as CBP/p300 [Citation4]. Under hypoxic conditions, the hydroxylation of this asparagine is suppressed, allowing the interaction between C-TAD and CBP/p300 [Citation9].Interestingly, several viruses induce the degradation of PHDs as a mechanism to activate HIF-1α during infections, although there is also evidence indicating that some viruses may alternatively use other pathways to activate HIF-1α. For instance, infection with viruses such as hepatitis C or hepatitis B can promote processes that induce oxidative stress in the cell, influencing the stability of HIF-1α after infection [Citation27]. Alternatively, other viruses such as Epstein-Barr virus may use intracellular kinases to induce the activation of HIF-1α, which is further discussed below [Citation27].
HIF-1α and immunity
The contribution of HIF-1 to some types of immune responses has been widely studied and extensively reviewed by Palazon et al. and Hellwig-Bürgel et al. [Citation28,Citation35]. This review focuses on the role of HIF-1 in viral infections and its role in the context of such immune responses, focusing on innate and adaptive immune components.
Innate immune components are responsible for immediate and early recognition of events after pathogen infection and are aimed to rapidly counteract their presence and replication [Citation36]. As constant interactions occur between different types of immune cells, the innate immune response will likely influence the following adaptative immune response elicited by the host against the pathogen [Citation37].
Previous studies have shown that inhibition of serine/threonine kinases and phosphatases can modulate HIF-1 activity, as treating cells with genistein -an inhibitor of tyrosine kinase- has significant effects on the transcriptional activity of HIF-1 [Citation38, Citation39, Citation40]. However, the exact mechanisms regulating this activity are somewhat controversial due to contradictory reports on both positive and negative regulation of HIF-1 activity [Citation41, Citation42]. Activation of the PI3/Akt pathway increases HIF-1α protein levels [Citation43]. Consistently, inhibition of mTOR -and, therefore, inhibition of PI3/Akt- reduces the synthesis of HIF-1α [Citation44, Citation45, Citation46]. Noteworthy, NF-κB is activated during hypoxia and involves the activation of IκB kinase-β in HeLa cells [Citation47]. This activation inhibits neutrophil apoptosis via NF-κB, resulting in sustained inflammation [Citation48]. Thus, inhibition of HIF-1α or PHDs could be used as a strategy for dampening exacerbated inflammatory responses. There is no evidence showing that MAPK levels affect the stabilization of HIF-1α, although a study reported that they might increase the trans-activation ability of HIF-1 [Citation28].
Interferons are a group of cytokines that can either alert cells for limiting viral replication (mainly type-I interferons) or modulate immune system components (mainly type-II interferons) for virus control [Citation49]. Under hypoxic conditions, the antiviral effects of IFN-α and IFN-γ are higher than in aerobic conditions, yet the mechanisms underlying these observations are unknown. However, it has been hypothesized that hypoxia may increase the expression of the corresponding IFN receptors, increasing signal transduction, or expression of IFN-stimulated genes (ISGs) [Citation50].
Adaptive immunity is characterized as more specific than innate immunity and is involved in establishing immunological memory, yet this response takes some time to establish [Citation51]. Professional antigen-presenting cells (APCs), such as dendritic cells (DCs), link innate and adaptive immunity, activating antigen-specific helper T cells that can help B cells differentiate and produce a particular antibody isotype [Citation52]. These helper T cells can also activate cytotoxic T lymphocytes (CTLs) [Citation49]. Noteworthy, the function and relevance of HIF-α over DC function is not fully understood; however, hypoxic conditions induce the production of numerous proinflammatory cytokines by these cells, such as TNF-α and IL-1β [Citation53], and also modulates chemokine receptor expression [Citation54]. In turn, these cytokines induce the expression of nitric oxide synthase (NOS), and the production of nitric oxide (NO), which may cause PHD inhibition by O2 competition, causing HIF-1α accumulation in normoxic conditions [Citation28]. On the other hand, NO inhibition of active HIF-1 formation in hypoxic conditions may be explained by direct activation of PHDs via the redistribution of intracellular oxygen, given that NO inhibits mitochondrial O2 consumption [Citation28].
In contrast to the effect of HIF-1α over DCs, this transcription factor may have suppressive effects on the production of proinflammatory cytokines by CD4+ and CD8+ T cells, such as IFN-γ. There might be a HIF-1-mediated anti-inflammatory response pathway in T cells, complementing immunosuppressive signaling mediated by extracellular adenosine produced by numerous cells, such as fibroblasts, epithelial cells, and muscle cells in different tissues [Citation55, Citation56]. Noteworthy, hypoxia induces the expression of T cell activation-related receptors, such as 4–1BB and OX40, and inhibitory receptors such as LAG3 and CTLA-4, as well as effector molecules such as granzyme B [Citation35].
Importantly, HIF-1α has been reported to have a vital role over the survival of T cell, the regulation of T and B cells, and the regulation of myeloid cells such as macrophages and neutrophils [Citation57]. Consistently, HIF-1α plays a crucial role in the survival of T cells by preventing activation-induced cell death under hypoxic conditions [Citation35]. This observation makes HIF1-α a key factor in regulating inflammatory processes.
HIF-1 activity has an impact on T cell activation and differentiation. For instance, HIF-1α is highly expressed in Th17-polarized T cells [Citation35], and the deletion of this gene in T cells impairs the differentiation of CD4+ into this T-helper phenotype in vivo [Citation58]. There is also evidence suggesting that HIF-1 negatively regulates Treg development [Citation58], and in CD8+ T cells HIF-1 activation promotes glycolytic metabolism and effector functions, such as granzyme B expression [Citation59].
HIF-1 in viral infections
During viral infections, many studies report that HIF-1α is upregulated in infected cells, suggesting that this factor may have favorable effects for the virus, rather than for the host. Nevertheless, there is also evidence showing that some viruses downregulate the activity of this transcription factor. Altogether, the mechanisms by which viruses modulate HIF-1 seem quite variable and are discussed below in detail for different types of viruses.
Double-stranded DNA viruses
Human papillomavirus
Human papillomaviruses (HPV) are known for producing cervical cancer, although they can also elicit other types of cancer [Citation60]. Overexpression of HIF-1α has been reported to be a marker of poor prognosis in patients with cervical cancer. A study performed with 91 patients showed that individuals with higher levels of HIF-1α in tumor histological samples -obtained surgically- had significantly shorter overall survival rates and disease-free periods than those with lower levels [Citation61]. This observation is consistent with human papillomavirus type 16 (HPV-16) oncoproteins showing an increased capacity to stabilize HIF-1α without necessarily increasing HIF-1α mRNA levels [Citation62]. There is also evidence indicating that these oncoproteins -especially E6 and E7- promote the expression of vascular endothelial growth factor (VEGF) and other angiogenesis factors, such as IL-8, which can promote tumor angiogenesis via a HIF-1α/VEGF pathway in non-small cell lung cancers and human cervical carcinoma cells [Citation63]. Furthermore, there is evidence showing that several HPVs, both low- and high-risk types, can enhance the expression of HIF-1α in human foreskin keratinocytes under hypoxic conditions by stabilizing HIF-1α. However, later studies showed that this increased activation of HIF-1α did not involve the PI3/mTOR, nor the VHL pathway as expected [Citation64]. The information exposed above, suggest that HIF-1 modulation during HPV infection may be beneficial for the control of the disease .
Figure 2. Schematic representation of the effects of double-stranded DNA viruses on HIF-1α and (possible) mechanisms of action
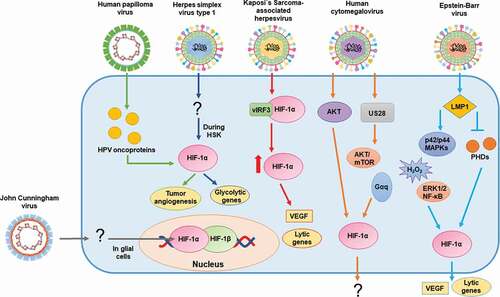
Epstein-Barr virus
Epstein-Barr virus (EBV, HHV-4) is a herpesvirus associated with Hodgkin’s lymphoma, B cell lymphoma, and nasopharyngeal carcinoma [Citation65]. EBV is highly prevalent worldwide, with most infected individuals not showing clinical symptoms [Citation65]. The latent membrane protein 1 (LMP1) of EBV induces the synthesis of the HIF-1α and promotes the expression of HIF-1 response-genes, such as VEGF [Citation66, Citation68]. The mechanism of action underlying this activation in nasopharyngeal epithelial cells requires the up-regulation of Siah1 E3 ubiquitin ligase by LMP1. This up-regulation leads to proteasomal degradation of PHD1 and PHD3. These events promote the stabilization of HIF-1α by preventing the formation of the VHL/HIF-1α complex [Citation66]. Another study suggests that LMP1 increases the expression of HIF-1α through the p42/44 MAPK pathway and H2O2 [Citation68]. This study also indicates that the activity of the promoter of the HIF-1α gene is induced by the latent membrane protein 1 C-terminal activating region 1 recruited extracellular signal-regulated protein kinases 1 and 2/NF-κB pathway (LMP1 CTAR1-recruited ERK1/2/NF-κB pathway) [Citation67]. Mechanistically, there is evidence showing that LMP1 also enhances mRNA levels of HIF-1α via reduced expression of the RNA-destabilizing proteins tristetraprolin (TTP) and pumilio RNA-binding family member 2 (PUM2). These proteins are RNA binding proteins (RBPs) that induce a decay of the HIF-1α mRNA by binding to AU rich elements in mRNAs and repressing their transcription, thus promoting mRNA degradation [Citation67]. Moreover, HIF-1α plays a role in the induction of the lytic cycle of EBV, as hypoxic conditions increase the expression of the immediate-early protein Zta, which mediates the switch between latent and lytic infection, consequently increasing the number of viral DNA copies, as determined in a B-lymphoblastoid cell line [Citation69]. Another study showed that HIF-1α binds to the promoter of the latent-lytic switch BZLF1 gene Zp, activating its transcription and likely inducing EBV lytic-gene expression, suggesting EBV effects over HIF-1 and vice versa [Citation70] .
