ABSTRACT
We exploited bacterial infection assays using the fruit fly Drosophila melanogaster to identify anti-infective compounds that abrogate the pathological consequences in the infected hosts. Here, we demonstrated that a pyridine-3-N-sulfonylpiperidine derivative (4a) protects Drosophila from the acute infections caused by bacterial pathogens including Pseudomonas aeruginosa. 4a did not inhibit the growth of P. aeruginosa in vitro, but inhibited the production of secreted toxins such as pyocyanin and hydrogen cyanide, while enhancing the production of pyoverdine and pyochelin, indicative of iron deprivation. Based on its catechol moiety, 4a displayed iron-chelating activity in vitro toward both iron (II) and iron (III), more efficiently than the approved iron-chelating drugs such as deferoxamine and deferiprone, concomitant with more potent antibacterial efficacy in Drosophila infections and unique transcriptome profile. Taken together, these results delineate a Drosophila–based strategy to screen for antipathogenic compounds, which interfere with iron uptake crucial for bacterial virulence and survival in host tissues.
GRAPHICAL ABSTRACT
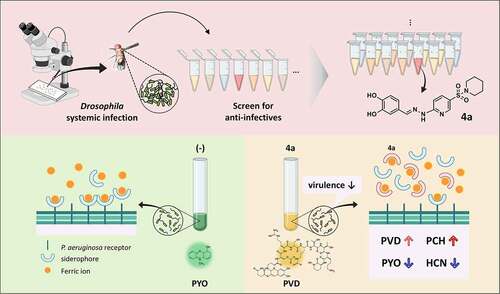
Disclosure statement
No potential conflict of interest was reported by the authors.
Data availability statement
The data that support the findings of this study are available from the corresponding author upon reasonable request.
Supplementary material
Supplemental data for this article can be accessed here