Kaposi’s sarcoma-associated herpesvirus
Kaposi’s Sarcoma-associated herpesvirus (KSHV, HHV-8) is the causative agent of Kaposi’s Sarcoma cancer, characterized by skin lesions with excessive vascularization of the epithelium [Citation71]. Importantly, infections with KSHV have been reported to upregulate the transcript levels of both HIF-1α and HIF-2α in endothelial cells [Citation72]. Noteworthy, KSHV viral homolog of the host interferon regulatory factor 3(IRF), vIRF3 interacts with HIF-1α to stabilize its protein levels and leads to its transcriptional activation [Citation73]. This interaction also prevents the degradation of HIF-1α under normoxic conditions [Citation73]. Kaposi’s sarcoma is a highly angiogenic endothelial tumor with VEGF playing an important role in the regulation of angiogenesis [Citation74, Citation75]. The interaction between vIRF3 and HIF-1α induces VEGF expression and facilitates endothelial tube growth [Citation73]. Pyruvate kinase-2 acts as a coactivator of HIF-1 and increases the levels of angiogenic factors, such as VEGF [Citation76].
Hypoxia may have a role in the switch between latent to lytic infections of this virus. Interestingly, the KSHV latency-associated nuclear antigen (LANA) has been reported to associate with HIF-1α, enhancing the latter’s transcriptional activity and its mRNA levels. HIF-1α:LANA complex could bind to the HRE of the Rta promoter and upregulate lytic Rta gene expression when KSHV-infected cells are under hypoxic conditions [Citation77]. Hypoxic conditions also induce lytic replication by this virus, via the viral inducer 12-O-tetradecanoylphorbol-13-acetate, that increases the level of IL-6 in HHV-8 infected cells. This increase stimulates spindle cell growth and plays a role in activating the production of angiogenic factors in infected cells [Citation78]. The inhibition of KAP1 (KRAB-associated protein 1) enhances the association of HIF-1α and RBP-Jκ, leading to RTA expression, which has been shown to activate the expression of early and late genes in the KSHV lytic cycle [Citation79]. Another study reported an effect of HIF-1α in KSHV infection as metabolic reprograming of KSHV-infected B cells under hypoxic conditions via vGPCR (G protein-coupled receptor), a lytic cycle-associated- and oncogenic protein that stimulates angiogenesis by increasing the secretion of VEGF [Citation80] .
Human cytomegalovirus
Human cytomegalovirus (HCMV or HHV-5) is also highly prevalent in the human population [Citation81]. Although initially thought to be innocuous, more recent studies have shown multiple pathologies related to this virus, involving a wide range of tissues due to its broad cellular tropism [Citation81]. Human fibroblasts have shown increased HIF-1α expression after infection with HCMV [Citation82]. This effect was also seen when cells were inoculated with UV-inactivated HCMV, indicating that HIF-1α induction occurs due to the interaction of virus structural components with the cell and that viral gene expression is not necessary to induce this response [Citation82]. Consistently, increased phosphorylation of the protein kinase AKT was reported, which is required for the induction of HIF-1α [Citation82]. Interestingly, the HCMV-encoded chemokine receptor US28 increases the stabilization of HIF-1α via a Gq protein alpha subunit- (Gαq), calcium/calmodulin-dependent protein kinase II- (CaMKII) and AKT/mTOR-dependent manner, which supports the notion that HCMV infection may be favored by HIF-1 activation [Citation83] .
Herpes simplex virus type 1
Herpes simplex virus type 1 (HSV-1 or HHV-1) infection is lifelong, like other herpesviruses infections, and recurrent reactivations may occur throughout life. HSV-1 elicits several diseases, both mild and life-threatening. Importantly, HSV-1 can produce herpes stromal keratitis (HSK), which translates into corneal chronic inflammatory conditions and hypoxic conditions [Citation84]. Importantly, HIF-1α stabilization has been observed in infiltrating immune cells during HSK, as well as increased expression of HIF-1 target genes, such as genes related to the glycolytic and lactate pathways in infected corneas [Citation84]. Interestingly, depletion of neutrophils infiltrating the corneas significantly reduced the development of hypoxia in this tissue. This reduction may affect the development of HSK, given that neutrophils are considered essential for the development of this disease [Citation84]. Although an inhibitor of HIF-1α reduced the severity of neovascularization in HSK, the corneas’ opacity was still observed [Citation84]. Thus, the contribution of HIF-1α in HSK is not entirely understood .
John Cunningham virus
The John Cunningham virus (JCV) has been reported to be the etiological cause of progressive multifocal leukoencephalopathy, a demyelinating disease similar to multiple sclerosis, but with a much quicker progressiveness [Citation85]. Glial cells infected with JCV display increased levels of HIF-1α in the nucleus as compared to healthy glial cells. In this study, the presence of HIF-1α promoted the activation of the JCV early and late promoters and was shown to bind to the JCV control region, which may lead to the activation of the JCV promoter [Citation86]. Thus, HIF-1α may be required by JCV for its replication cycle .
Positive-sense single-stranded RNA viruses
Hepatitis C virus
Hepatitis C virus (HCV) is a pathogen capable of causing severe liver diseases, such as cirrhosis or hepatocellular carcinoma in humans. Its infection is considered chronic if untreated, due to the ability of this virus to evade the host’s immune response for extended periods [Citation87]. Importantly, low oxygen concentrations enhance hepatitis C virus replication and induce the upregulation of host genes related to hypoxic stress, such as those involved in glycolytic metabolism, cell growth, and proliferation [Citation88]. Furthermore, HCV stabilizes HIF-1α by activating NF-κB, signal transducer and activator of transcription 3 (STAT-3), PI3-K/AKT, and p42/44 mitogen-activated protein kinase pathways. The induction of HIF-1α leads to the stimulation of VEGF [Citation43]. A study reports that the induction of the HCV core protein leads to stabilization and overexpression of HIF-1α in a cancer cell line and, consequently, to the stimulation of VEGF [Citation89]. HIF-1α stabilization was shown to be insensitive to antioxidant treatments, and mimicking an impairment of mitochondrial oxidative phosphorylation elicited the stabilization of this transcription factor [Citation90]. Therefore, HCV stabilization of HIF-1α by different mechanisms may be contributing to increased HIF-1α upon viral infection with this virus .
Figure 3. Schematic representation of the effects of positive single-stranded RNA viruses on HIF-1α and (possible) mechanisms of action
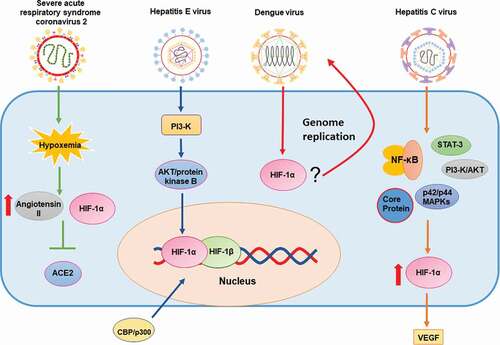
Hepatitis E virus
Hepatitis E virus (HEV) is another etiological agent producing severe liver disease. HEV has an incidence estimated at 20 million new infections every year [Citation91]. Few studies have assessed HEV and HIF-1. One study showed that HEV stabilizes HIF-1α and increases its binding to HRE. This binding increases the transactivation activity of HIF-1 in cells expressing the viral protein ORF3 through the phosphatidylinositol-3-kinase (PI3K)-mediated activation of AKT/protein kinase B. The HIF-1 heterodimer also recruited CBP/p300 to particular gene promoters, in which cases the phosphorylation of CBP/p300 was required for the interaction [Citation92] .
Dengue virus
Dengue virus (DENV) is a Flavivirus transmitted by Aedes mosquitos in tropical and subtropical areas [Citation93]. The infection of this virus leads to flu-like symptoms such as fever, joint pain, and a rash, but in some cases, it can lead to more severe pathologies and reach a mortality rate of up to 20% in some areas [Citation93]. In DENV-infected cells, hypoxia was not shown to have a significant effect on virus entry and viral RNA translation but was shown to enhance DENV genome replication. Interestingly, the hypoxia-mediated enhancement of DENV replication was facilitated by HIF-1α/2α and by the serine/threonine kinase AKT, correlating with increased anaerobic glycolysis. Reactive oxygen species contributed to the hypoxia-mediated increase in DENV replication and to virus-induced hypoxic reprogramming [Citation94] .
Severe acute respiratory syndrome coronavirus 2
Severe acute respiratory syndrome coronavirus 2 (SARS-CoV-2) is a recently discovered virus that causes a severe respiratory disease known as COVID-19, which has extended throughout the world, eliciting a full-blown pandemic [Citation95]. Importantly, SARS-CoV-2 is known to cause hypoxemia due to the loss of hypoxic vasoconstriction, ventilation/perfusion mismatch, and increased coagulopathy [Citation95]. The angiotensin-converting enzyme 2 (ACE2), a transmembrane protein expressed in the respiratory tract, lungs, heart, arteries, veins, kidney, and intestines has been identified as the cellular receptor required for SARS-CoV-2 infection [Citation96,Citation97]. ACE2 protein levels are decreased upon HIF-1α accumulation in human pulmonary artery smooth muscle cells (hPASMCs) [Citation98], as well as in human embryonic kidney cells overexpressing HIF-1α [Citation99]. Importantly, angiotensin II, which is increased under hypoxic conditions, inhibits the synthesis of its ligand ACE2. Moreover, HIF-1α overexpression in hPASMCs -in which angiotensin II was inhibited- did not result in ACE2 downregulation, thus evidencing a direct relationship between HIF-1α and ACE2 expression [Citation98]. Consistent with this notion, recent reports suggest that populations living in high altitudes (3,000 meters above sea level) such as the Tibetan plateau, El Alto in Bolivia and Ecuador, -which are chronically exposed to hypoxic conditions- appear to display decreased pathology upon SARS-CoV-2 infection [Citation100] .
Negative-sense single-stranded RNA viruses
Influenza A virus
Influenza A virus (IAV) is a zoonotic pathogen constantly challenging the public health systems worldwide, as it has a high mutation rate and kills thousands of people around the world each year due to respiratory disease [Citation101] Some of the most common symptoms are fever, severe headache, sore throat, muscle pain, coughing and fatigue [Citation101]. IAV H1N1 infection of human lung adenocarcinoma epithelial cells stabilizes HIF-1α in normoxic conditions but does not increase HIF-1α mRNA transcription. H1N1 infection did not impair post-translational prolyl hydroxylation or ubiquitination of HIF-1α. Interestingly, HIF-1α stabilization in human lung adenocarcinoma epithelial cells infected with IAV seems to be caused by inhibition of the proteasome and decreases in the expression of the factor inhibiting HIF-1 (FIH-1) [Citation102]. Infection with IAV H1N1 resulted in the translocation of HIF-1α to the nucleus during normoxia. Treatment with 2-methoxyestradiol (2ME2) resulted in inhibition of its nuclear accumulation and decreased mRNA and protein expression levels of TNF-α and IL-6, while also increasing levels of IL-10. Under hypoxic conditions, there was an increase in HIF-1α nuclear accumulation, accompanied by increased levels of TNF-α and IL-6, and decreased levels of IL-10 [Citation103]. Therefore, nuclear translocation of HIF-1α induced by IAV H1N1 infection to the nucleus plays an important role in the production of proinflammatory cytokines. Importantly, recent studies show that deficiency of HIF-1α in a human alveolar type-II epithelial cell line (HAE) reduces glycolysis and enhances AMPKα-ULK1-mediated autophagy, therefore facilitating the replication of this virus [Citation104] .
Figure 4. Schematic representation of the effects of negative single-stranded RNA and double-stranded RNA viruses on HIF-1α, and (possible) mechanisms of action
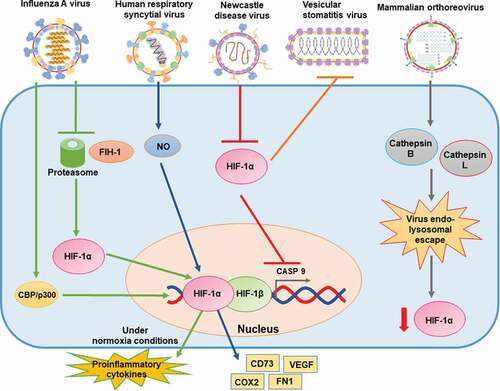
Human respiratory syncytial virus
Human respiratory syncytial virus (hRSV) is a respiratory pathogen that accounts for most acute lower respiratory tract infections among pediatric patients and leads to considerable economic burdens for health systems around the globe [Citation105]. hRSV symptoms vary from rhinorrhea, congestion and low grade fever, to bronchiolitis, pneumonia and sever alveolitis [Citation105]. Pulmonary epithelial cells infected with hRSV display activated HIF-1α. An 8-fold increase in HIF-1α protein expression during hRSV infection is reported in HAE cells, and the expression of HIF-1α target genes, such as VEGF, CD73, FN1, and COX2 was also detected [Citation106]. The activation of HIF-1α was suppressed upon UV-inactivation of the virus, suggesting that viral gene expression is required for these effects. Partial oxygen pressure measurements in the supernatants of infected cells showed no changes against controls, suggesting that activation of HIF-1α is independent of oxygen levels in HAE cells infected with this virus [Citation106]. Noteworthy, NO released from cells infected with hRSV may influence HIF-1α expression, as the addition of an inhibitor of NO blocked the expression of HIF-1α in hRSV-infected human bronchial epithelial cells. This treatment also inhibited VEGF production, which has been previously demonstrated to play a role in changing the permeability of bronchial epithelial monolayers [Citation107] .
Newcastle disease virus
Newcastle disease virus (NDV) is an avian pathogen that elicits high morbidity and mortality in poultry, thus generating high economic burdens to this industry [Citation108]. Depending on the virulence, NDV has been divided into four groups; velogenic viscerotropic (lethal), velogenic nerotropic (high mortality), mesogenic (low mortality) and lentogenic (asymptomatic), each one is characterized by different symptoms [Citation109]. The infection of several cell lines with NDV inhibits HIF-1α protein accumulation and, consequently, decreases the transcription of the HIF-1α target genes, particularly the carbonic anhydrase 9 gene, which encodes the CAIX protein [Citation110]. This protein plays a critical role in primary cancer development, progression, and metastatic disease [Citation111]. Accumulation of HIF-1α was due to post-translational events and decreased upon inhibition of the proteasome, implicating that NDV likely downregulates HIF-1α through the proteasomal pathway. Interestingly, this pathway appeared to be independent of the p53 and VHL host proteins, implicated in regulating the stabilization of HIF-1α [Citation110] .
Vesicular stomatitis virus
Vesicular stomatitis virus (VSV) is a zoonotic pathogen that affects a wide range of species, from arthropods to humans [Citation112]. Humans’ infection leads to flu-like symptoms without severe illness; however, this virus causes considerable economic losses when infecting cattle, in which symptoms include vesicular lesions of the gums, tongue, naso-oral mucosa, teats and coronary bands [Citation112]. Interestingly, renal carcinoma cells devoid of VHL, which is known to play an important role in the regulation of HIF-1α, conferred cells enhanced resistance to VSV-mediated cytotoxicity. Consistently, hypoxic conditions also promoted resistance to the infection by VSV, as determined by the assessment of live cells. This effect may be explained by HIF-1α-mediated induction of genes with antiviral effects, such as IFN-β. In contrast, the inhibition of HIF-1α enhanced the infection of cells with this virus [Citation113] .
Double stranded RNA viruses
Mammalian orthoreovirus
Mammalian orthoreovirus (MRV, reovirus) is a highly prevalent benign human virus. Infections with this pathogen usually cause subclinical manifestations and are unnoticed [Citation114]. Three reovirus serotypes are known: type 1 Lang (T1L), type 2 Jones (T2J), and serotype 3 which is divided into the two prototypes type 3 Abeney (T3A) and type 3 Dearing (T3D) [Citation115]. Infection of reovirus-permissive tumor cells and reovirus-resistant tumor cells with reovirus has been shown to significantly downregulate HIF-1α protein levels, which also occurred with UV-inactivated virus, indicating that downregulation of HIF-1α was independent of virus replication [Citation115]. Furthermore, transfection of reovirus genome into human tumor cell lines also downregulated HIF-1α protein levels, even in the presence of polyinosinic-polycytidylic acid (polyI:C), which is a synthetic double-stranded RNA analogue that acts as a pathogen-associated molecular pattern (PAMP), indicating that reovirus RNA plays an important role in this reduction [Citation115]. Interestingly, this effect was inhibited when cells were pretreated with inhibitors of cathepsins B and L, suggesting that endo-lysosomal escape of the reovirus genome into the cytoplasm is crucial for HIF-1α downregulation [Citation115]. Another study also showed that reovirus infection downregulates the expression of HIF-1α-target genes in subcutaneous tumors. However, UV-inactivated virus did not downregulate HIF-1α protein levels in these cells [Citation116] .
Viruses that replicate through DNA intermediates
Hepatitis B virus
Hepatitis B virus (HBV) also causes severe hepatic illness in humans, which is chronic and is estimated to affect approximately 250 million people worldwide [Citation117]. The hepatitis B virus X protein (HBx) is involved in hepatocellular carcinoma [Citation118], and likely in the regulation of viral genes by acting on viral promoters [Citation119]. Studies focusing on the HBx protein have reported increases both in the transcriptional activity and protein levels of HIF-1α in normoxic and hypoxic conditions [Citation118]. This increases also stimulated angiogenesis. Interestingly, HBx inhibited pVHL binding to HIF-1α, preventing the degradation of this transcription factor [Citation118]. Stabilization of HIF-1α by HBx induced the nuclear translocation of C/EBPβ and caused induction of the activity of the transporter of multidrug resistance 1 gene (MDR1) [Citation120] .
Figure 5. Schematic representation of the effects of retrotranscribing viruses on HIF-1α and (possible) mechanisms of action
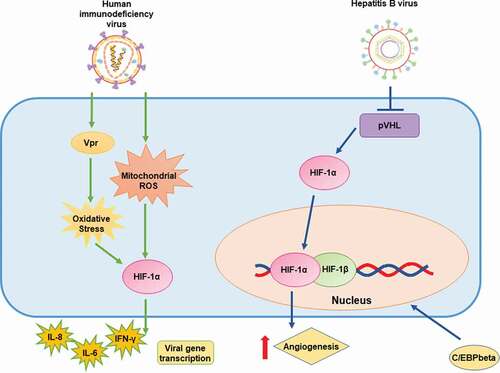
Human immunodeficiency virus
Human immunodeficiency virus (HIV) causes acquired immunodeficiency syndrome (AIDS) by inducing cell death of T CD4+ lymphocytes [Citation121]. The viral protein Vpr promotes HIF-1α expression by activating cellular oxidative stress, which induces HIF-1α protein accumulation and, consequently, stimulates viral gene transcription [Citation122, Citation123]. Importantly, the HIV promoter has been reported to be positively modulated not only by Vpr but also by HIF-1α through the GC-rich binding domain in the long terminal repeat (LTR) [Citation122]. There is also evidence suggesting that cytosolic double-stranded DNA generated during the viral replication cycle of HIV in CD4+ T cells induces mitochondrial ROS-dependent HIF-1α stabilization, which enhances viral replication. Stabilization of HIF-1α promoted the release of extracellular vesicles, which induced the secretion of IFN-γ by bystander CD4+ T cells, and the secretion of IL-6 and IL-1β by bystander macrophages [Citation124]. These results suggest that the stabilization of HIF-1α in the context of HIV infection triggers the secretion of inflammatory cytokines .
Taken together, while some of the viruses described above downregulate HIF-1 expression and the expression of HIF-1-targeted genes, other viruses activate this factor. Nevertheless, there are some similarities that exist between different viruses in how they affect HIF-1. These aspects are summarized in .
Figure 6. Common features between different viruses and HIF-1α. A
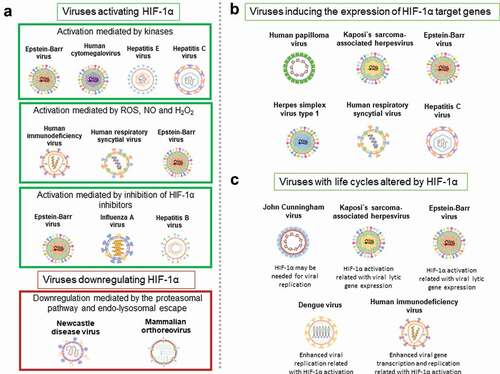
HIF-1α as a therapeutic target
HIF-1α is involved in numerous viral infection processes, and hypoxia signaling has been described in a variety of diseases, such as heart conditions (i.e., ischemic heart disease), lung and kidney injuries (i.e., acute lung injury and acute kidney injury), liver steatohepatitis, cancer, and infectious diseases [Citation29, Citation125, Citation126].
Therefore, this transcription factor has been considered as a potential target for the development of new therapies [Citation125, Citation126]. However, it is essential to note that HIF-1 protects the organism during hypoxic conditions, and thus, its inhibition may have detrimental effects for the host [Citation126]. HIF-1 is induced in normoxic conditions in several infectious and non-infectious situations and contributes to the pathophysiology of numerous diseases. Therefore either, the activation or inhibition of HIF-1α may contribute to more favorable outcomes depending on the particular disease.
In the context of organ protection, there are several studies in which HIF-1α was targeted for activation in different tissues with favorable results (e.g., heart, lung, liver, and kidney) [Citation127]. For instance, HIF-1 activity has been shown to be induced after tissue hypoxia due to reduced tissue perfusion, with the consequent transcription of genes encoding for angiogenic factors such as EPO, ANGPT1 and VEGF [Citation128]. These factors play important roles in ischemic heart diseases because they stimulate the remodeling of collateral blood vessels, which leads to increased blood flow in this tissue [Citation128] and play an important role in cardiac adaptation during cardiac hypertrophy [Citation129]. Reportedly, a common method used in clinical trials for stabilizing HIF-1α is ischemic preconditioning/ischemic postconditioning (IPC/IPostC) [Citation126]. There is evidence that HIF-1 is capable of mediating cardioprotection induced by ischemic preconditioning, and by coordinating a balance between glycolytic and oxidative metabolisms [Citation130]. Furthermore, HIF-1 activity has been reported to be important at maintaining O2 homeostasis during compensatory myocardial hypertrophy in response to pressure overload, and to play an important role in protecting against pressure overload after heart failure [Citation129, Citation128]. Consistently, knockdown of endothelial HIF-1α in the heart increased TGF-β signaling, which led to pathological remodeling [Citation131]. However, it is noteworthy that, in some cases contrary effects have been described after HIF-1 overexpression. For example, a study reported that increasing the expression of cardiac HIF-1α resulted in aggravated heart failure [Citation132].
Another strategy considers the use of inhibitors of PHDs, which lead to the activation of HIF-1α. To date, non-specific PHDs inhibitors, such as vadadustat, roxadustat, daprodustat, and molidustat are being tested or have been recently evaluated in clinical trials for the treatment of patients with chronic kidney disease (CKD) [Citation126, Citation133]. This illness increases systemic oxidative stress and inflammation, activates the renin-angiotensin system, and causes nutritional disorders, which altogether may increase the risk of cardiovascular events [Citation134, Citation135]. Anemia caused by a reduction in renal erythropoietin (EPO), as a consequence of CKD may also affect cardiac function [Citation135]. Interestingly, the gene encoding EPO is a target of HIF-1, although primarily HIF-2 [Citation136]. Direct administration of molecules that are induced by HIF-1, such as EPO, are also being evaluated for their ability to protect the heart and kidneys from acute injuries, as well as for treating anemia in CKD [Citation126, Citation133].
Cancer is another disease that has been reported to have a connection with HIF-1. Immunohistochemical analyses of several types of cancer show an overexpression of HIF-1α [Citation137]. Interestingly, this effect is caused by gain of functional mutations in oncogenes, loss of functional mutations in tumor suppressor genes, as well as a result of intratumor hypoxia [Citation138, Citation139, Citation140, Citation137]. Although the overexpression of HIF-1 in head and neck cancers and non-small-cell lung cancer was reported to reduce the mortality of the patients, other studies have failed to reproduce these effects [Citation141, Citation142, Citation143, Citation144, Citation137]. Indeed, other reports have associated HIF-1α expression with increased mortality for several cancers, such as cervical, breast, endometrial, oropharyngeal and ovarian cancer [Citation145, Citation146, Citation147, Citation148, Citation149, Citation137].
Currently, several studies exploring the potential of HIF-1α inhibition for treating cancer are ongoing, as HIF-1α activity increases solid tumor survival, as well as tumor aggressiveness, invasion, and metastasis [Citation150, 152]. In this regard, HIF-1α inhibitors are being assessed both in pre-clinical and clinical trials with two major types of HIF-1 inhibitors: direct or indirect inhibitors, which modulate factors that are up- or downstream of HIF-1-related signaling pathways [Citation125]. Importantly, HIF-1α and hypoxic conditions affect the function of immunosuppressive myeloid-derived suppressor cells (MDSCs) in tumors, and thus the modulation of this factor may have direct effects over these cells [Citation153]. Other studies have reported that HIF-1 plays a role in tumor resistance to chemo- and radiotherapy [Citation125]. Interestingly, a combination of inhibitors of HIF-1α and chemotherapy showed a higher anti-tumor effect than the chemotherapy agent gemcitabine alone [Citation154]. A combination of radiotherapy and HIF-1α inhibitors showed similar results, increasing the tumor’s sensitivity to radiation [Citation155]. The role of HIF-1α in other ailments and infectious diseases (e.g. bacterial) has been extensively reviewed by Semenza and dos Santos [Citation29, Citation23].
Alternatively, targeting HIF-1 may also help treat pathogenesis associated with inflammation, as HIF-1α regulates essential pathways for maintaining energy homeostasis in myeloid cells [Citation156]. Additionally, inactivation of HIF-1α has been reported to inhibit the motility, invasiveness, and homotypic adhesion of isolated peritoneal macrophages [Citation156]. Given that inhibition of glycolysis prevents inflammatory responses, and glycolytic metabolism-related genes are targeted by HIF-1α activation, the inhibition of this transcription factor may help inhibit inflammatory responses [Citation157]. Therefore, as inflammation is a component or a symptom related to the severity of numerous infectious diseases, inhibiting HIF-1 may have potential therapeutic effects against microbial infections [Citation157].
For instance, this could be the case for the Kaposi’s sarcoma-associated herpesvirus, Epstein-Barr virus, and human respiratory syncytial virus, in which cases the expression of HIF-1 target genes has been reported to have positive effects regarding the pathogenicity of viral infections [Citation77, Citation69, Citation107, Citation76]. Particularly, VEGF has been shown to play essential roles in KSHV and hRSV infections, so inhibition of HIF-1 and, therefore VEGF inhibition, may help overcome either infection or even decrease disease severity.
HIF-1α expression can be suppressed with siRNAs or specific molecules. Interestingly, several studies have used siRNAs to understand the effect of HIF-1 in different contexts [Citation158, Citation159]. For example, inhibition of HIF-1α with a siRNA promoted a metabolic shift in human pluripotent stem cell-derived cardiomyocytes [Citation158], and in podocytes where the inhibition of this transcription factor decreased the expression of inflammatory cytokines [Citation159]. On the other hand, numerous molecules that inhibit HIF-1α expression have been discovered or synthetized as a therapy for cancer. There are different mechanisms by which these molecules inhibit this transcription factor, such as through the inhibition of mRNA expression (e.g. by using EZN-2968 or EZN-2208), inhibition of protein synthesis (e.g. by using 2-methoxyestradiol or CAY10585), or by promoting its degradation (e.g. by using 17-DMAG or bisphenol A) [Citation160], among others.
Some molecules such as vadadustat, roxadustat, daprodustat, and molidustat, which are PHDs inhibitors, have opposite effects compared to the molecules described above and activate HIF-1α. These molecules may be used for developing antiviral treatments for viruses in which HIF-1 activity may be detrimental, such as VSV, as cells that do not express the von Hippel-Lindau protein are more resistant to VSV-mediated cytotoxicity [Citation113]. Also, this type of drug may be used for treating NDV, because infection with this virus downregulates HIF-1α stabilization, and therefore the activation of this transcription factor may have an antiviral effect [Citation110]. Finally, these types of drugs may be used against SARS-CoV-2, as ACE2 which is crucial for viral infection, decreases upon HIF-1α accumulation [Citation98].
Till date, there are no drugs capable of modulating HIF-1 that are being tested in clinical trials for the control of viral infections. Nevertheless, we foresee this to happen in the short term. Inhibition of HIF-1 should be approached with caution in microbial infections, as studies report uncertain outcomes for infectious diseases when stimulating HIF-1. For example, Okumura et al. reported that stabilizing HIF-1α with AKB-4924 -an inhibitor of PHDs- resulted in a positive effect on the innate immune response by enhancing the antibacterial activity of macrophages and keratinocytes against methicillin-sensitive and methicillin-resistant Staphylococcus aureus. The same drug stimulated the bacteria-killing capacity of keratinocytes against Pseudomonas [Citation161].
Concluding remarks
Numerous studies carried out till date support that HIF-1 plays relevant roles in the infectious cycles of numerous viruses, as well as the host response to these pathogens. Noteworthy, most viruses studied to date have been shown to positively modulate this pathway by upregulating the stabilization of HIF-1α as a consequence of infection. Importantly, HIF-1 also plays an important role in the modulation of both, the innate and the adaptive immune responses of the host against viral infection.
Although the relationship between HIF-1-related pathways and the modulation of the life-cycles of some viruses have been dilucidated, the precise molecular associations occurring with several other viruses and this host factor remain to be determined. However, some common features exist among different types of viruses .
Despite the involvement of HIF-1 in the infection of diverse viruses, there are reportedly no ongoing clinical trials evaluating the potential therapeutic effects of the pharmacological modulation of HIF-1 in viral infections. However, given the increasing amount of evidence regarding the participation of HIF-1 in viral infections, we foresee that additional studies will originate in the short term, assessing the contribution of this host factor over the replication of viruses and viral diseases, as well as the mechanisms of action by which HIF-1 and its related pathways influence viral infection and disease outcomes.
Acknowledgments
Authors of this work are supported by the Millennium Institute on Immunology and Immunotherapy (P09/016-F; ICN09_016) and FONDECYT grants #1190864, #1190830, #1170964 from the Agencia Nacional de Investigación y Desarrollo (ANID) and CONICYT scholarship 21190183.
Disclosure statement
The authors declare no conflict of interest.
Additional information
Funding
References
- Semenza GL, Wang GL. A nuclear factor induced by hypoxia via de novo protein synthesis binds to the human erythropoietin gene enhancer at a site required for transcriptional activation. Mol Cell Biol. 1992;12(12):5447–5454.
- Ponting CP, Aravind L. PAS: a multifunctional domain family comes to light. Curr biol: CB. 1997;7(11):R674-R677.
- Weidemann A, Johnson RS. Biology of HIF-1α. Cell Death Differ. 2008;15(4):621–627.
- Ke Q, Costa M. Hypoxia-inducible factor-1 (HIF-1). Mol Pharmacol. 2006;70(5):1469–1480.
- Loboda A, Jozkowicz A, Dulak J. HIF-1 and HIF-2 transcription factors–similar but not identical. Mol Cells. 2010. DOI:https://doi.org/10.1007/s10059-010-0067-2
- Yang SL, Wu C, Xiong ZF, et al. Progress on hypoxia-inducible factor-3: its structure, gene regulation and biological function (Review). Mol Med Rep. 2015. DOI:https://doi.org/10.3892/mmr.2015.3689
- Dales JP, Beaufils N, Silvy M, et al. Hypoxia inducible factor 1α gene (HIF-1α) splice variants: potential prognostic biomarkers in breast cancer. BMC Med. 2010;8(1). DOI:https://doi.org/10.1186/1741-7015-8-44
- Tolonen JP, Heikkilä M, Malinen M, et al. A long hypoxia-inducible factor 3 isoform 2 is a transcription activator that regulates erythropoietin. Cell Mol Life Sci. 2019. DOI:https://doi.org/10.1007/s00018-019-03387-9
- Lando D. Asparagine hydroxylation of the HIF transactivation domain: a hypoxic switch. Science. 2002;295(5556):858–861.
- Ng KM, Lee YK, Chan YC, et al. Exogenous expression of HIF-1α promotes cardiac differentiation of embryonic stem cells. J Mol Cell Cardiol. 2010;48(6):1129–1137.
- Ryan HE. HIF-1alpha is required for solid tumor formation and embryonic vascularization. Embo J. 1998;17(11):3005–3015.
- Jiang BH, Agani F, Passaniti A, et al. V-SRC induces expression of hypoxia-inducible factor 1 (HIF-1) and transcription of genes encoding vascular endothelial growth factor and enolase 1: involvement of HIF-1 in tumor progression. Cancer Res. 1997;57(23):5328–5335.
- Zhong H, Agani F, Baccala AA, et al. Increased expression of hypoxia inducible factor-1alpha in rat and human prostate cancer. Cancer Res. 1998;58(23):5280–5284.
- Chen D, Li M, Luo J, et al. Direct interactions between HIF-1α and Mdm2 modulate p53 function. J Biol Chem. 2003. DOI:https://doi.org/10.1074/jbc.C200694200
- Greijer AE. The role of hypoxia inducible factor 1 (HIF-1) in hypoxia induced apoptosis. J Clin Pathol. 2004;57(10):1009–1014.
- Kothari S, Cizeau J, McMillan-Ward E, et al. BNIP3 plays a role in hypoxic cell death in human epithelial cells that is inhibited by growth factors EGF and IGF. Oncogene. 2003;22(30):4734–4744.
- Forsythe JA, Jiang BH, Iyer NV, et al. Activation of vascular endothelial growth factor gene transcription by hypoxia-inducible factor 1. Mol Cell Biol. 1996;16(9):4604–4613.
- Melillo G, Musso T, Sica A, et al. A hypoxia-responsive element mediates a novel pathway of activation of the inducible nitric oxide synthase promoter. J Exp Med. 1995;182(6):1683–1693.
- Pugh CW, Ratcliffe PJ. Regulation of angiogenesis by hypoxia: role of the HIF system. Nat Med. 2003. DOI:https://doi.org/10.1038/nm0603-677
- Takahashi Y, Takahashi S, Shiga Y, et al. Hypoxic induction of prolyl 4-hydroxylase α(I) in cultured cells. J Biol Chem. 2000;275(19):14139–14146.
- Nalbandian M, Takeda M. Lactate as a signaling molecule that regulates exercise-induced adaptations. Biology (Basel). 2016. DOI:https://doi.org/10.3390/biology5040038
- Wenger RH. Cellular adaptation to hypoxia: O2-sensing protein hydroxylases, hypoxia-inducible transcription factors, and O2-regulated gene expression. Faseb J. 2002;16(10):1151–1162.
- Semenza GL. Hif-1 and human disease: one highly involved factor. Genes Dev. 2000. DOI:https://doi.org/10.1101/gad.14.16.1983
- Werth N, Beerlage C, Rosenberger C, et al. Activation of hypoxia inducible factor 1 is a general phenomenon in infections with human pathogens. PLoS ONE. 2010;5(7):e11576.
- Morinet F, Parent M, Pillet S, et al. Hypoxia inducible factor one alpha and human viral pathogens. Curr Res Transl Med. 2017;65(1):7–9.
- Morinet F, Parent M, Bergeron C, et al. Oxygen and viruses: a breathing story. J Gen Virol. 2015;96(8):1979–1982.
- Morinet F, Casetti L, François JH, et al. Oxygen tension level and human viral infections. Virology. 2013;444(1–2):31–36.
- Hellwig-Bürgel T, Stiehl DP, Wagner AE, et al. Review: hypoxia-inducible factor-1 (HIF-1): a novel transcription factor in immune reactions. J Interferon Cytokine Res. 2005;25(6):297–310.
- Dos Santos SA, de Andrade DR Júnior. HIF–1alpha and infectious diseases: A new frontier for the development of new therapies. Rev Inst Med Trop Sao Paulo. 2017. DOI:https://doi.org/10.1590/S1678-9946201759092
- Kallio PJ, Pongratz I, Gradin K, et al. Activation of hypoxia-inducible factor 1 : posttranscriptional regulation and conformational change by recruitment of the Arnt transcription factor. Proc Natl Acad Sci U S A. 1997;94(11):5667–5672.
- Masson N, Willam C, Maxwell PH, et al. Independent function of two destruction domains in hypoxia-inducible factor-α chains activated by prolyl hydroxylation. Embo J. 2001;20(18):5197–5206.
- Hirota K, Semenza GL. Regulation of hypoxia-inducible factor 1 by prolyl and asparaginyl hydroxylases. Biochem Biophys Res Commun. 2005;338(1):610–616.
- Ivan M, Kondo K, Yang H, et al. HIFα targeted for VHL-mediated destruction by proline hydroxylation: implications for O2 sensing. Science. 2001;292(5516):464–468.
- Huang LE, Arany Z, Livingston DM, et al. Activation of hypoxia-inducible transcription factor depends primarily upon redox-sensitive stabilization of its α subunit. J Biol Chem. 1996;271(50):32253–32259.
- Palazon A, Goldrath AW, Nizet V, et al. HIF transcription factors, inflammation, and immunity. Immunity. 2014;41(4):518–528.
- Biron CA. Innate immunity: recognizing and responding to foreign invaders-no training needed. Viral Pathogenesis: Basics Syst Biol: Third Ed. 2016. DOI:https://doi.org/10.1016/B978-0-12-800964-2.00004-5
- Medzhitov R, Janeway CA. Innate immunity: the virtues of a nonclonal system of recognition. Cell. 1997;91(3):295–298.
- Kao RL, Browder W, Li C. Cellular cardiomyoplasty: what have we learned? Asian Cardiovasc Thorac Ann. 2009;17(1):89–101.
- Wang GL, Jiang BH, Semenza GL. Effect of protein kinase and phosphatase inhibitors on expression of hypoxia inducible factor 1. Biochem Biophys Res Commun. 1995;216(2):669–675.
- Zhang M, Wu Y, Wang M, et al. Genistein rescues hypoxia-induced pulmonary arterial hypertension through estrogen receptor and β-adrenoceptor signaling. J Nutr Biochem. 2018;58:110–118.
- Banerjee S, Li Y, Wang Z, et al. Multi-targeted therapy of cancer by genistein. Cancer Lett. 2008;269(2):226–242.
- Li S, Li J, Dai W, et al. Genistein suppresses aerobic glycolysis and induces hepatocellular carcinoma cell death. Br J Cancer. 2017;117(10):1518–1528.
- Nasimuzzaman M, Waris G, Mikolon D, et al. Hepatitis C virus stabilizes hypoxia-inducible factor 1α and stimulates the synthesis of vascular endothelial growth factor. J Virol. 2007;81(19):10249–10257.
- Chan DA, Sutphin PD, Denko NC, et al. Role of prolyl hydroxylation in oncogenically stabilized hypoxia-inducible factor-1α. J Biol Chem. 2002;277(42):40112–40117.
- Sodhi A, Montaner S, Miyazaki H, et al. MAPK and akt Act cooperatively but independently on hypoxia inducible factor-1α in rasV12 upregulation of VEGF. Biochem Biophys Res Commun. 2001;287(1):292–300.
- Zhong H, Chiles K, Feldser D, et al. Modulation of hypoxia-inducible factor 1α expression by the epidermal growth factor/phosphatidylinositol 3-kinase/PTEN/AKT/FRAP pathway in human prostate cancer cells: implications for tumor angiogenesis and therapeutics. Cancer Res. 2000;60(6):1541–1545.
- Cummins EP, Berra E, Comerford KM, et al. Prolyl hydroxylase-1 negatively regulates IκB kinase-β, giving insight into hypoxia-induced NFκB activity. Proc Natl Acad Sci U S A. 2006;103(48):18154–18159.
- Walmsley SR, Print C, Farahi N, et al. Hypoxia-induced neutrophil survival is mediated by HIF-1α-dependent NF-κB activity. J Exp Med. 2005;201(1):105–115.
- Abbas AK, L AH, P S. Cellular and molecular immunology, ninth edition. Cell Mol Immunol. 2018;Chapter 4, 57-95.
- Naldini A, Carraro F, Fleischmann WR, et al. Hypoxia Enhances the Antiviral Activity of Interferons. J Interferon Res. 1993;13(2):127–132.
- Netea MG, Schlitzer A, Placek K, et al. Innate and adaptive immune memory: an evolutionary continuum in the host’s response to pathogens. Cell Host Microbe. 2019;25(1):13–26.
- Ito T, Connett JM, Kunkel SL, et al. The linkage of innate and adaptive immune response during granulomatous development. Front Immunol. 2013;4. DOI:https://doi.org/10.3389/fimmu.2013.00010.
- Mancino A, Schioppa T, Larghi P, et al. Divergent effects of hypoxia on dendritic cell functions. Blood. 2008;112(9):3723–3734.
- Ricciardi A, Elia AR, Cappello P, et al. Transcriptome of hypoxic immature dendritic cells: modulation of chemokine/receptor expression. Mol Cancer Res. 2008;6(2):175–185.
- Lappas CM, Rieger JM, Linden J. A 2A adenosine receptor induction inhibits IFN-γ production in murine CD4 + T cells. J Immunol. 2005;174(2):1073–1080.
- Lukashev D, Klebanov B, Kojima H, et al. Cutting edge: hypoxia-inducible factor 1α and Its activation-inducible short isoform i.1 negatively regulate functions of CD4+ and CD8+ T lymphocytes. J Immunol. 2006;177(8):4962–4965.
- Sitkovsky M, Lukashev D. Regulation of immune cells by local-tissue oxygen tension: HIF1α and adenosine receptors. Nat Rev Immunol. 2005;5(9):712–721.
- Dang EV, Barbi J, Yang HY, et al. Control of TH17/Treg balance by hypoxia-inducible factor 1. Cell. 2011;146(5):772–784.
- Doedens AL, Phan AT, Stradner MH, et al. Hypoxia-inducible factors enhance the effector responses of CD8+ T cells to persistent antigen. Nat Immunol. 2013;14(11):1173–1182.
- Yang A, Farmer E, Wu TC, et al. Perspectives for therapeutic HPV vaccine development. J Biomed Sci. 2016;23(1). DOI:https://doi.org/10.1186/s12929-016-0293-9
- Birner P, Schindl M, Obermair A, et al. Overexpression of hypoxia-inducible factor 1alpha is a marker for an unfavorable prognosis in early-stage invasive cervical cancer. Cancer Res. 2000b;60(17):4693–4696.
- Li G, He L, Zhang E, et al. Overexpression of human papillomavirus (HPV) type 16 oncoproteins promotes angiogenesis via enhancing HIF-1α and VEGF expression in non-small cell lung cancer cells. Cancer Lett. 2011;311(2):160–170.
- Tang X, Zhang Q, Nishitani J, et al. Overexpression of human papillomavirus type 16 oncoproteins enhances hypoxia-inducible factor 1 protein accumulation and vascular endothelial growth factor expression in human cervical carcinoma cells. Clin Cancer Res. 2007;13(9):2568–2576.
- Nakamura M, Bodily JM, Beglin M, et al. Hypoxia-specific stabilization of HIF-1alpha by human papillomaviruses. Virology. 2009;387(2):442–448.
- Cohen JI. Optimal treatment for chronic active Epstein-Barr virus disease: editorial. Pediatr Transplant. 2009;13(4):393–396.
- Kondo S, Seo SY, Yoshizaki T, et al. EBV latent membrane protein 1 up-regulates hypoxia-inducible factor 1α through Siah1-mediated down-regulation of prolyl hydroxylases 1 and 3 in nasopharyngeal epithelial cells. Cancer Res. 2006. DOI:https://doi.org/10.1158/0008-5472.CAN-06-1679
- Sung -W-W, Chu Y-C, Chen P-R, et al. Positive regulation of HIF-1A expression by EBV oncoprotein LMP1 in nasopharyngeal carcinoma cells. Cancer Lett. 2016;382(1):21–31.
- Wakisaka N, Kondo S, Yoshizaki T, et al. Epstein-Barr Virus latent membrane protein 1 induces synthesis of hypoxia-inducible factor 1α. Mol Cell Biol. 2004;24(12):5223–5234.
- Jiang J-H, Wang N, Li A, et al. Hypoxia can contribute to the induction of the Epstein-Barr virus (EBV) lytic cycle. J Clin Virol. 2006;37(2):98–103.
- Kraus RJ, Yu X, Cordes B, et al. Hypoxia-inducible factor-1α plays roles in Epstein-Barr virus’s natural life cycle and tumorigenesis by inducing lytic infection through direct binding to the immediate-early BZLF1 gene promoter. PLoS Pathog. 2017;13(6):e1006404.
- Mesri EA, Cesarman E, Boshoff C. Kaposi’s sarcoma and its associated herpesvirus. Nat Rev Cancer. 2010;10(10):707–719.
- Carroll PA, Kenerson HL, Yeung RS, et al. Latent kaposi’s sarcoma-associated Herpesvirus infection of endothelial cells activates hypoxia-induced factors. J Virol. 2006;80(21):10802–10812.
- Shin YC, Joo CH, Gack MU, et al. Kaposi’s sarcoma-associated herpesvirus viral IFN regulatory factor 3 stabilizes hypoxia-inducible factor-1α to induce vascular endothelial growth factor expression. Cancer Res. 2008;68(6):1751–1759.
- DiMaio TA, Lagunoff M. KSHV induction of angiogenic and lymphangiogenic phenotypes. Front Microbiol. 2012;3. DOI:https://doi.org/10.3389/fmicb.2012.00102.
- Purushothaman P, Uppal T, Sarkar R, et al. KSHV-mediated angiogenesis in tumor progression. Viruses. 2016;8(7):198.
- Ma T, Patel H, Babapoor-Farrokhran S, et al. KSHV induces aerobic glycolysis and angiogenesis through HIF-1-dependent upregulation of pyruvate kinase 2 in Kaposi’s sarcoma. Angiogenesis. 2015;18(4):477–488.
- Cai Q, Lan K, Verma SC, et al. Kaposi’s sarcoma-associated Herpesvirus latent protein LANA interacts with HIF-1α to upregulate RTA expression during hypoxia: latency control under low oxygen conditions. J Virol. 2006;80(16):7965–7975.
- Davis DA, Rinderknecht AS, Paul Zoeteweij J, et al. Hypoxia induces lytic replication of Kaposi sarcoma-associated herpesvirus. Blood. 2001;97(10):3244–3250.
- Zhang L, Zhu C, Guo Y, et al. Inhibition of KAP1 enhances hypoxia-induced kaposi’s sarcoma-associated Herpesvirus reactivation through RBP-J. J Virol. 2014. DOI:https://doi.org/10.1128/jvi.00283-14
- Singh RK, Lang F, Pei Y, et al. Metabolic reprogramming of Kaposi’s sarcoma associated herpes virus infected B-cells in hypoxia. PLoS Pathog. 2018;14(5):e1007062.
- Griffiths P, Baraniak I, Reeves M. The pathogenesis of human cytomegalovirus. J Pathol. 2015;235(2):288–297.
- McFarlane S, Nicholl MJ, Sutherland JS, et al. Interaction of the human cytomegalovirus particle with the host cell induces hypoxia-inducible factor 1 alpha. Virology. 2011;414(1):83–90.
- de Wit RH, Mujic-Delic A, van Senten JR, et al. Human cytomegalovirus encoded chemokine receptor US28 activates the HIF-1α/PKM2 axis in glioblastoma cells. Oncotarget. 2016;7(42):67966–67985.
- Rao P, Suvas S. Development of inflammatory hypoxia and prevalence of glycolytic metabolism in progressing herpes stromal keratitis lesions. J Immunol. 2019;202(2):514–526.
- Pietropaolo V, Prezioso C, Bagnato F, et al. John Cunningham virus: an overview on biology and disease of the etiological agent of the progressive multifocal leukoencephalopathy. New Microbiol. 2018;41(3):179–186.
- Piña-Oviedo S, Khalili K, Del Valle L. Hypoxia inducible factor-1 alpha activation of the JCV promoter: role in the pathogenesis of Progressive Multifocal Leukoencephalopathy. Acta Neuropathol. 2009;118(2):235–247.
- Chan ST, Ou JHJ. Hepatitis C virus-induced autophagy and host innate immune response. Viruses. 2017. DOI:https://doi.org/10.3390/v9080224
- Vassilaki N, Kalliampakou KI, Kotta-Loizou I, et al. Low oxygen tension enhances hepatitis C virus replication. J Virol. 2013;87(5):2935–2948.
- Zhu C, Liu X, Wang S, et al. Hepatitis C virus core protein induces hypoxia-inducible factor 1α-mediated vascular endothelial growth factor expression in Huh7.5.1 cells. Mol Med Rep. 2014. DOI:https://doi.org/10.3892/mmr.2014.2039
- Ripoli M, D’Aprile A, Quarato G, et al. Hepatitis C virus-linked mitochondrial dysfunction promotes hypoxia-inducible factor 1α-mediated glycolytic adaptation. J Virol. 2010;84(1):647–660.
- Nimgaonkar I, Ding Q, Schwartz RE, et al. Hepatitis e virus: advances and challenges. Nat Rev Gastroenterol Hepatol. 2018. DOI:https://doi.org/10.1038/nrgastro.2017.150
- Moin SM, Chandra V, Arya R, et al. The hepatitis E virus ORF3 protein stabilizes HIF-1α and enhances HIF-1-mediated transcriptional activity through p300/CBP. Cell Microbiol. 2009;11(9):1409–1421.
- Uno N, Ross TM. Dengue virus and the host innate immune response. Emerging Microbes Infect. 2018;7(1):1–11.
- Frakolaki E, Kaimou P, Moraiti M, et al. The role of tissue oxygen tension in Dengue Virus replication. Cells. 2018;7(12):241.
- Afsar B, Kanbay M, Afsar RE. Hypoxia inducible factor-1 protects against COVID-19: a hypothesis. Med Hypotheses. 2020;143:109857.
- Hamming I, Timens W, Bulthuis MLC, et al. Tissue distribution of ACE2 protein, the functional receptor for SARS coronavirus. A first step in understanding SARS pathogenesis. J Pathol. 2004;203(2):631–637.
- Lu R, Zhao X, Li J, et al. Genomic characterisation and epidemiology of 2019 novel coronavirus: implications for virus origins and receptor binding. Lancet. 2020;395(10224):565–574.
- Zhang R, Wu Y, Zhao M, et al. Role of HIF-1α in the regulation ACE and ACE2 expression in hypoxic human pulmonary artery smooth muscle cells. Am J Physiol Lung Cell Mol Physiol. 2009;297(4):L631-L640.
- Glinsky GV. Tripartite combination of candidate pandemic mitigation agents: vitamin D, Quercetin, and Estradiol Manifest properties of medicinal agents for targeted mitigation of the COVID-19 pandemic defined by genomics-guided tracing of SARS-CoV-2 targets in human. Biomedicines. 2020. DOI:https://doi.org/10.3390/BIOMEDICINES8050129
- Arias-Reyes C, Zubieta-DeUrioste N, Poma-Machicao L, et al. Does the pathogenesis of SARS-CoV-2 virus decrease at high-altitude? Respir Physiol Neurobiol. 2020;277:103443.
- Shao W, Li X, Goraya MU, et al. Evolution of influenza a virus by mutation and re-assortment. Int J Mol Sci. 2017;18(8):1650.
- Ren L, Zhang W, Han P, et al. Influenza A virus (H1N1) triggers a hypoxic response by stabilizing hypoxia-inducible factor-1α via inhibition of proteasome. Virology. 2019;530:51–58.
- Guo X, Zhu Z, Zhang W, et al. Nuclear translocation of HIF-1α induced by influenza A (H1N1) infection is critical to the production of proinflammatory cytokines. Emerging Microbes Infect. 2017;6(1):1–8.
- Zhao C, Chen J, Cheng L, et al. Deficiency of HIF-1α enhances influenza A virus replication by promoting autophagy in alveolar type II epithelial cells. Emerging Microbes Infect. 2020;9(1):691–706.
- Bohmwald K, Espinoza JA, Rey-Jurado E, et al. Human respiratory Syncytial Virus: infection and pathology. Semin Respir Crit Care Med. 2016;37(4):522–537.
- Haeberle HA, Dürrstein C, Rosenberger P, et al. Oxygen-independent stabilization of hypoxia inducible factor (HIF)-1 during RSV infection. PLoS ONE. 2008. DOI:https://doi.org/10.1371/journal.pone.0003352
- Kilani MM, Mohammed KA, Nasreen N, et al. RSV causes HIF-1α stabilization via NO release in primary bronchial epithelial cells. Inflammation. 2004;28(5):245–251.
- Ganar K, Das M, Sinha S, et al. Newcastle disease virus: current status and our understanding. Virus Res. 2014;184:71–81.
- Etriwati RD, Setiyaningsih S, Setiyaningsih S. Pathology and immunohistochemistry study of Newcastle disease field case in chicken in Indonesia. Vet World. 2017;10(9):1066–1071.
- Abd-Aziz N, Stanbridge EJ, Shafee N. Newcastle disease virus degrades HIF-1α through proteasomal pathways independent of VHL and p53. J Gen Virol. 2016;97(12):3174–3182.
- McDonald PC, Dedhar S. Carbonic anhydrase IX (CAIX) as a mediator of hypoxia-induced stress response in cancer cells. Subcell Biochem. 2014. DOI:https://doi.org/10.1007/978-94-007-7359-2_13
- Rozo-Lopez P, Drolet BS, Londoño-Renteria B. Vesicular stomatitis virus transmission: a comparison of incriminated vectors. Insects. 2018;9(4):190.
- Hwang IIL, Watson IR, Der SD, et al. Loss of VHL confers hypoxia-inducible factor (HIF)-dependent resistance to vesicular Stomatitis Virus: role of HIF in antiviral response. J Virol. 2006;80(21):10712–10723.
- Norman KL, Hirasawa K, Yang AD, et al. Reovirus oncolysis: the Ras/RalGEF/p38 pathway dictates host cell permissiveness to reovirus infection. Proc Natl Acad Sci U S A. 2004;101(30):11099–11104.
- Hotani T, Tachibana M, Mizuguchi H, et al. Reovirus double-stranded RNA genomes and polyI:C induce down-regulation of hypoxia-inducible factor 1α. Biochem Biophys Res Commun. 2015;460(4):1041–1046.
- Hotani T, Mizuguchi H, Sakurai F. Systemically administered Reovirus-induced downregulation of hypoxia inducible factor-1α in subcutaneous tumors. Mol Ther Oncolytics. 2019;12:162–172. .
- Karayiannis P. Hepatitis B virus: virology, molecular biology, life cycle and intrahepatic spread. Hepatol Int. 2017. DOI:https://doi.org/10.1007/s12072-017-9829-7
- Moon EJ, Jeong CH, Jeong JW, et al. Hepatitis B virus X protein induces angiogenesis by stabilizing hypoxia-inducible factor-1alpha. FASEB J : Off Publ Fed Am Soc Exp Biol. 2004;18(2):1–16.
- Slagle BL, Bouchard MJ. Hepatitis B virus X and regulation of viral gene expression. Cold Spring Harb Perspect Med. 2016;6(3):a021402.
- Han HK, Han CY, Cheon EP, et al. Role of hypoxia-inducible factor-α in hepatitis-B-virus X protein-mediated MDR1 activation. Biochem Biophys Res Commun. 2007;357(2):567–573.
- Levy JA. Pathogenesis of human immunodeficiency virus infection. Microbiol Rev. 1993;57(1):183–289.
- Deshmane SL, Amini S, Sen S, et al. Regulation of the HIV-1 promoter by HIF-1 and Vpr proteins. Virol J. 2011;8(1):477.
- Deshmane SL, Mukerjee R, Fan S, et al. Activation of the oxidative stress pathway by HIV-1 Vpr leads to induction of hypoxia-inducible factor 1α expression. J Biol Chem. 2009;284(17):11364–11373.
- Duette G, Pereyra Gerber P, Rubione J, et al. Induction of HIF-1α by HIV-1 infection in CD4 + T cells promotes viral replication and drives extracellular vesicle-mediated inflammation. MBio. 2018;9(5). DOI:https://doi.org/10.1128/mBio.00757-18
- Fallah J, Rini BI. HIF inhibitors: status of current clinical development. Curr Oncol Rep. 2019;21(1). DOI:https://doi.org/10.1007/s11912-019-0752-z
- Lee JW, Ko J, Ju C, et al. Hypoxia signaling in human diseases and therapeutic targets. Exp Mol Med. 2019. DOI:https://doi.org/10.1038/s12276-019-0235-1
- Eltzschig HK, Bratton DL, Colgan SP. Targeting hypoxia signalling for the treatment of ischaemic and inflammatory diseases. Nat Rev Drug Discov. 2014. DOI:https://doi.org/10.1038/nrd4422
- Semenza GL. Hypoxia-inducible factor 1 and cardiovascular disease. Annu Rev Physiol. 2014;76(1):39–56.
- Sano M, Minamino T, Toko H, et al. p53-induced inhibition of Hif-1 causes cardiac dysfunction during pressure overload. Nature. 2007;446(7134):444–448.
- Semenza GL. Regulation of metabolism by hypoxia-inducible factor 1. Cold Spring Harb Symp Quant Biol. 2011;76:347–353.
- Wei H, Bedja D, Koitabashi N, et al. Endothelial expression of hypoxia-inducible factor 1 protects the murine heart and aorta from pressure overload by suppression of TGF- signaling. Proc Natl Acad Sci U S A. 2012;109(14):E841-E850.
- Hölscher M, Schäfer K, Krull S, et al. Unfavourable consequences of chronic cardiac HIF-1α stabilization. Cardiovasc Res. 2012;94(1):77–86.
- Haase VH. HIF-prolyl hydroxylases as therapeutic targets in erythropoiesis and iron metabolism. Hemodialysis Int. 2017;21:S110-S124. .
- Rapa SF, Di Iorio BR, Campiglia P, et al. Inflammation and oxidative stress in chronic kidney disease—potential therapeutic role of minerals, vitamins and plant-derived metabolites. Int J Mol Sci. 2020. DOI:https://doi.org/10.3390/ijms21010263
- Tanaka T, Eckardt KU. HIF Activation Against CVD in CKD: novel Treatment Opportunities. Semin Nephrol. 2018;38(3):267–276.
- Jelkmann W. Regulation of erythropoietin production. J Physiol. 2011;589(6):1251–1258.
- Semenza GL. Targeting HIF-1 for cancer therapy. Nat Rev Cancer. 2003;3(10):721–732.
- Laughner E, Taghavi P, Chiles K, et al. HER2 (neu) signaling increases the rate of hypoxia-inducible factor 1α (HIF-1α) synthesis: novel mechanism for HIF-1-mediated vascular endothelial growth factor expression. Mol Cell Biol. 2001;21(12):3995–4004.
- Ravi R, Mookerjee B, Bhujwalla ZM, et al. Regulation of tumor angiogenesis by p53-induced degradation of hypoxia- inducible factor 1α. Genes Dev. 2000. DOI:https://doi.org/10.1101/gad.14.1.34
- Maxwell PH, Wlesener MS, Chang GW, et al. The tumour suppressor protein VHL targets hypoxia-inducible factors for oxygen-dependent proteolysis. Nature. 1999;117(10):1518–1528.
- Beasley NJP, Leek R, Alam M, et al. Hypoxia-inducible factors HIF-1α and HIF-2α in head and neck cancer: relationship to tumor biology and treatment outcome in surgically resected patients. Cancer Res. 2002;62(9):2493–2497.
- Volm M, Koomägi R. Hypoxia-inducible factor (HIF-1) and its relationship to apoptosis and proliferation in lung cancer. Anticancer Res. 2000;20(3A):1527–1533.
- Koukourakis MI, Giatromanolaki A, Sivridis E, et al. Hypoxia-inducible factor (HIF1A and HIF2A), angiogenesis, and chemoradiotherapy outcome of squamous cell head-and-neck cancer. Int J Radiat Oncol Biol Phys. 2002;53(5):1192–1202.
- Giatromanolaki A, Koukourakis MI, Sivridis E, et al. Relation of hypoxia inducible factor 1α and 2α in operable non-small cell lung cancer to angiogenic/molecular profile of tumours and survival. Br J Cancer. 2001;85(6):881–890.
- Birner P, Schindl M, Obermair A, et al. Overexpression of hypoxia-inducible factor 1α is a marker for an unfavorable prognosis in early-stage invasive cervical cancer. Cancer Res. 2000a;60(17):4693–4696.
- Schindl M, Schoppmann SF, Samonigg H, et al. Overexpression of hypoxia-inducible factor 1α is associated with an unfavorable prognosis in lymph node-positive breast cancer. Clin Cancer Res. 2002;8(6):1831–1837.
- Sivridis E, Giatromanolaki A, Gatter KC, et al. Association of hypoxia-inducible factors 1α and 2α with activated angiogenic pathways and prognosis in patients with endometrial carcinoma. Cancer. 2002;95(5):1055–1063.
- Aebersold DM, Burri P, Beer KT, et al. Expression of hypoxia-inducible factor-1α: A novel predictive and prognostic parameter in the radiotherapy of oropharyngeal cancer. Cancer Res. 2001;61(7):2911–2916.
- Birner P, Schindl M, Obermair A, et al. Expression of hypoxia-inducible factor 1α in epithelial ovarian tumors: its impact on prognosis and on response to chemotherapy. Clin Cancer Res. 2001;7(6):1661–1668.
- Choudhry H, Albukhari A, Morotti M, et al. Tumor hypoxia induces nuclear paraspeckle formation through HIF-2α dependent transcriptional activation of NEAT1 leading to cancer cell survival. Oncogene. 2015. DOI:https://doi.org/10.1038/onc.2014.378
- Kaidi A, Qualtrough D, Williams AC, et al. Direct transcriptional up-regulation of cyclooxygenase-2 by hypoxia-inducible factor (HIF)-1 promotes colorectal tumor cell survival and enhances HIF-1 transcriptional activity during hypoxia. Cancer Res. 2006;66(13):6683–6691.
- Yoo Y-G, Christensen J, Huang LE. HIF-1α confers aggressive malignant traits on human tumor cells independent of its canonical transcriptional function. Cancer Res. 2011;71(4):1244–1252.
- Corzo CA, Condamine T, Lu L, et al. HIF-1α regulates function and differentiation of myeloid-derived suppressor cells in the tumor microenvironment. J Exp Med. 2010;207(11):2439–2453.
- Zhao T, Ren H, Jia L, et al. Inhibition of HIF-1α by PX-478 enhances the anti-tumor effect of gemcitabine by inducing immunogenic cell death in pancreatic ductal adenocarcinoma. Oncotarget. 2015. DOI:https://doi.org/10.18632/oncotarget.2948
- Pore N, Gupta AK, Cerniglia GJ, et al. Nelfinavir down-regulates hypoxia-inducible factor 1α and VEGF expression and increases tumor oxygenation: implications for radiotherapy. Cancer Res. 2006;66(18):9252–9259.
- Cramer T, Yamanishi Y, Clausen BE, et al. HIF-1α is essential for myeloid cell-mediated inflammation. Cell. 2003;112(5):645–657.
- Giaccia A, Siim BG, Johnson RS. HIF-1 as a target for drug development. Nat Rev Drug Discov. 2003;2(10):803–811.
- Hu D, Linders A, Yamak A, et al. Metabolic maturation of human pluripotent stem cellderived cardiomyocytes by inhibition of HIF1α and LDHA. Circ Res. 2018. DOI:https://doi.org/10.1161/CIRCRESAHA.118.313249
- Huang H, Fan Y, Gao Z, et al. HIF-1α contributes to Ang II-induced inflammatory cytokine production in podocytes. BMC Pharmacol Toxicol. 2019;20(1). DOI:https://doi.org/10.1186/s40360-019-0340-8
- Yu T, Tang B, Sun X. Development of inhibitors targeting hypoxia-inducible factor 1 and 2 for cancer therapy. Yonsei Med J. 2017;58(3):489.
- Okumura CYM, Hollands A, Tran DN, et al. A new pharmacological agent (AKB-4924) stabilizes hypoxia inducible factor-1 (HIF-1) and increases skin innate defenses against bacterial infection. J Mol Med. 2012;90(9):1079–1089.